DOI:
10.1039/D1MA00944C
(Review Article)
Mater. Adv., 2022,
3, 756-778
Design of two-dimensional halide perovskite composites for optoelectronic applications and beyond
Received
11th October 2021
, Accepted 19th November 2021
First published on 24th November 2021
Abstract
Two-dimensional (2D) halide perovskites have attracted increasing attention because of their unique optical and electronic properties, resulting in versatile applications in the fields of energy, information, display and lighting, etc. However, due to some unfavorable characteristics of 2D halide perovskites, such as their low carrier mobility and low radiation recombination efficiency, researchers have combined them with other functional materials to form composites with enhanced features not observed in their individual components, manifesting the positive “1 + 1 > 2” effect. In this review, we summarize the latest progress and future perspectives of 2D halide perovskite composites, focusing on their structures, fundamental optoelectronic properties, characterization techniques and typical use. 2D halide perovskite composites have become the most recently discovered goldmine to be exploited for endless possibilities beyond optoelectronic applications and require joint endeavors of researchers from interdisciplinary fields.

Tao Song
| Tao Song received his Master's Degree in Chemical Engineering from Northwest Normal University. He is currently pursuing his PhD Degree in Physical Chemistry in Lanzhou University under the supervision of Prof. Qiang Wang. His interest focuses on the optoelectronic properties of perovskite materials. |

Qing-Xiu Ma
| Qing-Xiu Ma is currently pursuing her Master's Degree in Physical Chemistry in Lanzhou University under the supervision of Prof. Qiang Wang. Her research interest focuses on conductive pastes for flexible electronic devices. |

Qiang Wang
| Qiang Wang received his BS Degree from Wuhan University in 1999. He then joined Prof. Yi Chen's group at the Institute of Chemistry, Chinese Academy of Sciences as a Graduate Student and performed research in the field of capillary electrophoresis (CE) and spectroscopic analysis. He obtained his MS Degree in 2002. Subsequently, he pursued his PhD Degree in Prof. Rosina Georgiadis’ group at Boston University in the USA, developing novel label-free detection methods based on surface plasmon resonance (SPR). He transferred to Boston College in 2004 and worked on ultrafast time-resolved spectroscopy under the guidance of Prof. Torsten Fiebig. His main topics were focused on DNA photonics study on the femtosecond time scale and photoinduced electron and energy transfer processes in DNA upon photon excitation. He obtained his PhD Degree in May 2008 and became an Associate Professor of Chemistry at Lanzhou University in October. He was promoted as a Full Professor in 2017. His current research interests include nonlinear optics and ultrafast spectroscopy. |

Hao-Li Zhang
| Hao-Li Zhang received his BS in Organic Chemistry in 1994, and PhD Degree in 1999 from Lanzhou University. He then worked in the University of Leeds and Oxford University as a postdoc. In 2004, he was appointed as a Full Professor by the State Key Laboratory of Applied Organic Chemistry (SKLAOC) of Lanzhou University. He is currently the Deputy Director of SKLAOC and Deputy Dean of the College of Chemistry and Chemical Engineering. In 2014, he became a Fellow of The Royal Society of Chemistry (FRSC). He is an Editorial Board Member of Acta Physico-Chimica Sinica and Chinese Chemical Letters, and an Advisory Board Member of Chem. Soc. Rev. Prof. Hao-Li Zhang is interested in developing new organic functional materials for electronic and optoelectronic applications. He has published more than 240 research papers in peer-reviewed international journals with over 5000 citations, and his H index is 37. His current research projects include the design and synthesis of organic semiconductors; novel light-emitting materials; nonlinear optical materials; molecular electronics; and novel organic/inorganic hybrid materials for advanced optoelectronics. |
1. Introduction
Halide perovskites belong to the enormous perovskite family, which have the form ABX3, where A is a monovalent cation such as methylammonium and alkali cation; B is a bivalent cation such as Pb2+; and X is a monovalent anion such as a halide anion. The metal halide [BX6]4− octahedral building blocks show great structural tunability in various formats including three-dimensional (3D), two-dimensional (2D), and one-dimensional (1D) or even zero-dimensional (0D) crystal structures.1–3 In general, 2D perovskites can be divided into two types, where the first type includes traditional 2D geometric nanostructure morphologies such as like nanoplates and nanosheets, which are obtained by limiting the growth of their different crystal faces with an eventual thickness on the atomic scale.4,5 The second type is electronically “2D” even in the bulk, with a crystallographic-stacking layered crystal structure. Note that both 2D perovskites and 3D perovskites with a 2D morphology are discussed in this review.
Generally, if cut along the (100), (110) and (111) crystallographic planes of the parent 3D perovskite structure, 2D halide perovskites are obtained, which results in three different orientations. Among them, the (100) oriented perovskites are most intensively studied6 and can be further divided into three types of alternating cations in the interlayer (ACI),6,7 Dion–Jacobson (DJ) phase,8 and Ruddlesden–Popper (RP) phase9,10 according to the capping cation type and relative position between the inorganic layers. Among them, the RP phase with the general chemical formula of L2An−1BnX3n+1 (here L is a large cation and A is a small cation) is the most popular, where n represents the number of metal halide octahedral layers between the two L cation layers.11,12 An infinite n number indicates a 3D perovskite and a finite n number suggests an ideal quantum well (QW). Thus, by adjusting the size of n, tailored band gaps and quantum confinement effects can be achieved.13
Moreover, with a hydrophobic organic spacer, 2D halide perovskites generally exhibit greater moisture resistance than their 3D counterparts. Due to their high photostability, chemical stability and tunable optoelectronic properties, 2D halide perovskite materials have found broad applications in fields such as solar cells and light-emitting diodes (LEDs) in recent years.14,15 Usually in these devices, crystals growing along the vertical direction of the substrate in the film contain numerous phases instead of a single phase. The 3D phase generally has a smaller exciton binding energy and is more appropriate for application in solar cells. The 2D phase with a relatively high exciton binding energy is more appropriate for application in luminescent LEDs. Meanwhile, electrons and holes can transfer between different phases based on the energy level, with electron transport occurring from the 2D phase to 3D phase, whereas the reverse occurs for holes. Hence, depending on the type of device, the direction of crystal growth is crucial. Specifically, a deposition of the 3D phase on the bottom is beneficial for forward devices given that it is more conductive to charge collection and transfer, whereas deposition of the 2D phase on the bottom is more favorable for reverse devices.16
Compared with single semiconductor devices, those based on heterostructures can effectively manipulate the generation, reorganization and transmission of carriers. Due to the unfavorable characteristics of 2D halide perovskites, such as low carrier mobility and low radiation recombination efficiency,17 researchers have combined them with other optoelectronic materials to solve these shortcomings. In comparison with pristine perovskites, heterostructures consist of two or more materials with different energy levels and demonstrate enhanced performances not exhibited by a single material system. Due to the weak van der Waals coupling between the organic layer and the inorganic layer, the 2D perovskite sheets can be mechanically stripped from their bulk crystals. In particular, the possibility of the delamination of 2D perovskites allow them to form different van der Waals heterostructures with 2D materials such as graphene, transition metal disulfides (TMDS), black phosphorus (BP) and other types of 2D perovskites.26–28 These 2D/2D heterostructures have a sharp atomic interface without surface dangling bonds, which are especially important for high-performance and low-noise optoelectronic devices.29 2D perovskites can also be combined with 3D perovskites, organic polymers, carbon nanotubes (CNTs) and others to create optoelectronic devices with novel properties. Therefore, composite structures based on 2D halide perovskites will provide an excellent platform for the further exploration of new applications.
Our group have made contributions in this field by developing methods for the fabrication of novel perovskites and/or composites and studying their photoexcited state dynamics through various time-resolved spectroscopic techniques. In this review, we summarize the latest progress on composite structures based on 2D halide perovskites (Fig. 1), focusing on their structure, fundamental optoelectronic properties, characterization techniques and applications. Initially, we introduce the types and fabrication of 2D perovskite composites in Section 2. Subsequently, in Sections 3 and 4, the fundamental optoelectronic properties and common characterization techniques of 2D perovskite composites are discussed, respectively. We discuss these composites in detail based on their latest optoelectronic applications and beyond in Section 5. Finally, the prospects and challenges of this field are summarized in Section 6. This review is expected to provide new insights for the future direction of 2D perovskite composites.
 |
| Fig. 1 Schematic illustration of 2D halide perovskite composite structures with versatile applications. Adapted with permission from ref. 18–25. | |
2. Fabrication of 2D perovskite composites
2.1 General fabrication methods
The liquid phase method is the most commonly used method to prepare metal halide perovskites.30,31 The growth process can be controlled by adjusting the surfactants in solution to obtain perovskite nanosheets directly, which are usually uniformly distributed and convenient for further processing. Many research groups have reported the synthesis of MAPbBr3 and CsPbBr3 nanosheets with a thickness of several nanometers in solution. Zeng et al.32 prepared CsPbBr3 nanosheets with a thickness of about 3.3 nm via a solution method for the first time using long-chain alkyl amine and oleic acid soft surfactant templates (Fig. 2a). Similarly, Manna et al.33 obtained CsPbBr3 nanosheets by introducing shorter alkylamines and longer oleic acid amines as ligands. The transverse size was adjusted by changing the ratio of shorter ligands to longer ligands, while the thickness was maintained at 3 nm (Fig. 2b). Liu et al.34 synthesized CsPbX3 nanosheets at a high temperature (170 °C) using long-chain carboxylic acids and short-chain amines as ligands. Dou et al.35 directly grew large-area and atomic thin 2D halide perovskite sheets in solution (Fig. 2c), where ternary solvent mixtures were used and crystals grew during solvent evaporation. The atomic force microscopy (AFM) images confirmed that the thinnest sheet was a single-layer structure with a thickness of about 1.6 nm. This group36 further used the liquid phase epitaxy growth method to inhibit the in-plane ion diffusion in 2D halide perovskites by rigid π-conjugated organic ligands, such as dithiophenylethylammonium (2T) and phenylethylammonium (PEA), to create a transverse heterostructure close to the atomic interface.
 |
| Fig. 2 (a) 2D CsPbBr3 nanosheets. Low-magnification and high-magnification images. Adapted with permission from ref. 32. (b) TEM images of the CsPbBr3 nanosheets (scale bar is 1 μm). Adapted with permission from ref. 33. (c) Structural illustration of a single layer of (C4H9NH3)2PbBr4 and optical images of the 2D square sheets (scale bar is 10 μm). Adapted with permission from ref. 35. (d) Crystal structure of (PEA)2PbBr4 and TEM image of the 2D (PEA)2PbBr4 nanosheets. Adapted with permission from ref. 37. (e). Morphology of perovskite thin films (scale bar is 10 μm). Adapted with permission from ref. 40. (f) Optical microscopic images of 2D PbI2 platelets (scale bar is 10 nm). Adapted with permission from ref. 41. | |
The substitution of organic ammonium ions for Cs+ ions has been proven to be an effective method to transform perovskites into 2D RP layered structures and introduce quantum confinement effects. For example, Jie et al.37 reported the colloidal synthesis of (PEA)2PbBr4via the substitution of Cs+ ion with PEA+ (Fig. 2d). The large organic cations prevented water from invading PbX4− and improved the chemical stability. Zhao et al.38 fabricated a 2D/3D perovskite composite by adding Cs+ to 2D (PEA)2PbBr4 nanoplates, which combined the excellent stability of 2D perovskites and low exciton binding energy of 3D perovskites, showing promise for practical LEDs. Wright et al.39 reported the preparation of a heterostructure of both horizontally and vertically aligned perovskite (PEA)2PbBr4 grown on 3D CsPbBr3 in solution, which provided an ideal platform to study the physical properties and cross-heterojunction charge transfer process in more detail.42Table 1 summarizes the experimental conditions for the synthesis of 2D perovskites via liquid phase methods.
Table 1 2D perovskites synthesized via liquid-phase methods
Component |
Temperature |
Ligands |
Atmosphere |
Thickness |
Preparation methods |
Emission peak |
Ref. |
2D CsPbBr3 |
140 °C |
Oleyl amine (OAm), dodecylamine, and n-octylamine and oleic acid (OA) |
N2 |
3.3 nm |
Hot-injection |
— |
32
|
2D CsPbX3 (Cl−, Br− and I−) |
145–155 °C |
Octanoic acid, octylamine, OA and OAm |
N2 |
3 nm |
Hot-injection |
452 nm |
33
|
2D CsPbBr3 |
140 °C |
Hexanoic acid and OAm |
N2 |
2.6 ± 0.4 nm |
Hot-injection |
— |
34
|
2D (C4H9NH3)2PbBr4 |
75 °C |
— |
Ar2 |
∼1.6 nm (±0.2 nm) |
Solvent evaporation |
406 nm |
35
|
(2T)2PbI4–(2T)2PbBr4 |
50 °C |
Dithiophenylethylammonium (2T) |
N2 |
5 nm |
Solvent evaporation |
520 nm |
36
|
(PEA)2PbBr4 |
Room temperature |
n-Octylamine |
Air |
4 nm |
Anti-solvent recrystallization |
410 nm |
37
|
2D (BA)2(MA)n−1PbnBr3n+1 |
140 °C |
— |
Air |
50–90 μm |
Precursor acid precipitation |
420–690 nm |
48
|
2D (PEA)2PbBr4 |
Room temperature |
Octylamine |
Air |
— |
Anti-solvent recrystallization |
415 nm |
38
|
However, using the colloidal method, it is difficult to obtain larger sheets and mostly mixtures with different thicknesses are produced. In comparison, non-colloidal solution synthesis simply involves blending stoichiometric amounts of precursors to control the sheet thickness. Gas-phase growth, one of the commonly used methods for the synthesis of 2D materials, including chemical vapor deposition (CVD) and van der Waals epitaxy, is another popular method for the preparation of smooth large-area perovskite nanosheets. Xiong et al.43 first used the van der Waals epitaxy method to grow lead halide nanosheets on mica, and then through the gas–solid heterogeneous reaction with methyl ammonium halide molecules, 2D perovskite nanosheets with a transverse size of 5–30 μm and thickness from several atoms to hundreds of nanometers were obtained. Subsequently, Shi et al.40 reported the 2D van der Waals growth of 3D materials (non-layered semiconductors of MAPbCl3) on ultra-thin mica (Fig. 2e). Although high-quality perovskites were obtained by this method, a substrate with a high melting point (>350 °C) was required during high-temperature growth, which is not suitable for flexible substrates with low melting points. Therefore, alternative methods need to be developed to produce 2D perovskites on different substrates, especially on flexible ones. To solve this problem, Li et al.41 reported the controlled synthesis of 2D perovskite (Fig. 2f) on arbitrary substrates, including SiO2/Si, Si, mica, glass and flexible polydimethylsiloxane (PDMS) substrates (as long as the melting point of the substrate was higher than 100 °C). The results showed that the gas-phase synthesis provided additional flexibility for the fabrication of a new 2D halogen perovskite composite.
2.2 Inorganic material/2D perovskite composites
Composite materials have played an indispensable role in the long history of materials development and use. After two or more components are combined in a specific order, the complex often exhibits the unique advantages of each component, thus improving the overall properties of the material. 2D perovskites have shown great potential as new materials for next-generation optoelectronic technology. Moreover, they can be synthesized at a low cost, providing more possibilities for their industrialization. However, the application of 2D perovskite is still limited by some inherent drawbacks such as low carrier mobility and low radiation recombination efficiency.44 Accordingly, many researchers have combined 2D perovskites with inorganic materials to overcome these issues. In this section, we discuss the composite structure of 2D perovskite and inorganic materials based on the early and ongoing research progress.
The past few years have witnessed the rapid development of 2D van der Waals materials with unique physical and chemical properties. As one of the earliest 2D materials, graphene has shown extraordinary electronic, thermal and mechanical properties.45 It is usually used as a carrier transport layer in heterostructures to obtain a greater gain. The heterostructure formed by the combination of 2D perovskite with graphene and its analogues has great potential in optoelectronic research. For example, Peng et al.46 constructed photodetectors based on 2D perovskite (C4H9NH3)2PbBr4 and monolayer graphene heterostructures (Fig. 3a) to enhance the performance of the devices. The covered monolayer graphene can protect the 2D perovskite crystals from dissolving in water or acetone, further increasing the device stability. TMDs are also used to construct heterostructures with 2D perovskites, usually forming II-type heterostructures. Duan et al.47 reported a combination of perovskite and 2D materials through a layer-by-layer van der Waals assembly process to achieve a high photoconductivity gain of up to 2200 and high optical responsiveness of 950 A W−1 in a vertical heterojunction device (Fig. 3b). Through the effective protection of perovskite by boron nitride (BN), the lifetime of the perovskite 2D heterostructure was extended to 7 months. Li et al.29 constructed photodetectors based on few-layer MoS2 (n-type) and 2D perovskite (PEA)2SnI4 (p-type) heterostructures, which exhibited an amazing responsivity of 1100 A W−1 and high rectifier ratio of 500 under a bias of 3 V. Also, the heterojunction device could sense light in the whole range of visible and near-infrared wavelengths with an adjustable light response peak.
 |
| Fig. 3 (a) Schematic illustration of graphene-contacted 2D perovskite photodetector and current–voltage (ISD–VSD) curves. Adapted with permission from ref. 46. (b) Schematic diagram of the device structure. Adapted with permission from ref. 47. (c) Optical images of PbI2 nanosheets, optical images and fluorescence photographs of MAPbI3 nanosheets (scale bar is 5 μm). Adapted with permission from ref. 49. | |
Andrew et al.50 constructed a type II heterostructure of WS2 monolayer/2D (C6H5C2H4NH3)2PbI4 (PEPI) perovskite, which had a photoresponsivity 5-times higher than that of the WS2 monolayer. Huang et al.49 reported a reversible cation exchange of hybrid perovskite and effective surface functionalization of low-dimensional materials, showing the phase and heterojunction engineering of ultra-thin perovskites. Using PbI2 as a precursor and template, perovskite nanosheets with different thicknesses and hexagonal shapes could be obtained on different substrates such as PbI2, MAPbI3 and FAPbI3 (Fig. 3c). The transverse heterojunction of perovskite and PbI2 was derived from a patterned single perovskite nanosheet using a mask made of a 2D material such as graphene, hexagonal boron nitride (h-BN) or MoS2 to cover the template prior to the conversion of PbI2 to perovskite. Perovskite-based vertical heterostructures were constructed through monolayer MoS2 and MAPbI3 stacking. The flexible design of perovskites and their integration with a variety of 2D materials offer numerous possibilities for new features and functions.
2D perovskites can also be compounded with other inorganic materials. Ren et al.51 made a field-effect transistor (FET) using CNTs and the 2D perovskite (PEA)2PbI4 and achieved light-enhanced ion migration by changing the doping amount of CNTs. Meanwhile, the FET could achieve multi-stage optical memory by changing the excitation intensity. Ion doping was also used to regulate the optical, electrical and magnetic properties of 2D perovskites. Fu et al.52 reported Mn2+-alloyed 2D perovskite QW of Mn:(PEA)2PbBr4 (PEA, phenylethyl-ammonium) with a high energy transfer efficiency (ΦET = 56%) and solid-state photoluminescence quantum yield (PLQY = 54%). Considering the huge tunability of 2D perovskites and large number of available inorganic materials, we expect that there is a lot of room for the exploration of composite structures with novel physical properties and expanded functions.
2.3 Organic material/2D perovskite composites
The selection of 2D perovskite composite materials is not only limited to inorganic materials. Organic materials have also been widely used to couple with 2D perovskites.53 Compared with inorganic materials, organic materials can make use of their own hydrophobic interaction to reduce the contact between perovskite nanocrystals and the external environment, and thus increase the stability of the perovskite. Perovskites can also be modified by functional organic molecules (such as strong electron donor or acceptor) to contribute directly to the electron band, and thus promote charge separation.54 Chemical modification in the form of chemical bonding is unique for organic material/2D perovskite composites and provides endless functionalization possibility for 2D perovskites.
Thus far, a large number of optoelectronic devices based on 2D perovskites have been constructed, and the stability of perovskites is the key to their practical application. Although several methods that encapsulated perovskites in a bulk matrix (for example, alumina55 and silica56) improved the stability, the systems were usually complex macroscopic mixed materials, rather than nanostructures with clearly defined colloid dispersibility and processability. Through a dual protection layer, i.e., an inorganic shell ligated with hydrophobic polymers, Lin et al.57 developed an amphiphilic diblock copolymer method to prepare highly stable anisotropic CsPbBr3 nanosheets (Fig. 4a). Anchoring other multi-dentate amphiphilic polymer ligands on the surface and templated growth of shell materials can create a rich variety of multi-functional and stable perovskite nanosheets.
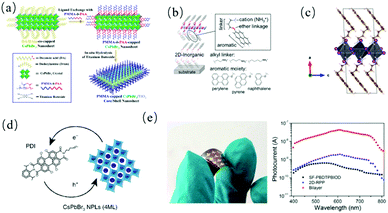 |
| Fig. 4 (a) Schematic illustration of the fabrication of the CsPbBr3/TiO2 core/shell structure. Adapted with permission from ref. 57. (b) Layered perovskite and ammonium cation-linking method. Adapted with permission from ref. 58. (c) Unit cell of the (PyrC4)2PbI4 2D layered hybrid perovskite. Adapted with permission from ref. 60. (d) Schematic representation of the charge transfer between CsPbBr3 NPLs and PDI molecules. Adapted with permission from ref. 54. (e) Flexible device and spectral photocurrents. Adapted with permission from ref. 62. | |
In some recent studies, functional organic cations were introduced into 2D perovskites to change their photovoltaic properties. Samuel et al.58 chose n = 1 layered perovskite with the molecular formula of (aromatic-O-linker-NH3)2PbI4; naphthalene, pyrene, or perylenes as the aromatic moiety; and ethyl, propyl, or butyl as the linker. Through this method, customized synthetic organic cations were employed to enhance the out-of-plane conductivity of 2D perovskites (Fig. 4b).
Although the photovoltaic performance of materials can be significantly improved by using conjugated organic molecules as cations in 2D layered perovskite, the available organic cations of 2D layered perovskite are still limited because of their large volume.59 Thus, to overcome this limitation, Dirk et al.60 introduced charge transfer complexes into a 2D layered perovskite via self-assembly using pyrene-butylammonium (pyrene-C4H8-NH3+; PyrC4) as the donor molecule, tetracyanoquinodimethane (TCNQ) and tetracyanobenzene (TCNB) as strong acceptor molecules (Fig. 4c). Besides inducing charge separation, the strategy also opened up many other possibilities such as the use of chiral molecules or singlet fission/up-conversion chromophores. Ferdinand et al.61 achieved charge separation by replacing non-conductive organic cations with organic charge-transfer complexes consisting of the electron-donor pyrene-alkylammonium (pyrene-Cn) and strong electron acceptor TCNQ. The microwave conductivity and femtosecond transient absorption showed that the light excitation of these charge transfer states led to long-lived moving charges in the inorganic layer, evidencing that charge separation could be induced in solid 2D perovskite by designing the organic layers. Recently, the same research group54 went one step further to replace the non-functional organic ligands (Fig. 4d) on the surface of colloidal nanoplatelets with perylene diimides (PDI) to achieve the effective formation of mobile free charge carriers, which were generated at ten times higher yield with lifetimes (tens of microseconds) two orders of magnitude longer. The composites greatly enhanced the performance of the 2D perovskites.
Organic material/2D perovskite composites also show great potential in flexible optoelectronics. Wang et al.62 designed a transverse photodetector by depositing a double-cable conjugated polymer SF-PBDTPBIOD (based on benzodithiophene (BDT) backbone polymer and perylene bisimide (PBI) side units) layer on 2D perovskite (BA)2(MA)n−1PbnI3n+1 films (Fig. 4e). Due to the enhanced charge transfer and collection, the device exhibited a high responsivity of 27.06 A W−1 and an on/off ratio of 1379. The performance of the flexible device using polyimide as the substrate was comparable to that of the device on glass.
3. Optoelectronic properties of 2D perovskite composites
Metal halide perovskites have great advantages in optical and optoelectronic properties, such as high quantum yield,63 high color purity,64 adjustable band gap,65 and high optical absorption coefficient.66 Hybrid 2D halide perovskites perform even better in some aspects. For instance, 2D perovskite thin films with low defect density and good crystallization quality have high electron and hole mobility and transport equilibrium. Compared with the traditional III–V and II–VI materials, with a decrease in their size, the Coulomb force between electrons and holes in the 2D structure increases, and the dielectric constant between the organic layer and the inorganic layer is quite different. Thus these electron–hole pairs have higher binding energy and higher photoluminescence efficiency. Specifically, the following attributes of 2D halide perovskites are particularly noteworthy.
3.1 Strong exciton binding energy
One of the most interesting optoelectronic properties of 2D materials is their large exciton binding energy due to the decrease in dielectric shielding and quantum confinement effect.67 For example, the exciton binding energy of monolayer WS2 is as high as 700 meV. A large number of studies have reported the large exciton binding energy of 2D halide perovskites.68–70 For these materials, due to the huge energy difference and high contrast of dielectric constant between their inorganic and organic layers, the Coulomb interaction between photogenerated electrons and holes is very strong, resulting in a significant oscillator strength and very large exciton binding energy (EB, usually hundreds of meV), and hence efficient radiative recombination and high PLQY.71,72 Note that the exciton binding energy of 2D perovskites can be fine-tuned via small-molecule intercalation. Smith et al.73 stabilized 2D perovskite by inserting I2 molecules into the binding spacer, which led to a more polarized organic layer than the inorganic layer and reduced the dielectric limitation of excitons. Their electronic, optical and magnetic properties can be adjusted accordingly.13
3.2 Tunable band gap
The band gap is one of the most important parameters of semiconductors. Narrow band gap perovskite semiconductors are widely used in devices related to photon absorption or emission. The optical properties of 2D perovskites depend on their crystal composition, the thickness of the perovskite layer and the dielectric contrast between perovskites and organic spacer. For example, in 2D perovskite nanosheets with the molecular formula of ABX3, the valence band depends on the p-orbital of halogen X and the s-orbital of the B ion, the conduction band depends on the p-orbital of the B ion, and the ion at the A site does not have any effect on the electron transition. Adjusting the ratio of the B-site ion to halogen atom can tailor the band gap of 2D perovskites. The band gap also depends on the composition of the perovskite layers. With a decrease in the number of perovskite layers, the band gap of perovskite increases gradually.74,75
3.3 Ion migration
For perovskite-based optoelectronics, ion migration accounts for many unique behaviors such as current–voltage hysteresis, low-frequency giant dielectric response, and switchable photovoltaic effect.76 Among them, the reversible structural swell–shrink effect can be employed to fabricate tunable photonic/optoelectronic devices such as ultrafast solid-state displays, semi-transparent smart glasses and artificial retina devices.76,77 The ion migration in perovskite materials is also applied to memristors.78 Huang et al.79 fabricated a 2D organometal trihalide perovskite-based memristor. By adding a bias voltage, the p–i–n structure was converted to an n–i–p structure, realizing a gradual change in the device resistance. Compared with 3D perovskites, 2D perovskites are more popular in memristors because of their enhanced stability. Ren et al.80 constructed a single-crystal perovskite memristor based on a 2D RP phase, which showed outstanding resistive memory with a program current one order of magnitude lower than that of conventional materials, i.e., as low as 10 pA.
However, ion migration may cause perovskite aging and destroy the device stability. For instance, ion diffusion often corrodes the charge transfer layer or metal electrode81,82 of the device to accelerate the degradation of perovskite-based optoelectronics. Recent studies showed that long-chain organic ligands in 2D layered perovskite formed a barrier to prevent ions from migrating out of the plane and greatly improved the stability of the devices.83 Huang et al.84 synthesized single-crystal RP-type BA2MA2Pb3I10 (n = 3) to suppress the formation of iodide vacancies (VI) and methylammonium vacancy (VMA), and therefore ion migration along the electric channel in 2D perovskites.
3.4 Rashba splitting due to strong spin–orbit coupling (SOC)
The Rashba effect of perovskites stems from the strong spin–orbit coupling (SOC) with structural inversion asymmetry, which essentially affects the carrier dynamics, and thus the luminescence and photovoltaic performance of materials.13,85 Vardeny et al.86 measured the giant Rashba splitting in 2D (PEA)2PbI4 using the picosecond transient photo modulation technique. The perovskite showed a Rashba parameter of (1.6 ± 0.1) eV Å and a large Rashba energy splitting of (40 ± 5) meV. Mohammed et al.87 further used density functional theory (DFT) and time-resolved spectroscopy to study the Rashba effect of a series of organic–inorganic hybrid perovskites. They found that inherent Rashba splitting occurred in the perovskite crystals with an even number of inorganic layers (n = 2), proving that stable perovskites with a large Rashba effect could be designed by artificially controlling the number of layers. Recently, they reported 2D (3AMP)PbI4 and (4AMP)PbI4 (3AMP
=
3-(aminomethyl)piperidinium; 4AMP
=
4-(aminomethyl)piperidinium) in a DJ phase, the hot-carrier cooling kinetics of which could be controlled by Rashba band splitting.88 These studies imply that 2D perovskites may result in new breakthroughs in the development of semiconductor spintronics. However, the influence of the Rashba effect on the lattice distortion and dynamics in 2D perovskites remains largely elusive.
4. Characterization techniques for 2D perovskite composites
Besides the conventional spectroscopic techniques including steady-state absorption and fluorescence spectroscopy, laser confocal scanning microscopy for the investigation of optical properties, transmission electron microscopy (TEM) and scanning electron microscopy (SEM) for the investigation of morphology, X-ray photoelectron spectroscopy (XPS) and X-rays diffraction (XRD) for the investigation of the structures of perovskites, one noteworthy trend is the application of many advanced characterization techniques, such as highly time and spatially resolved and in situ methods.
4.1 Highly time-resolved techniques of ultrafast spectroscopy
Femtosecond transient absorption spectroscopy has become one of the most powerful approaches to disentangle the complicated excited-state photo physics and photochemistry of optoelectronic materials. In our previous review,22,89,90 we summarized the study of the excited-state dynamics of 2D materials by ultrafast spectroscopy. Many of the applications of perovskites are also closely related to their photoinduced dynamics, which is crucial for understanding the origin of the excellent optoelectronic features of materials.
In recent years, the in depth study on the ultrafast process of 2D perovskite materials has also been widely reported. Beard et al.95 reported the phonon bottleneck effect in perovskites and the time scale of biexciton recombination by using transient spectroscopy. Sum et al.96 reported the dynamic process of extracting hot electrons from perovskite materials. Feldmann et al.91 used femtosecond differential transmission spectroscopy to compare the carrier relaxation and recombination kinetics in 2D and quasi-3D halide perovskites (Fig. 5a), and the hot electron relaxation process and transient absorption in 2D perovskites. The basic understanding of carrier dynamics in perovskite composites is very important for their applications in optoelectronic devices. Knowledge of these processes is critical to design optoelectronic devices that rely on fast relaxation and charge- or energy-transfer mechanisms. Our group synthesized CsPbBr3 quantum dots (QDs) based on our previously developed mechanochemical methods.97 A type I 3D CsPbBr3/2D CsPb2Br5 heterojunction was constructed by introducing excess PbBr2 to transform CsPbBr3 into CsPb2Br5via a phase transition (Fig. 5b).92 Femtosecond transient absorption spectroscopy was employed to explore the carrier dynamics of the heterojunction, which provided the first observation of a CsPb2Br5 crystal signal in transient absorption spectra. The type I hierarchical structure enhanced the composite stability, prolonged the lifetime of photogenerated carriers, and resulted in a higher quantum yield. With the perovskite composites and additive phosphors, a white LED with a 94% color rendering index was obtained.
 |
| Fig. 5 (a) Thermalization and relaxation of photoexcited electrons and holes and transient carrier temperature cooling curves of quasi-3D and 2D perovskites. Adapted with permission from ref. 91. (b) Carrier dynamics of CsPbBr3/CsPb2Br5 heterojunction nanosheets and 3D spectra. Adapted with permission from ref. 92. (c) Evidence for an influx of electrons into CdSe from CsPbBr3 through transient absorption. Adapted with permission from ref. 93. (d) Photo-induced ultrafast electron transfer from CdSe to CsPbX3. Adapted with permission from ref. 94. | |
Richard et al.93 reported the electron transfer between 0D and 2D nanoplatelets of CsPbBr3 and CdSe. The electronic interactions among the 0D–0D, 0D–2D and 2D–2D systems were compared using static and transient absorption spectroscopy. The transient absorption measurement disclosed the ultrafast transfer of an influx of electrons into CdSe from CsPbBr3 (Fig. 5c). Amitava et al.94 reported the preparation of composites of 2D layered CdSe and CsPbX3 perovskite nanocrystals. The kinetics of ultrafast electron transfer in the composites was studied by femtosecond spectroscopy (Fig. 5d). The sample was excited at low energy (<5 μJ cm−2) at 400 nm to eliminate the multiexciton process. In the presence of CsPbX3, the in-band relaxation of pure CdSe (800 fs) decreased sharply to <100 fs. Moreover, the ultrafast electron transfer from CdSe to the perovskite increased with an increase in the content of CsPbX3 iodide composition (Fig. 5d).
4.2 High spatial resolution
The development of TEM techniques enables the internal structure of matter to be observed and characterized on the atomic and molecular scale. However, the instability of perovskites during electron beam irradiation results in a huge challenge to characterize their internal atomic/dynamic structures using conventional TEM techniques. In this context, various new techniques including cryoelectron microscopy (cryo-EM), spherical aberration correction TEM, and in situ TEM have emerged. Cryo-EM allows the observation of systems where traditional electron microscopy is powerless, such as biomolecules. Different from the traditional electron microscope using a charge coupled detector (CCD), cryo-EM uses a direct electron detector (DED), which has a better detection quantum efficiency and higher signal-to-noise ratio and can be used for imaging under lower electron radiation. Cui et al.98 reported the structure and decomposition mechanism of perovskite materials in the environment by cryo-EM for the first time, where atomic resolution imaging of MAPbI3 was illustrated (Fig. 6a). The iodide nanoparticles precipitated on the surface of MAPbI3 after short-time UV irradiation and surface roughening after exposure to air for 10 s, a phenomenon never observed by XRD techniques. The authors established the definition of a critical electron dose, where that for MAPbI3 at low temperature was 12 e− Å−2, and the spatial resolution was 1.49 Å. Similarly, the photostability of perovskite is a serious issue, but its mechanism remains controversial. It has been reported that the photoluminescence of CH3NH3PbI3 thin films increases significantly under light, which is attributed to the photo-assisted migration of I− ions.99 Recently, Sui et al.100 used quasi-in situ TEM for the first time to observe the photodegradation of MAPbI3 thin films at the grain boundary, accompanied by the formation of Pb0 particles. The growth kinetics of the Pb0 particles obeyed the exponential law of r ∝ t1/2, indicating that the degradation model was determined by the interfacial diffusion of elements. The quasi-in situ study indicated that the perovskite film degradation was accompanied not only by the production of Pb0 but also the deposition of I2. Thus, the use of advanced TEM techniques to understand the specific degradation mechanism of 2D perovskites provides useful insights for the development of more stable and effective optoelectronic devices.
 |
| Fig. 6 (a) TEM images of MAPbI3 nanowires with atomic resolution. Adapted with permission from ref. 98. (b) In situ structural evolution of MAPbI3. Adapted with permission from ref. 104. (c) In situ GI-XRD measurements of the perovskite. Adapted with permission from ref. 21. | |
Nuclear magnetic resonance spectroscopy (NMR), one indispensable tool for identifying organic molecular composition and structures, also finds applications to explore the large composition tunability and rich structural dynamics typical of 2D perovskites.101 NMR as structural analysis technology is advantageous to detect the structure and dynamics of heavy and light elements, as well as the interaction between them through heteronuclear correlation experiments.102 NMR is becoming increasingly beneficial to characterize and clarify the role of organic cations in 2D perovskites, which not only identifies the structure of the organic spacer layer and inorganic metal halide perovskite layer, but also determines the interactions between them. Especially for perovskites with a mixed organic spacer, which is difficult to characterize using diffraction techniques, NMR shows superiority. Vela et al.103 first used 207Pb solid-state NMR to monitor the degree of alloying and phase separation in mixed halide perovskites with the general formula of “CH3NH3PbX3−aX′a” (X, X′ = I, Br or Cl), revealing the existence of nonstoichiometric impurities or “dopants”. Recently the sensitivity of 207Pb has been significantly improved and the measurement time has been dramatically reduced by applying advanced NMR techniques such as magic-angle spinning (MAS), low temperatures, dynamic nuclear polarization (DNP), and proton detection.105 Lee et al.102 detected and quantified the composition and local structural characteristics of 2D RP phase BA2MAn−1Pb by combining 207Pb and 1H nuclear solid-state NMR. 1D NMR spectra were used to identify each Pb and H site. The 207Pb–1H 2D heteronuclear correlation (HETCOR) experiment disclosed the spatial proximity between them. Thus, by determining the structure and dynamics of the organic and perovskite components in the RP phase, one can better understand the local atomic environment in the material.
4.3 Time- and space-resolved multidimensional techniques
Time- and space-resolved multidimensional techniques are powerful for investigating 2D perovskites. With the combination of microscopy and ultrafast laser sources, these techniques are especially suitable for the study of dynamic processes with high time and space resolution, among which time-resolved photoluminescence (TRPL) microscopy106 and transient absorption (TA) microscopy107 are representative ones.
Transient absorption microscopy combines the ultrafast spectroscopic method and optical microscopic technique to investigate material dynamics in both space and time. Moran et al.108 imaged the electron excitation dynamics in the quantum well of the layered 2D perovskite (PEA)2(MA)n−1[PbnI3n+1] by transient absorption microscopy and compared the layered thin film with a pure phase single crystal to determine the diffusivity and two-body recombination rate. Recently, their team further combined linear spectroscopy with transient absorption microscopy to illustrate the mechanism of light-induced energy and charge transfer in layered perovskites. The specific carrier mobility trajectory38 of the layered perovskite system could be determined by the cyclic laser pulse sequence and external bias applied to photovoltaic cells. These findings shed light on the rational design of 2D perovskite-based high-performance optoelectronic devices.107
Mohite et al.106 studied the 2D layered perovskite (BA)2(MA)n−1PbnI3n+1 with n from 1 to 5 by using confocal spatial photoluminescence (PL) mapping with a resolution of ≈1 μm. They found that the lower energy edge states dominated the photophysics of 2D layered perovskites with n > 2 by dissociating excitons into longer-lived free carriers. Jin et al.109 developed a time-resolved photoluminescence imaging microscope with a submicrometer spatial resolution (≈1 μm). They studied layered (BA)2(MA)n−1PbnI3n+1 single crystals and discovered that the crystal edges with exciton dissociation ability were induced by the loss of BA ligands. Later, the same group observed long-distance carriers beyond the exciton limit in 2D perovskites.110 Using confocal fixed-point excitation of a single crystal, the luminescence of the “boundary state” of the low energy state at a certain distance from the excitation point was observed. Given that there was no recombination luminescence on the carrier transport path, they speculated that the carriers travelled in a non-luminous “dark state” in the crystal and proposed a new mechanism of long-distance carrier transport assisted by defect states.
4.4
In situ techniques
In situ technology can track the changes in the structure, composition, morphology, and optoelectronic properties of perovskites in real time. The more commonly used techniques include in situ transmission TEM,111in situ XPS,112,113 and in situ XRD.21In situ TEM is used to study the degradation process of perovskites to solve their instability problems, where the changes in their structure and morphology are observed in real time at the atomic level. However, the high-energy electron beam will cause serious damage to the target area, and hence it is necessary to carefully examine whether the perovskite decomposition is caused by electron beam-induced decomposition. Ducati et al.111 introduced in situ TEM observation of perovskites for the first time, where the effect of the manufacturing route on the thermal degradation of perovskite materials was studied. Duan et al.104 observed the thermal-induced degradation of MAPbI3in situ under controlled gas flow and showed that the crystal gradually evolved from tetragonal MAPbI3 to triangular lead iodide with a fixed crystallographic direction (Fig. 6b). Sheng et al.114 observed the degradation of perovskites by adding a bias voltage. Although in situ TEM characterization tools provide important information about the degradation mechanism of perovskites, due to the influence of electron beam irradiation, more advanced in situ scaffolds and robust samples are needed for more in-depth understanding.
Alternatively, X-ray-assisted characterization technology has been proven to be a powerful tool to study the crystal structure, chemical composition and morphology of perovskite materials. The in situ X-ray techniques commonly used in the field include XRD,115 XPS,116 and synchrotron radiation. These techniques can be employed to comprehensively evaluate the chemical, structural, phase and morphological changes in perovskites. Chen et al.21 observed in situ the formation process of perovskite films using X-ray grazed incidence diffraction (GI-XRD) based on synchrotron radiation, disclosing the transformation process of the films in different solvent vapor atmospheres (Fig. 6c). By adjusting the preparation conditions of the thin films, a highly efficient perovskite solar cell was fabricated. Different characterization methods can be used to understand perovskites from different perspectives. Advanced in situ methods are specialized in revealing how water, oxygen, heat and light act on the surface of perovskites and cause physical and chemical changes.
5. Applications
2D perovskites can be conveniently tailored to the specific application needs of traditional research areas.12 Among the many exciting properties of 2D perovskites, their excellent optoelectronic features, such as large absorption coefficient, high carrier mobility, and long diffusion length, make them suitable for applications beyond conventional ones. For example, they can be used for one-component white light emission of solid-state lighting equipment117 and have shown strong optical nonlinearity. Herein, we summarize the latest research progress on 2D perovskite composites in optoelectronic applications and beyond.
5.1 LEDs
In the past few years, 2D metal halide perovskites have become a promising candidate for high-performance LEDs. Compared with 3D perovskites, 2D layered perovskites usually have a larger exciton binding energy (hundreds of meV), which leads to the enhancement of radiation recombination and higher PLQY. The formation of cascade energy structures in 2D perovskite thin films with mixed n (layer thickness) can promote rapid and effective energy transfer from a lower n quantum well (QW) to a higher n QW (in sub-nanosecond), resulting in reduced exciton quenching and enhanced radiation recombination. Compared with 3D perovskites without hydrophobic molecules, the incorporation of hydrophobic organic ligands and the enhanced van der Waals interaction between organic molecules lead to a significant enhancement in environmental and thermal stability.118 Similar to traditional semiconductors, the luminous efficiency of optically excited or electrically excited charge carriers in perovskite materials is controlled by the relative intensity of radiative and non-radiative processes. Since the first halogen perovskite LED was reported in 2014, the device external quantum efficiency (EQE) has increased from ∼0.1%119 to ∼23%.120 Although the perovskite family has good tolerance to electronic defects, the electroluminescence efficiency achieved thus far shows that suppressing non-radiative recombination under electrical excitation is still a challenge. Non-radiative recombination is also an important mechanism of voltage loss in photovoltaic solar cells. Thus, to enhance the radiation emission process in LEDs, one of the most successful strategies is to use low-dimensional structures. Sargent et al.15 used mixed perovskites of (PEA)2MAn−1PbnI3n+1 (n = 1–5 and 10) with different quantum sizes to fabricate LEDs with an ITO/TiO2/quasi-2D layered perovskite/F8(poly(9,9′-dioctylfluorence))/MoO3/Au structure. The dynamics investigations indicated that the multi-phased perovskites channelled energy across an inhomogeneous energy landscape and concentrated carriers on smaller bandgap emitters, facilitating more effective radiation recombination.121 You et al.24 proposed a new passivation strategy for the defects at the grain boundaries. By adding ethoxylated trimethylolpropane triacrylate (ETPTA) to the antisolvent, passivation was realized both on the surface and in the bulk (Fig. 7a). By restricting the formation of defects, the authors obtained an efficient green perovskite LED with a maximum EQE of 22.49%. Di et al.122 constructed an LED emitting layer by combining quasi-2D and three-dimensional (2D/3D) perovskites and insulating polymer bulk. The photogenerated excitation migrated from quasi-2D to low-energy sites within 1 ps. The ultrafast migration ensured that any non-radiative traps with an energy higher than the final emitting species became negligible. Radiative bimolecular recombination emission in the 3D region effectively eliminated the non-radiative recombination path, resulting in an EQE of 20.1% and photoluminescence QE close to 100%. However, the high-efficiency LEDs based on perovskite are still limited to small devices, and serious performance degradation is always observed when producing large-area perovskite LEDs. Yuan et al.123 proposed a low-barrier crystallization pathway by partially anchoring amphiphilic L-norvaline molecules to a [PbBr6]4− inorganic plate to form a new mesophase with quasi-2D geometry, which overcame phase separation to obtain high-quality large-area thin films (Fig. 7b). The LEDs possessed an active area of 9.0 cm2 and EQE of 16.4%.
 |
| Fig. 7 (a) Device using ETPTA additive. Adapted with permission from ref. 24. (b) Growth of quasi-2D films without and with antisolvent. Adapted with permission from ref. 123. (c) Device architecture for a PeLED and electroluminescence spectra. Adapted with permission from ref. 124. (d) Defect passivation by crown and MPEG-MAA. Adapted with permission from ref. 125. | |
In addition to efficiency, stability and lifetime are key factors determining the eventual commercial application of perovskite optoelectronic devices. Appropriate device packaging strategies can improve their resistance to water and oxygen. Other strategies have also been reported. Ye et al.126 built a composite structure by embedding CsPbBr3 nanosheets into a lattice-matched Cs4PbBr6 crystal matrix. This design led to the improvement of surface passivation, enhancement of radiation recombination and inhibition of agglomeration, resulting in a high PLQY for the solid perovskite. Gao et al.124 used MAPbBr3 nanoplatelets as the luminescent layer and poly(9-vinylcarbazole):2-(4-biphenylyl)-5-phenyl-1,3,4-oxadiazole (PVK:PBD) as the passivation luminescent layer. The addition of the PVK:PBD polymer removed pinholes in the perovskite luminescent layer and inhibited the non-radiative current loss, showing a high luminescence of 10
590 cd m−2 at 12 V (Fig. 7c). Qi et al.125 used 18-crown-6 and poly(ethylene glycol) methyl ether acrylate (MPEG-MAA) as additives in perovskite films, which coordinated with Pb2+ and suppressed the self-aggregation of phenylbutylammonium bromide (PEABr). Consequently, the structure conversion from quasi-2D into nanocrystalline perovskite was prevented and a low defect density and high environmental stability were realized (Fig. 7d).
In short, 2D or quasi-2D layered perovskites have high brightness, wide band color tunability and excellent color purity. Combined with other desirable features such as facile solution processability and composition flexibility, these materials demonstrate potential to become mainstream emitters for LEDs.
5.2 Solar cells
In recent years, the power conversion efficiency (PCE) of 3D perovskite solar cells has exceeded 25%,127 which is comparable to that of crystalline silicon solar cells. However, the stability of perovskite solar cells seriously restricts their practical applications. Compared with 3D perovskites, their 2D counterparts offer an opportunity to overcome the stability issue because of the relatively higher chemical stability of the latter.128 However, due to the existence of long-chain complexes, the formation of multiple quantum well (MQW) structures in 2D perovskites limits the transport of photogenerated carriers, which usually reduces the photovoltaic performance.129,130 In this case, the 2D/3D hybrid structure provides a balance by inheriting the good stability of 2D perovskites and the excellent photovoltaic performance of 3D perovskites. Indeed, perovskite solar cells based on these mixed structures show higher stability and improved PCE.129 Snaith et al.131 incorporated n-butylammonium cations into FA0.83Cs0.17Pb(IyBr1−y)3 3D perovskites to form 2D perovskite sheets, which were scattered between highly oriented 3D perovskite grains and suppressed the recombination of non-radiative charge. An average PCE of 17.5% was obtained and the device remained stable in air for more than 1000 h. However, due to the existence of MQWs in 2D perovskites, the devices with 2D/3D mixed structures still need to sacrifice part of the efficiency of charge-carrier extraction. To solve this problem, Song et al.129 inserted long-chain EDBEPbI4 (EDBE = 2,2-(ethylenedioxy)bis(ethyl8bammonium)) into 3D perovskites to form a 2D/3D perovskite vertical heterostructure (Fig. 8a). The 3D perovskite grain boundaries were vertically passivated by 2D perovskites, which minimized the localization of photogenerated charge carriers in the low-dimensional perovskites. Also, the vertically arranged structure did not affect the charge carrier extraction from the 3D perovskites to the electrode, achieving a stable efficiency of 19.66%. The solar cells with an original efficiency of 90% were maintained after being placed in the air for three months (more than 3000 h). Dai et al.132 introduced two hydrophobic short-chain alkyl ammonium salts (2-chloroethylamine, CEA+ and 2-bromoethylamine, BEA+) with halogen functional groups into (Cs0.1FA0.9)Pb(I0.9Br0.1)3 3D perovskites to obtain a 2D/3D perovskite structure (Fig. 8b). The cells exhibited a PCE of up to 20.08% under one sun exposure, and the initial efficiency of 92% was maintained after aging for 2400 h at 50% ± 5% relative humidity. Noh et al.134 grew stable and highly crystalline 2D (C4H9NH3)2PbI4 on 3D (FAPbI3)0.95(MAPbBr3)0.05 thin films to produce 2D/3D heterostructures. Given that the built-in potential was augmented at the 2D/3D heterojunction, a high photovoltage was obtained in the device. The heterojunction structure produced an open-circuit voltage of 1.185 V and PCE of 24.35%. Under the hygrothermal test (85 °C/85% relative humidity), the packaged device maintained 94% of its initial efficiency after 1056 h and 98% after 1620 h of full sunlight.
 |
| Fig. 8 (a) Schematic illustration of 2D–3D PVHH structure and SEM images of perovskite films with different 2D concentrations. Adapted with permission from ref. 129. (b) Self-assembled 2D/3D perovskite. Adapted with permission from ref. 132. (c) Prepared (PDMA)MAPb2I7 thin films. (d) Temperature changes with time and current–voltage curve of the solar cell with (PDMA)MAPb2I7 as the dynamic layer. Adapted with permission from ref. 133. | |
In addition, 2D perovskites can also be used in thermochromic smart windows. Sheng et al.133 showed the robust and fast reversible thermochromic properties of 2D perovskite (PDMA)(MA)n−1PbnI3n+1 (PDMA = C6H4(CH2NH3)22+ and MA = CH3NH3+). After exposure to environmental conditions, the initial brown film became colorless upon the absorption of water, which was restored to its original color by heating at 100 °C for a few minutes or above 60 °C for several hours under ambient conditions (Fig. 8c). The reversible thermochromic property could be employed in applications such as thermochromic smart windows and thermochromic solar cells. For instance, as shown in the inset in Fig. 8d, the black box covered by perovskite windows reduced the indoor air temperature more effectively than that covered with pure glass in an enclosed space after 20 min-irradiation at 1.5 sun light intensity (∼150 mW cm−2). The photovoltaic devices with the (PDMA)(MA·H2O)Pb2I7 perovskite (n = 2) as the absorption layer displayed a PCE of more than 0.5% at high ambient temperatures.
5.3 Nanolasers
Low-dimensional perovskites have also shown huge potential in the lasing field, including edge-emitting amplified spontaneous emission (ASE), vertical-cavity surface-emitting lasers (VCSELs), miniature laser, and polaron laser.135 Xiong et al.136 demonstrated for the first time lasing based on the whispering-gallery-mode (WGM) optical resonant cavity with hexagonal and triangular shapes of MAPbI3. Fu et al.137 used the 2D perovskite (BA)2(MA)n−1PbBr3n+1 to fabricate a WGM laser using a polydimethylsiloxane (PDMS) template. The ultrafast energy transfer together with the unique 2D perovskite quantum well (QW) structure concentrated the photogenerated carriers in the lowest-bandgap QW state. Consequently, the particle number inversion was easily established and spontaneous emission and laser amplification at room temperature were realized. The 〈n〉 = 6 sample demonstrated an ASE threshold of 13.6 μJ cm−2 and a high gain coefficient (G) at least four-times that of 3D thin films (112 cm−1). The results implied that 2D perovskites can be used for electrically driven lasers.
To enhance the stability of perovskites, Alex et al.138 used CsPbBr3 perovskite quantum dots and silica composite materials to fabricate WGM lasers. When immersed in water for 13
h, 80% of the initial emission quantum yield was maintained. Meanwhile, the thermal stability of perovskites is also very important for perovskite-based lasers. 2D materials, especially h-BN are widely regarded as ideal candidates for thermal management because of their high inherent thermal conductivity and high mechanical flexibility.139,140 A recent report showed that the surface degradation of the MAPbI3 microporous plate was inhibited and its thermal stability was greatly improved by using h-BN sheet packaging.141 Dai et al.142 synthesized CsPbI3 nanosheets on muscovite substrates via CVD as gain materials and resonators to form high-quality WGM lasers. A covered perovskite–BN composite structure was prepared via the all-dry polydimethylsiloxane (PDMS) viscoelastic stamping method, which demonstrated excellent anisotropic thermal conductivity. Consequently, the heat dissipation of the perovskite nano-laser was accelerated and achieved clear laser behavior at a temperature of up to 75.6 °C. In addition, h-BN with high environmental stability can effectively protect perovskites from polar solvents. The CsPbI3 nanolaser protected by h-BN lased continuously in water for 1 h, and the lasing behavior was maintained even after soaking in water for 24 h. The same group143 further shielded CsPbBr3 nanosheets from polar solvents via the atomic layer deposition (ALD) of Al2O3. After being coated by a thick Al2O3 layer of 50 nm, the CsPbBr3 nanosheets emitted continuously in water for more than 1 h and still emitted after soaking in water for one month. Compared with the perovskite protected by h-BN, this method is simpler and more applicable to large-scale preparation.
Perovskite materials may also emit after two-photon absorption (TPA) or multiphoton absorption (MPA), and hence are promising candidates for up conversion lasers.144,145 Perovskites with large TPA cross-sections are good optical gain materials. Zhao et al.146 reported two-photon pumped lasers with linear and plate-like MAPbBr3 microcrystals. Particularly, the unique QW structure of 2D perovskite helps to limit the electric charge carriers in the inorganic layer, thus enhancing the interaction between light and matter,147 which is suitable for producing strong TPA.
5.4 Novel memory devices
In the context of the big data era brought by the Internet and cloud computing, traditional storage devices are required to demonstrate even higher memory performances including lower power consumption and faster data processing.143 Halide perovskite has potential applications in resistance switchable memory because of its relatively low conductivity but good ion transport characteristics. However, currently, most halide perovskite resistive memory devices are based on 3D perovskite polycrystalline films, which usually contain defects on their surface and grain boundaries. Furthermore, due to their crystal structure transformation, chemical decomposition, and halide segregation, they are sensitive to oxygen and water and easily decompose. Ren et al.148 studied the light-enhanced ion migration in perovskite single crystals by constructing a stripped 2D perovskite/CNT FET (Fig. 9a), where CNTs monitored the ion redistribution within the perovskite sheet upon photoexcitation. The device successfully displayed the optical and electrical multivalued two-phase photomemory effect.
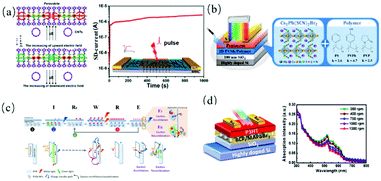 |
| Fig. 9 (a) In-plane ion migration of perovskite under an electric field and photomemory test. Adapted with permission from ref. 148. (b) Device configuration of the studied photomemory and the structures of 2D Cs2Pb(SCN)2Br2 and the host polymers. (c) Proposed operating mechanism of fully optically driven Cs2Pb(SCN)2Br2/PVP-based photomemory. Adapted with permission from ref. 151. (d) Schematic image of the studied photonic synapse and optical absorption spectra of BCP/MAPbBr3/P3HT prepared from different rotational speeds. Adapted with permission from ref. 23. | |
Given that perovskites have a high light absorption efficiency, which is desirable for optical memory, recently, a new type of optical programming transistor memory was developed using a polymer-perovskite mixed dielectric layer, showing a good optical response, suitable on/off ratio and non-volatile memory characteristics with long retention time.149,150 Through space constraints, perovskite nanocrystals embedded in the polymer matrix may act as charge trapping sites to isolate charges, and the related trapping/untrapping mechanisms can be realized by applying an electric field or using photons. Chueh et al.151 created a transistor memory through Cs2Pb(SCN)2Br2/polymer (SCN− = thiocyanide) hybrid films. The 2D Cs2Pb(SCN)2Br2 with intrinsic QW structure exhibited not only a considerable photoresponse but also good environmental stability. In addition, three host polymers with different functional groups, including polystyrene (PS), poly(4-vinylphenol) (PVPh) and polyvinyl pyrrolidone (PVP) (Fig. 9b), were systematically studied for comparison. All the manufactured devices showed light-induced memory recovery behaviors. According to the preliminary analyses, the mechanism of the programming–reading–erasing–reading function was proposed (Fig. 9c). Chen et al.23 constructed red-light-stimulated nonvolatile perovskite-based photomemory using MAPbBr3/polystyrene-block-poly(ethyleneoxide) (BCP) as the charge trapping layer and poly(3-hexylthiophene-2,5-diyl) (P3HT) as the active channel. Through the energy level mismatch and isolation effect of the polymer matrix, the photogenerated holes in P3HT and perovskite were left in the P3HT layer, while the photogenerated electrons were stored in the perovskite (Fig. 9d). Benefiting from the synergistic effect between the two components, the optical memory based on P3HT/perovskite showed optically recordable behavior in the whole visible spectrum. The response under red light was optimized by manipulating the thickness of P3HT. Due to the limitation of the exciton diffusion length, the optimized thickness of P3HT was 7.54 ± 0.42 nm, the minimum characteristic time was 36.6 s and the maximum on/off current ratio was 3.62 × 105 under red light illumination (500 mW cm−2).
5.5 Photodetectors
2D perovskites are promising candidates for optoelectronic detection due to their unique anisotropic charge carrier behaviors. Given that 2D perovskites composited with more materials can expand their functions, many fabrication strategies, such as molecular beam epitaxy and metal–organic vapor phase epitaxy, have been employed to produce transverse and longitudinal heterostructures for photodetectors. Li et al.152 prepared this type of heterostructure at low temperature through the combination of solution and gas–solid phase intercalation (Fig. 10a). However, this method only yielded polycrystals with relatively poor quality, and grains were introduced in the intercalation process, resulting in the poor performance of the photodetectors. Recently, Li's team153 developed a solution method for the synthesis of highly stable (C4H9NH3)2PbI4/(C4H9NH3)2(CH3NH3)Pb2I7 heterojunctions (Fig. 10b) with a centimeter size, high crystallization quality and narrow-band dual-frequency photoresponse. The photodetectors fabricated with this heterostructure exhibited a low dark current (∼10−12 A), high on–off current ratio (∼103), narrow full-width at half-maximum (fwhm) (less than 40 nm) and efficient narrow dual-band spectra. Dou et al.154 demonstrated a liquid-phase epitaxial growth method to improve the crystal quality of perovskite heterostructures. By inserting rigid π-conjugated organic spacers, such as dithiophenylethylammonium (2T), phenylethylammonium (PEA) and tetrathiophenylethylammonium (4Tm), the ion migration was significantly inhibited in the plane and led to atomically sharp heterostructures. Further simulations showed that conjugated ligands reduced the heterostructure disorder and increased the vacancy formation energy, thus forming a highly stable interface. Similarly, to overcome some disadvantages of 2D perovskites, such as instability, low carrier mobility and low radiation recombination efficiency,155 researchers have combined 2D perovskites with other optoelectronic materials (such as graphene, TMDs, and BP) to construct van der Waals heterostructures. For example, Atwater et al.162 prepared a heterostructure consisting of WS2 and (BA)2(MA)3P4I13 hybrid perovskite (Fig. 10c) and observed improved stability and enhanced emission two orders of magnitude greater than that of WS2. The first-principles calculation attributed the photoluminescence enhancement to the charge transfer between the interfaces originating from the dipole moment, which caused a local electric field and led to an indirect to direct band gap transition in the multilayer WS2. Likewise, graphene can also form a heterostructure with 2D perovskites to stabilize them. Rand et al.163 improved the stability of perovskite by covering a thin sheet of 2D (PEA)2PbI4 perovskite with a graphene layer to restrain the loss of iodide. An ultra-stable phototransistor based on the graphene/2D perovskite/graphene structure was demonstrated, which did not degrade for a period of 75 days (Fig. 10d).
 |
| Fig. 10 (a) Electrical measurement and stability properties of as-grown lateral and vertical heterostructures. Adapted with permission from ref. 152. (b) Schematic illustration of the device configuration based on the (BA)2PbI4/(BA)2(MA)Pb2I7 heterostructure. Adapted with permission from ref. 153. (c) Crystal structures and lattice constants of WS2 and 2D perovskites (n = 4). Adapted with permission from ref. 162. (d) SEM image of the sandwich structure of graphene/2D perovskite/graphene and the photocurrent of the device stabilized for 75 days. Adapted with permission from ref. 163. | |
The ultra-smooth surface and strong interface coupling of 2D perovskites provide an excellent platform for the design of new composite structures such as flexible materials and devices. Although progress has been made in perovskite-based flexible devices such as LEDs, photodetectors, solar cells and lasers,164,165 studies on these devices with 2D perovskite composites are relatively rare. Recently Wang et al.156 designed a transverse photodetector by stacking a double-cable conjugated polymer film on the top of the 2D perovskite (BA)2(MA)3Pb4I13, using polyimide as a flexible substrate. It displayed a high responsivity of 27.06 A W−1, high on/off ratio of 1379, and rapid rise/fall time of 3.53/3.78 ms. Table 2 presents a summary of the performance of the recently reported photodetectors based on 2D perovskite composites. In general, the stability and operation duration of these flexible devices still need to be improved.
Table 2 Comparison of the performance the reported photodetectors based on 2D perovskite composites
Materials |
Flexible base type |
Photodetector architecture |
Photoresponsivity (A W−1) |
Detectivity (Jones) |
ON/OFF ratio |
τ
rise/τfall (ms) |
EQE (%) |
Operation wavelength |
Ref. |
(PEA)2SnI42D/SnF2 |
Polyethylene terephthalate (PET) |
rGO(reduced graphene oxide)/(PEDOT:PSS) |
16 |
1.92 × 1011 |
— |
630/3600 |
— |
470 nm |
19
|
SF-PBDTPBIOD/(BA)2(MA)3Pb4I13 |
Polyimide (PI) |
— |
27.06 |
— |
1379 |
3.53/3.78 |
— |
White light |
156
|
2D CsPbBr3 |
PET/ITO |
ITO/CsPbBr3/ITO |
0.64 |
— |
>104 |
0.019/0.024 |
— |
442 nm |
32
|
(iBA)2(M1−xFAx)3Pb4I13 |
Polyimide (PI) |
— |
0.4 |
1.68 × 1012 |
720 |
43/22 |
— |
532 nm |
157
|
(BA)2(MA)4Pb4I13 |
PI |
Au/(BA)2(MA)3Pb4I13/PI |
0.17 |
3.7 × 1012 |
2.3 × 103 |
24/65 |
— |
750 nm |
158
|
CsPbBr3 nanosheets/CNTs |
PET |
Ag/CsPbBr3 nanosheets/CNT/ITO/PET |
31.1 |
— |
90 |
0.016/0.38 |
7488 |
442 nm |
159
|
CsPbBr3 nanosheets/Ti3C2Tx |
Paper |
CsPbBr3 nanosheet/Ti3C2Tx/paper |
0.044 |
6.4 × 108 |
2.3 × 103 |
— |
— |
450 nm |
160
|
CsPbBr3 |
— |
— |
3.59 |
1.57 × 1012 |
— |
— |
840 |
442 nm |
161
|
2D CsPbBr3/PCBM |
PET |
Au/CsPbBr3/PCBM/ITO/PET |
10.85 |
3.06 × 1013 |
>105 |
0.04/0.39 |
3390 |
442 nm |
161
|
5.6 Nonlinear optical applications
Many of the above-mentioned perovskite nanolasers are based on the nonlinear optical properties of the materials. However, 2D perovskites also exhibit other types of nonlinear optics such as second harmonic generation (SHG) and third harmonic generation (THG). The study of nonlinear optics began with SHG, which was discovered by Franken in 1961.166 With the development of high brightness and highly coherent laser sources, nonlinear optics has become one of the most important branches of optical research. Therefore, the study of nonlinear optical materials has fundamental scientific and technical significance.167
Studies have shown that large nonlinear optical responses are widely observed in many inorganic 2D materials, such as graphene oxide,168 MoS2,169 hexagonal boron nitride (h-BN),170 and BP.171 Our research group also performed many studies on the nonlinear optics of 2D materials.172–174 Different from ideal harmonic oscillators, a large number of thermally stable and delocalized excitons populate 2D perovskites and strongly interact. Consequently, 2D perovskites may exhibit strong THG under resonant excitation.13 Abdelwahab et al.175 mechanically exfoliated 2D perovskite nanosheets (Fig. 11a), which exhibited ultra-strong THG with a maximum effective third-order susceptibility (χ(3)) of 1.12 × 10−17 m2 V−2 and maximum conversion efficiency of 0.006%.
 |
| Fig. 11 (a) Structure of different 2D RPP crystals and the fundamental wavelength dependence of the THG spectra of the most effective flakes. Adapted with permission from ref. 175. (b) SHG of chiral perovskites. Adapted with permission from ref. 176. (c) Schematic diagram of the ring cavity of the mode-locked fiber laser. Adapted with permission from ref. 180. (d) Mode-locking using 2D (C6H5C2H4NH3)2PbI4 saturable absorber. Adapted with permission from ref. 181. | |
For the specific centrosymmetric structure of 2D layered perovskites, it is difficult to produce a strong second-order nonlinear optical response. However, by incorporating 2D perovskites and organic materials (such as varying different ammonium functional groups), the structural symmetry can be adjusted. Xu et al.176 used the chiral molecule of methylphenylethylamine (MPEA) as an organic component to induce the non-centrosymmetric assembly of 2D inorganic layers (Fig. 11b). The 2D inorganic layers and perovskite crystals were assembled into a chiral P1 space group by anti-solvent vapor-assisted crystallization (AVC) with a structure of (MPEA)1.5PbBr3.5(DMSO)0.5, where DMSO was dimethyl sulfoxide. Then novel 2D perovskite nanowires were grown by anti-solvent-assisted crystallization in the ternary solvent system, which showed a strong SHG response with high polarizability. Therefore, the introduction of different ammonium groups as organic spacers provides a general platform for the functionalization of 2D perovskites, giving them tunable nonlinear optical characteristics. SHG was also used to detect the interaction between photons and local structures of 2D perovskites. Lu et al.177 found that the SHG intensity of 2D [(C6H5CH2NH3)2]PbCl4 depended on the thickness of the nanowires. DFT calculations and Monte Carlo (MC) simulations implied that the van der Waals interaction from flexible organic amine cations played a key role.178
The nonlinear saturable absorption of perovskite materials is of great use in mode-locked lasers. Perovskites can be inserted into the laser cavity by depositing on the end face of the optical fiber connector or the side polished surface of the D-shaped optical fiber for mode-locking.179 Li et al.180 used a single 2D CH3NH3PbI3 nanosheet as the saturator for a 1064 nm ytterbium-doped fiber laser (Fig. 11c). Compared with the control films, the 2D perovskite nanosheets had stronger saturable absorption, larger modulation depth and lower saturable strength. In particular, the perovskite nanosheets with a thickness of 105 nm had the highest modulation depth of 22.2% and the lowest saturation strength of about 1.8 × 103 GW cm−2. The fwhm of the stable mode-locked pulse output pulse was 931 ps, the signal-to-noise ratio was more than 53 dB, the peak power was 4.14 W, and the pulse energy was 3.85 nJ. Hong et al.181 constructed an all-fiber ring laser cavity using a 2D perovskite microcrystalline film of (C6H5C2H4NH3)2PbI4 as the saturated absorber and erbium-doped fiber as the optical gain medium (Fig. 11d) obtain transform-limited soliton pulses in the C and L bands under abnormal dispersion. The C-band soliton pulse showed a very short pulse duration of 381 fs and a spectral bandwidth of 7 nm with a central wavelength of 1565.9 nm and a high signal-to-noise ratio of more than 89 dB at a repetition rate of 41.88 MHz. Furthermore, L-band soliton pulses with a 6 nm spectral bandwidth were stably obtained at 1604 nm by adjusting the optical gain.
Lasers can impose great damage to optoelectronic devices and the human body (especially human eyes), and thus are also used as laser weapons. Laser protection has been a research frontier regardless of civilian or military use. Among them, optical limiters can reduce the transmittance of strong light and maintain weak light, well protecting the human eyes and precision optical sensors from damage caused by high-intensity light. Perovskite is a potential laser radiation-limiting material because of its two-photon and multiphoton absorption. Pai et al.182 reported the optical confinement behavior of a lead benzylamine(II) perovskite bromide microdisk from strong TPA under 800 nm fs-laser excitation. A limiting offset of 1.2 mJ cm−2 was obtained, and the surmised optical limiting threshold of ∼25.6 mJ cm−2 was comparable to that of benchmark materials such as 2D MoS2. Thomas et al.183 also reported 2D RP organic–inorganic hybrid perovskite quantum dots with high optical confinement. Optical z-scanning experiments showed that the maximum optical limitation was 67% at 175 μJ under a 532 nm ns laser. The effective two-photon coefficient was 7.2 × 102 cm GW−1.
Although 2D perovskites show remarkable nonlinear optical properties, especially their nonlinear absorption properties including saturated absorption and two-photon or multiphoton absorption, the stability of these materials remains the main obstacle for their practical applications. Accordingly, by compounding with different materials, such as adding organic functional cations, recombination with inorganic materials, and ion doping, the stability of perovskites may be effectively improved. The composite structures are expected to expand the nonlinear optical applications of 2D perovskites.
5.7 Space applications
Mankind invented various spacecrafts to explore outer space, such as the International Space Station (ISS) and the Tianwen-1 Mars Probe. However, the harsh space environment featuring ultra-high vacuum, extreme temperature and containment of high-energy charged particles and rays174 puts a high demand on equipment. Spacecraft mainly use space solar cells for energy supply. In theory, perovskite solar cells are particularly suitable for use in space due to their high PCE of above 29.1%,184 high specific power due to sub-micron-thick absorber, low-temperature solution processability and radiation resistance. However, the study of perovskite solar cells for space applications is still in its infancy. The first report proposing this concept dates back to 2015 by Hirose and colleagues.185 In their work, the resistance of perovskite solar cells to electron irradiation was investigated, where a 1 MeV electron irradiation source was used with a dose of 1 × 1016 particle cm−2 (p cm−2). Using quartz as the substrate material, the performance of the device, such as short-circuit current (JSC), open circuit voltage (VOC) and EQE, did not decrease under electron radiation. Since then, numerous experiments have been carried out to examine the tolerance of PSC to various space irradiation, such as electron,186 proton,187,188 and γ-ray irradiations,189,190 and neutrons.191 Yan et al.186 reported the excellent tolerance of perovskite solar cells under electron irradiation. After high-fluence electron irradiation of 1015 e cm−2, the cells maintained high remaining factors of >87.7% in VOC and >93.5% in the fill factor (FF) (Fig. 12a). However, the JSC drastically decreased as the transmittance of substrate decreased and perovskite absorber layers decomposed partially. Neitzert et al.188 fabricated an inverted perovskite solar cell in a glass/ITO/PEDOT:PSS/CH3NH3PbI3/PCBM/BCP/Ag layer sequence with a stable PCE of 12.1% (Fig. 12b). The device was irradiated with 68 MeV protons until the total dose of 1.02 × 1013 p cm−2 was reached. The solar cells did not show any degradation at the proton dose of ≤2 × 1011 p cm−2. At the proton dose of 1012 and 1013 p cm−2, the JSC decreased by 10% and 40%, respectively. Therefore, the perovskite-type absorber withstood proton doses of up to 1012 p cm−2, nearly 3 orders of magnitude higher than the damage threshold of c-Si. Moreover, the self-healing process of perovskite began and JSC recovered once the proton irradiation ended. The fact that the CH3NH3PbI3 perovskite showed both radiation resistance and self-repair ability make this type of solar cell very attractive in space applications.
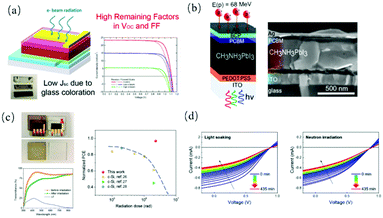 |
| Fig. 12 (a) Schematic illustration of the perovskite solar cell and J–V curve. Adapted with permission from ref. 186. (b) Sketch of the perovskite solar cell and cross-sectional scanning electron microscopy image. Adapted with permission from ref. 188. (c) Transmission spectra of the device and ITO glass after gamma-ray irradiation, and comparison of the normalized PCE as a function of gamma-ray radiation dose for perovskite and crystal silicon solar cells. Adapted with permission from ref. 189. (d) I–V characteristics under light-soaking and neutron irradiation. Adapted with permission from ref. 191. | |
Huang et al.189 irradiated perovskite solar cells under continuous light and gamma rays (cumulative dose of 2.3 Mrad) for 1535 h and found that they maintained excellent stability compared with glass or crystal silicon (Fig. 12c). The self-healing behavior accounted for the gamma-ray irradiation resistance given that the defect density characterization indicated that the irradiation did not impose electronic trap states. In addition, neutrons are also a type of radiation that affects aircraft. The International Space Station receives about ≈2.8 × 1011 neutrons cm−2 per year. Cassiali et al.191 reported a study on fast neutron irradiation on perovskite solar cells and found that the equipment was resilient to the dangerous radiation in space. Fast neutrons can permanently deteriorate the device performance due to the atomic displacement damage. However, the formation of neutron-induced shallow traps may work as dopants to increase the open-circuit voltage and decrease the leakage current to reduce the radiation damage on the device (Fig. 12d).
Another type of ray in space is X-rays,192 which are electromagnetic radiation with a very short wavelength between ultraviolet and gamma rays. Metal halide perovskites of MAPbBr3 were applied as semiconductor-based X-ray detectors.193 3D perovskite CsPbBr3 nanocrystals194 and nanosheets195 have been reported as scintillator screens in X-ray imaging.196 However, their practical applications remain plagued by the instability and low density of 3D perovskite nanomaterials. Accordingly, 2D bulk perovskites may offer alternative solutions. Dang et al.197 used lithium chemical doping in a 2D (PEA)2PbBr4 crystal and the scintillation mechanism was explored through temperature-dependent X-ray and thermoluminescence measurements. The crystal demonstrated a fast decay time of 11 ns (80%), a sharp peak with 12.4% energy resolution, and a scintillation yield of 11
000 photons per MeV under 662 keV gamma radiation.
It should be noted that perovskite-based nonlinear optical devices (optical limiters and saturable absorbers for mode-locking) may also find applications in space lasers and laser protection, but their study remains scant.174
6. Perspectives
Herein, we reviewed the latest development on 2D perovskite composites and their versatile applications. Although great progress has been made in the past few years, the research based on 2D perovskite composite structures is still in its infancy. Future studies should focus on the following issues.
AI-assisted functionalization of 2D halide perovskites
To fully realize the potential 2D perovskite composites and achieve the “1 + 1 > 2” effect, further methodologies of functionalization of 2D perovskites are necessary. 2D halide perovskites provide a good platform for defect regulation and surface functionalization given that most of their atoms are exposed to the external environment. In addition, because of their ultra-smooth surface and strong interface coupling, composite structures of ultra-thin 2D perovskites can be designed flexibly without considering the lattice mismatch.198 Alternatively, the long-term stability of 2D perovskites is still a great challenge. Currently, the following strategies are mostly employed: (1) rationally selecting polymers with specific functional groups and anchoring them on the perovskite surface, thus preventing the perovskites from damage caused by light, high temperature, moisture and other polar solvents and (2) directly stacking perovskites with graphene, h-BN, TMDs and other 2D materials.
In this context, artificial intelligence (AI)-assisted functionalization has proven to be very effective for the rational design of 2D perovskite composites and is the future trend. The trial-and-error method based on artificial experience has become a powerful method to design new materials. At present, there are more than 40
000 papers on chemical materials assisted by machine learning.199,200 Generally, organic cationic structures can be artificially designed and compounded with functional materials to improve the performance of 2D perovskites. Identifying the key units conducive to high performance and establishing the relationship between substructure and performance is very important for the development of new materials. However, AI alone cannot meet this challenge, and thus constant and collective efforts from both theorists and experimentalists are needed.201
Combination of new characterization techniques and theoretical calculations for deep understanding of the new physics of perovskites
Understanding the interaction between perovskites and functional materials (such as polymers) within composites is crucial for the development of new hybrid structures. For instance, questions such as which functional groups of the polymer passivate the trap state of 2D perovskites and how the polymer affects the termination and surface energy of perovskites are frequently met in research. In addition, it is necessary to clarify how the chain packing and composition of polymers affect the diffusion of water and oxygen, as well as the overall contribution of polymers to the electronic band structure and stability. Thus, to tackle these issues, surface analysis techniques including vibrational spectroscopy, XPS, small-angle neutron scattering techniques and NMR are very effective tools. Meanwhile, time-resolved spectroscopy is the key technique for investigating excited-state dynamics and identifying the correlation between the structural and optoelectronic properties of perovskite composites, while space-resolved techniques can detect the properties of different parts of materials. The combination of time and space resolution enables the study of dynamic processes in different positions in materials. For instance, 4D ultrafast electron microscopy (4D TEM) may directly visualize perovskites and realize simultaneous atomic spatial resolution (angstrom) and femtosecond time resolution.202,203 In summary, multi-dimensional, cross-scale, high-resolution and in situ characterization techniques are urgently needed for the observation of perovskite composites.
Alternatively, computation/simulation technology is very effective to promote the understanding of the interaction of composites and the development of nanometer composites with reinforced properties. For example, DFT and molecular dynamics are expected to play increasingly important roles in investigating exciton behaviours, carrier dynamics, surface defects, interactions between surface and functional molecules of perovskites, etc.
Perovskite-based miniaturized and flexible devices for future IT
With the rise of new information technologies such as artificial intelligence and the Internet of Things, information processing has shifted from computing-intensive to data-intensive. Low-latency and low-energy edge computing systems are urgently needed to meet the needs of terminal devices for massive unstructured data processing capabilities in the future. For instance, biologically inspired impulsive neural networks (SNN) use sparse and asynchronous pulse sequences as input/output and process information via in-memory computing, which has the characteristics of large-scale parallelism and low energy consumption. Thus, to fully utilize the advantages of SNN, new synaptic electronic devices with compact and low power consumption need to be developed in hardware. Recently, biomimetic artificial synapses have made great progress, in which the two-port memristor has the advantages of simple structure and good integration, and thus regarded as an ideal carrier of bionic synapses.204 The counterion of halide perovskite is movable under an electric field, which makes it very promising in the memristor field. Based on the excellent light absorption capacity of perovskites, these memristors can also provide novel functions through the collaborative coupling of photon, electron and ion processes. 3D halide perovskites (MA+, FA+ and Cs+ as the main monovalent cations) were the earliest and most commonly used in memristors,205 but their stability and durability are limiting parameters. The use of 2D perovskites and their composites have recently emerged80 but these materials have been proven to have great potential in low-cost and flexible memristors.
Ever-lasting expansion of applications
Similar to an “all-around” potent material, the application fields of 2D perovskite composites keep expanding. Photocatalysis such as photocatalytic CO2 reduction206 and water decomposition207 is one new field they have exceled in. Composites based on perovskite nanocrystals and low-dimensional materials (such as BP,208 graphene oxide, and MXenes) demonstrated efficacy as photocatalytic catalysts. Our group has recently combined CsPbBr3 quantum dots and a 2D copper-based porphyrin MOF to construct 0D/2D hybrid catalysts for the highly efficient photocatalytic reduction of CO2. Currently most of the reported perovskites are inorganic CsPbBr3 perovskites, whereas layered 2D perovskites are much less reported.25 However, the latter, with relatively higher stability and enhanced carrier separation efficiency209 by introducing organic compounds, is considered more promising for photocatalysis. Moreover, controlling the growth of 2D perovskites and reducing the distance between inorganic layers may also help to improve the vertical charge transfer.
The unique bonding structure of 2D composites implies new physics and applications. Chiral 2D perovskites can be obtained by introducing chiral organic ligands into the framework. Our group reported the first example of 2D hybrid perovskite chiral ferromagnets of (R-MPEA)2CuCl4 and (S-MPEA)2CuCl4 (MPEA = methylphenethylamine), which showed strong oppositely signed circular dichroism signals and ferromagnetic behaviors.210 The carriers in chiral 2D perovskites are self-polarized, and hence constructing chiral 2D perovskite composites with functional materials such as monolayer TMD can control valley polarization, providing a promising platform for spintronics. However, the spin injection process and related physical processes are unclear, which is an interesting but not widely studied field.211
Due to the rich structural diversity and “soft crystal” nature of 2D perovskites, magnetism and thermochromism can be integrated through the chemical adjustment of their organic spacer cations and metal ions,212 which offers the possibility of using these composites not only in optoelectronic devices but also in sensing applications such as monitoring of dopamine,213 CBr4,214 temperature,215etc. In addition, doping rare earth elements can realize the potential of 2D perovskites as light/heat switchable markers and high-efficiency thermometers in biomedical imaging and diagnosis. The record photoluminescence and electroluminescence efficiency216 of 2D perovskites has continuously been broken in displays, encryption, biological imaging and X-ray detection.52,217,218 For instance, Chandra et al.219 reported the use of specific antibody-attached water-resistant CsPbBr3 nanocrystals in different dimensions for two-photon imaging of breast cancer cells in the near IR region, which is superior to conventional organic fluorescence labels.
Finally, scaling-up (such as large-scale continuous) production is critical for 2D perovskite composites to move closer to commercial applications.220 In summary, 2D halide perovskite composites have shown great potential and researchers have made continuous progress in terms of their synthesis, fundamental understanding of composition–structure–property relationships, and various applications beyond optoelectronic use. We provided our insights into the future trend of this exciting field, which will hopefully promote joint efforts from research groups of different backgrounds to tackle the current challenges.
Conflicts of interest
There are no conflicts to declare.
Acknowledgements
This work is supported by the National Natural Science Foundation of China (NSFC 22073038, 51525303, 21233001), Key Research and Development Program of Gansu Province (20YF3WA013), Open Fund of National Key Laboratory of Materials Behavior and Evaluation Technology in Space Environment (6142910200106) and Supercomputing Center of Lanzhou University.
Notes and references
- C. Zhou, Y. Tian, M. Wang, A. Rose, T. Besara, N. K. Doyle, Z. Yuan, J. C. Wang, R. Clark, Y. Hu, T. Siegrist, S. Lin and B. Ma, Angew. Chem., Int. Ed., 2017, 56, 9018–9022 CrossRef CAS PubMed.
- G. H. Ahmed, J. Yin, R. Bose, L. Sinatra, E. Alarousu, E. Yengel, N. M. AlYami, M. I. Saidaminov, Y. Zhang, M. N. Hedhili, O. M. Bakr, J.-L. Brédas and O. F. Mohammed, Chem. Mater., 2017, 29, 4393–4400 CrossRef CAS.
- J. Qian, Q. Guo, L. Liu, B. Xu and W. Tian, J. Mater. Chem. A, 2017, 5, 16786–16795 RSC.
- J. A. Sichert, Y. Tong, N. Mutz, M. Vollmer, S. Fischer, K. Z. Milowska, R. García Cortadella, B. Nickel, C. Cardenas-Daw, J. K. Stolarczyk, A. S. Urban and J. Feldmann, Nano Lett., 2015, 15, 6521–6527 CrossRef CAS PubMed.
- Y. Bekenstein, B. A. Koscher, S. W. Eaton, P. Yang and A. P. Alivisatos, J. Am. Chem. Soc., 2015, 137, 16008–16011 CrossRef CAS PubMed.
- B. Vargas, E. Ramos, E. Pérez-Gutiérrez, J. C. Alonso and D. Solis-Ibarra, J. Am. Chem. Soc., 2017, 139, 9116–9119 CrossRef CAS PubMed.
- C. M. M. Soe, C. C. Stoumpos, M. Kepenekian, B. Traoré, H. Tsai, W. Nie, B. Wang, C. Katan, R. Seshadri, A. D. Mohite, J. Even, T. J. Marks and M. G. Kanatzidis, J. Am. Chem. Soc., 2017, 139, 16297–16309 CrossRef CAS PubMed.
- L. Mao, W. Ke, L. Pedesseau, Y. Wu, C. Katan, J. Even, M. R. Wasielewski, C. C. Stoumpos and M. G. Kanatzidis, J. Am. Chem. Soc., 2018, 140, 3775–3783 CrossRef CAS PubMed.
- C. C. Stoumpos, D. H. Cao, D. J. Clark, J. Young, J. M. Rondinelli, J. I. Jang, J. T. Hupp and M. G. Kanatzidis, Chem. Mater., 2016, 28, 2852–2867 CrossRef CAS.
- C. C. Stoumpos, C. M. M. Soe, H. Tsai, W. Nie, J.-C. Blancon, D. H. Cao, F. Liu, B. Traoré, C. Katan, J. Even, A. D. Mohite and M. G. Kanatzidis, Chem, 2017, 2, 427–440 CAS.
- S. Sun, D. Yuan, Y. Xu, A. Wang and Z. Deng, ACS Nano, 2016, 10, 3648–3657 CrossRef CAS PubMed.
- L. Mao, C. C. Stoumpos and M. G. Kanatzidis, J. Am. Chem. Soc., 2019, 141, 1171–1190 CrossRef CAS PubMed.
- Z. Gan, Y. Cheng, W. Chen, K. P. Loh, B. Jia and X. Wen, Adv. Sci., 2021, 8, 2001843 CrossRef CAS PubMed.
- A. Kojima, K. Teshima, Y. Shirai and T. Miyasaka, J. Am. Chem. Soc., 2009, 131, 6050–6051 CrossRef CAS PubMed.
- M. Yuan, L. N. Quan, R. Comin, G. Walters, R. Sabatini, O. Voznyy, S. Hoogland, Y. Zhao, E. M. Beauregard, P. Kanjanaboos, Z. Lu, D. H. Kim and E. H. Sargent, Nat. Nanotechnol., 2016, 11, 872–877 CrossRef CAS PubMed.
- A. Z. Chen and J. J. Choi, J. Vac. Sci. Technol., A, 2020, 38, 010801 CrossRef CAS.
- Y. Chen, Y. Sun, J. Peng, J. Tang, K. Zheng and Z. Liang, Adv. Mater., 2018, 30, 1703487 CrossRef PubMed.
- X. Fu, B. Chen, J. Tang, M. T. Hassan and A. H. Zewail, Science, 2017, 355, 494–498 CrossRef CAS PubMed.
- L. Qian, Y. Sun, M. Wu, C. Li, D. Xie, L. Ding and G. Shi, Nanoscale, 2018, 10, 6837–6843 RSC.
- C. Gu and J.-S. Lee, ACS Nano, 2016, 10, 5413–5418 CrossRef CAS PubMed.
- K. Meng, L. Wu, Z. Liu, X. Wang, Q. Xu, Y. Hu, S. He, X. Li, T. Li and G. Chen, Adv. Mater., 2018, 30, 1706401 CrossRef PubMed.
- X.-P. Zhai, B. Ma, Q. Wang and H.-L. Zhang, Phys. Chem. Chem. Phys., 2020, 22, 22140–22156 RSC.
- H.-T. Hsu, D.-L. Yang, L. D. Wiyanto and J.-Y. Chen, Adv. Photonics Res., 2021, 2, 2000185 CrossRef.
- Z. Chu, Q. Ye, Y. Zhao, F. Ma, Z. Yin, X. Zhang and J. You, Adv. Mater., 2021, 33, 2007169 CrossRef CAS PubMed.
- N. Li, X.-P. Zhai, W.-K. Yan, Y.-J. Zhang, Z.-T. Zhang, M.-J. Xiao, X.-D. Zhang, Q. Wang and H.-L. Zhang, Sol. RRL, 2021, 5, 2100558 CrossRef CAS.
- W. Haizhen, M. Jiaqi and L. Dehui, J. Phys. Chem. Lett., 2021, 12, 8178–8187 CrossRef PubMed.
- C. Tan, X. Cao, X.-J. Wu, Q. He, J. Yang, X. Zhang, J. Chen, W. Zhao, S. Han, G.-H. Nam, M. Sindoro and H. Zhang, Chem. Rev., 2017, 117, 6225–6331 CrossRef CAS PubMed.
- C. Chang, Y. Chen, Y.-H. Chen, Y. Chen, F. Ding, C.-H. Fan, H.-J. Fan, Z.-X. Fan, C. Gong, Y.-J. Gong, Q.-Y. He, X. Hong, S. Hu, W.-D. Hu, W. Huang, Y. Huang, W. Ji, D.-H. Li, L.-J. Li, Q. Li, L. Lin, C.-Y. Ling, M.-H. Liu, N. Liu, Z. Liu, K.-P. Loh, J.-M. Ma, F. Miao, H.-L. Peng, M.-F. Shao, L. Song, S. Su, S. Sun, C.-L. Tan, Z. D. Tang, D.-S. Wang, H. Wang, J.-L. Wang, X. Wang, X.-R. Wang, A. T. S. Wee, Z. M. Wei, Y. Wu, Z.-S. Wu, J. Xiong, Q.-H. Xiong, W.-G. Xu, P. Yin, H.-B. Zeng, Z.-Y. Zeng, T.-Y. Zhai, H. Zhang, H. Zhang, Q.-C. Zhang, T. R. Zhang, X. Zhang, L.-D. Zhao, M.-T. Zhao, W.-J. Zhao, Y.-X. Zhao, K.-G. Zhou, X. Zhou, Y. Zhou, H.-W. Zhu, H. Zhang and Z.-F. Liu, Acta Phys. – Chim. Sin., 2021, 37, 2108017 Search PubMed.
- F. Chen, W. Haizhen, S. Zixi, S. Hongzhi, W. Shuai, M. Jiaqi, W. Jun, L. Hongmei and L. Dehui, ACS Appl. Mater. Interfaces, 2019, 11, 8419–8427 CrossRef.
- E. Z. Shi, Y. Gao, B. P. Finkenauer, Akriti, A. H. Coffey and L. T. Dou, Chem. Soc. Rev., 2018, 47, 6046–6072 RSC.
- A. G. Ricciardulli, S. Yang, J. H. Smet and M. Saliba, Nat. Mater., 2021, 20, 1325–1336 CrossRef CAS PubMed.
- J. Song, L. Xu, J. Li, J. Xue, Y. Dong, X. Li and H. Zeng, Adv. Mater., 2016, 28, 4861–4869 CrossRef CAS PubMed.
- J. Shamsi, Z. Dang, P. Bianchini, C. Canale, F. D. Stasio, R. Brescia, M. Prato and L. Manna, J. Am. Chem. Soc., 2016, 138, 7240–7243 CrossRef CAS PubMed.
- A. Pan, B. He, X. Fan, Z. Liu, J. J. Urban, A. P. Alivisatos, L. He and Y. Liu, ACS Nano, 2016, 10, 7943–7954 CrossRef CAS PubMed.
- L. Dou, A. B. Wong, Y. Yu, M. Lai, N. Kornienko, S. W. Eaton, A. Fu, C. G. Bischak, J. Ma, T. Ding, N. S. Ginsberg, L.-W. Wang, A. P. Alivisatos and P. Yang, Science, 2015, 349, 1518–1521 CrossRef CAS PubMed.
- S. Enzheng, Y. Biao, B. S. Stephen, G. Yao, Akriti, G. Yunfan, S. Cong, L. Minliang, Y. Peidong, K. Jing, M. S. Brett, Y. Yi and D. Letian, Nature, 2020, 580, 614–620 CrossRef PubMed.
- W. Deng, X. Jin, Y. Lv, X. Zhang, X. Zhang and J. Jie, Adv. Funct. Mater., 2019, 29, 1903861 CrossRef.
- L. Ma, C. Wang, Y. Chu, Y. Guo, X. Feng, Z. Yu, H. Zhang and G. Zhao, 2D Mater., 2021, 8, 021003 CrossRef CAS.
- M. Y. Kuo, N. Spitha, M. P. Hautzinger, P. L. Hsieh, J. Li, D. Pan, Y. Zhao, L. J. Chen, M. H. Huang, S. Jin, Y. J. Hsu and J. C. Wright, J. Am. Chem. Soc., 2021, 143, 4969–4978 CrossRef CAS PubMed.
- Y. Wang, Y. Shi, G. Xin, J. Lian and J. Shi, Cryst. Growth Des., 2015, 15, 4741–4749 CrossRef CAS.
- Y. Z. Xue, J. Yuan, J. Y. Liu and S. J. Li, Nanomaterials, 2018, 8, 591–595 CrossRef PubMed.
- K. Ming-Yu, S. Natalia, P. H. Matthew, H. Pei-Lun, L. Jing, P. Dongxu, Z. Yuzhou, C. Lih-Juann, H. H. Michael, J. Song, H. Yung-Jung and C. W. John, J. Am. Chem. Soc., 2021, 143, 4969–4978 CrossRef PubMed.
- S. T. Ha, X. Liu, Q. Zhang, D. Giovanni, T. C. Sum and Q. Xiong, Adv. Mater., 2014, 2, 838–844 CAS.
- Y. Chen, Y. Sun, J. Peng, J. Tang, K. Zheng and Z. Liang, Adv. Mater., 2018, 30, 1703487 CrossRef PubMed.
- Z. Liu, L. Ma, G. Shi, W. Zhou, Y. Gong, S. Lei, X. Yang, J. Zhang, J. Yu, K. P. Hackenberg, A. Babakhani, J.-C. Idrobo, R. Vajtai, J. Lou and P. M. Ajayan, Nat. Nanotechnol., 2013, 8, 119–124 CrossRef CAS PubMed.
- T. Zhenjun, W. Yue, H. Hao, Y. Jianbo, Z. Jincan, L. Li, W. Mingzhan, S. Xiao, S. Luzhao, H. Yucheng, L. Kaihui, L. Zhongfan and P. Hailin, J. Am. Chem. Soc., 2016, 138, 16612–16615 CrossRef PubMed.
- H.-C. Cheng, G. Wang, D. Li, Q. He, A. Yin, Y. Liu, H. Wu, M. Ding, Y. Huang and X. Duan, Nano Lett., 2016, 16, 367–373 CrossRef CAS PubMed.
- L. Junze, W. Jun, M. Jiaqi, S. Hongzhi, L. Lu, D. Xiangfeng and L. Dehui, Nat. Commun., 2019, 10, 806 CrossRef PubMed.
- Y. Sun, Y. Yin, M. Pols, J. Zhong, Z. Huang, B. Liu, J. Liu, W. Wang, H. Xie, G. Zhan, Z. Zhou, W. Zhang, P. Wang, C. Zha, X. Jiang, Y. Ruan, C. Zhu, G. Brocks, X. Wang, L. Wang, J. Wang, S. Tao and W. Huang, Adv. Mater., 2020, 32, 2002392 CrossRef CAS PubMed.
- W. Qixing, Z. Qi, L. Xin, W. Junyong, Z. Rui, L. Qijie, Z. Lei, Y. Justin Zhou, W. Calvin Pei Yu, E. Goki, H. S. Jurgen and T. S. W. Andrew, ACS Appl. Mater. Interfaces, 2020, 12, 45235–45242 CrossRef PubMed.
- Y.-T. Li, L. Ding, J.-Z. Li, J. Kang, D.-H. Li, L. Ren, Z.-Y. Ju, M.-X. Sun, J.-Q. Ma, Y. Tian, G.-Y. Gou, D. Xie, H. Tian, Y. Yang, L.-W. Wang, L.-M. Peng and T.-L. Ren, ACS Cent. Sci., 2019, 5, 1857–1865 CrossRef CAS PubMed.
- H. Zhang, J. Yao, Y. Yang and H. Fu, Chem. Mater., 2021, 33, 2847–2854 CrossRef CAS.
- S. Liang, M. Zhang, G. M. Biesold, W. Choi, Y. He, Z. Li, D. Shen and Z. Lin, Adv. Mater., 2021 DOI:10.1002/adma.202005888.
- C. G.-R. María, B. F. Magnus, K. D. Rajeev, F. J. Wolter, S. Ward van der and C. G. Ferdinand, Nat. Commun., 2020, 11, 1901 CrossRef PubMed.
- Z. Li, L. Kong, S. Huang and L. Li, Angew. Chem., Int. Ed., 2017, 56, 8134–8138 CrossRef CAS PubMed.
- D. N. Dirin, L. Protesescu, D. Trummer, I. V. Kochetygov, S. Yakunin, F. Krumeich, N. P. Stadie and M. V. Kovalenko, Nano Lett., 2016, 16, 5866–5874 CrossRef CAS PubMed.
- Y. He, Y. Liang, S. Liang, Y. W. Harn, Z. Li, M. Zhang, D. Shen, Z. Li, Y. Yan, X. Pang and Z. Lin, Angew. Chem., Int. Ed., 2021, 60, 7259–7266 CrossRef CAS PubMed.
- V. P. James, J. F. Daniel, A. S. Nicholas, P. H. Mark, S. Hiroaki, L. S. Charlotte and I. S. Samuel, J. Am. Chem. Soc., 2018, 140, 7313–7323 CrossRef PubMed.
- B. Saparov and D. B. Mitzi, Chem. Rev., 2016, 116, 4558–4596 CrossRef CAS PubMed.
- T. M. V. G. Wouter, H. Roald, H. Kristof Van, R. Bart, D. H. Jan, L. Laurence and V. Dirk, Chem. Commun., 2019, 55, 2481–2484 RSC.
- C. G.-R. María, T. M. V. G. Wouter, H. Roald, L. Laurence, V. Dirk and C. G. Ferdinand, J. Phys. Chem. Lett., 2020, 11, 824–830 CrossRef PubMed.
- W. Yuanzhi, F. Guitao, M. Peng, L. Yigang, Z. Jing, C. Ningli, Y. Haixia, L. Weiwei, Y. Shiyong and W. Jizheng, ACS Appl. Mater. Interfaces, 2020, 12, 8826–8834 CrossRef PubMed.
- X. Gong, O. Voznyy, A. Jain, W. Liu, R. Sabatini, Z. Piontkowski, G. Walters, G. Bappi, S. Nokhrin, O. Bushuyev, M. Yuan, R. Comin, D. McCamant, S. O. Kelley and E. H. Sargent, Nat. Mater., 2018, 17, 550–556 CrossRef CAS PubMed.
- Y.-H. Kim, S. Kim, A. Kakekhani, J. Park, J. Park, Y.-H. Lee, H. Xu, S. Nagane, R. B. Wexler, D.-H. Kim, S. H. Jo, L. Martínez-Sarti, P. Tan, A. Sadhanala, G.-S. Park, Y.-W. Kim, B. Hu, H. J. Bolink, S. Yoo, R. H. Friend, A. M. Rappe and T.-W. Lee, Nat. Photonics, 2021, 15, 148–155 CrossRef CAS.
- M. Saliba, J.-P. Correa-Baena, M. Grätzel, A. Hagfeldt and A. Abate, Angew. Chem., Int. Ed., 2018, 57, 2554–2569 CrossRef CAS PubMed.
- S. De Wolf, J. Holovsky, S.-J. Moon, P. Löper, B. Niesen, M. Ledinsky, F.-J. Haug, J.-H. Yum and C. Ballif, J. Phys. Chem. Lett., 2014, 5, 1035–1039 CrossRef CAS PubMed.
- C. Huo, B. Cai, Z. Yuan, B. Ma and H. Zeng, Small Methods, 2017, 1, 1600018 CrossRef.
- Z. Tan, Y. Wu, H. Hong, J. Yin, J. Zhang, L. Lin, M. Wang, X. Sun, L. Sun, Y. Huang, K. Liu, Z. Liu and H. Peng, J. Am. Chem. Soc., 2016, 138, 16612–16615 CrossRef CAS PubMed.
- N. N. Wang, L. Cheng, R. Ge, S. T. Zhang, Y. F. Miao, W. Zou, C. Yi, Y. Sun, Y. Cao, R. Yang, Y. Q. Wei, Q. Guo, Y. Ke, M. T. Yu, Y. Z. Jin, Y. Liu, Q. Q. Ding, D. W. Di, L. Yang, G. C. Xing, H. Tian, C. H. Jin, F. Gao, R. H. Friend, J. P. Wang and W. Huang, Nat. Photonics, 2016, 10, 699–704 CrossRef CAS.
- L. N. Quan, Y. Zhao, F. P. Garcia de Arquer, R. Sabatini, G. Walters, O. Voznyy, R. Comin, Y. Li, J. Z. Fan, H. Tan, J. Pan, M. Yuan, O. M. Bakr, Z. Lu, D. H. Kim and E. H. Sargent, Nano Lett., 2017, 17, 3701–3709 CrossRef CAS PubMed.
- J. Xing, Y. B. Zhao, M. Askerka, L. N. Quan, X. W. Gong, W. J. Zhao, J. X. Zhao, H. R. Tan, G. K. Long, L. Gao, Z. Y. Yang, O. Voznyy, J. Tang, Z. H. Lu, Q. H. Xiong and E. H. Sargent, Nat. Commun., 2018, 9, 3541 CrossRef PubMed.
- M. Ahmadi, T. Wu and B. Hu, Adv. Mater., 2017, 29, 1605242 CrossRef PubMed.
- M. D. Smith, L. Pedesseau, M. Kepenekian, I. C. Smith, C. Katan, J. Even and H. I. Karunadasa, Chem. Sci., 2017, 8, 1960–1968 RSC.
- J. C. Blancon, J. Even, C. C. Stoumpos, M. G. Kanatzidis and A. D. Mohite, Nat. Nanotechnol., 2020, 15, 969–985 CrossRef CAS PubMed.
- H. L. Wang, W. Z. Lv, X. X. Tang, L. F. Chen, R. F. Chen and W. Huang, Prog. Chem., 2017, 29, 859–869 CAS.
- X. Qi, Y. Zhang, Q. Ou, S. T. Ha, C.-W. Qiu, H. Zhang, Y.-B. Cheng, Q. Xiong and Q. Bao, Small, 2018, 14, 1800682 CrossRef PubMed.
- H. Peiman, C. D. Wright and B. Harish, Nature, 2014, 511, 206–211 CrossRef PubMed.
- X. Zhao, H. Xu, Z. Wang, Y. Lin and Y. Liu, InfoMat, 2019, 1, 183–210 CrossRef CAS.
- Z. Xiao and J. Huang, Adv. Electron. Mater., 2016, 2, 1600100 CrossRef.
- H. Tian, L. Zhao, X. Wang, Y.-W. Yeh, N. Yao, B. P. Rand and T.-L. Ren, ACS Nano, 2017, 11, 12247–12256 CrossRef CAS PubMed.
- W. R. Mateker and M. D. McGehee, Adv. Mater., 2017, 29, 1603940 CrossRef PubMed.
- B.-X. Du, Z.-J. Quan, Y.-X. Da, Z. Zhang and X.-C. Wang, Adv. Synth. Catal., 2015, 357, 1270–1276 CrossRef CAS.
- Y. Lin, Y. Bai, Y. Fang, Q. Wang, Y. Deng and J. Huang, ACS Energy Lett., 2017, 2, 1571–1572 CrossRef CAS.
- X. Xiao, J. Dai, Y. Fang, J. Zhao, X. Zheng, S. Tang, P. N. Rudd, X. C. Zeng and J. Huang, ACS Energy Lett., 2018, 3, 684–688 CrossRef CAS.
- G. Dresselhaus, A. F. Kip and C. Kittel, Phys. Rev., 1954, 95, 568–569 CrossRef CAS.
- Y. Zhai, S. Baniya, C. Zhang, J. Li, P. Haney, C.-X. Sheng, E. Ehrenfreund and V. Vardeny Zeev, Sci. Adv., 2017, 3, 1700704 CrossRef PubMed.
- J. Yin, P. Maity, L. Xu, A. M. El-Zohry, H. Li, O. M. Bakr, J.-L. Brédas and O. F. Mohammed, Chem. Mater., 2018, 30, 8538–8545 CrossRef CAS.
- Y. Jun, N. Rounak, M. Partha, G.-A. Luis, A. Dhaifallah, S. R. Iman, B. Jean-Luc, M. B. Osman and F. M. Omar, Nat. Commun., 2021, 12, 3995 CrossRef PubMed.
- N. Li, Q. Wang and H.-L. Zhang, Chem. Rec., 2020, 20, 413–428 CrossRef CAS PubMed.
-
X. Zhai, Y. Huang, Z. Feng, X. Zhang and Q. Wang, in Quantum Dot Optoelectronic Devices, ed. P. Yu and Z. M. Wang, Springer International Publishing, Cham, 2020 DOI:10.1007/978-3-030-35813-6_6.
- V. A. Hintermayr, L. Polavarapu, A. S. Urban and J. Feldmann, ACS Nano, 2018, 12, 10151–10158 CrossRef CAS PubMed.
- Z.-P. Huang, B. Ma, H. Wang, N. Li, R.-T. Liu, Z.-Q. Zhang, X.-D. Zhang, J.-H. Zhao, P.-Z. Zheng, Q. Wang and H.-L. Zhang, J. Phys. Chem. Lett., 2020, 11, 6007–6015 CrossRef CAS PubMed.
- A. Brumberg, B. T. Diroll, G. Nedelcu, M. E. Sykes, Y. Liu, S. M. Harvey, M. R. Wasielewski, M. V. Kovalenko and R. D. Schaller, Nano Lett., 2018, 18, 4771–4776 CrossRef CAS PubMed.
- G. Ghosh, A. Dutta, A. Ghosh, S. Ghosh and A. Patra, J. Phys. Chem. C, 2020, 124, 10252–10260 CrossRef CAS.
- Y. Yang, D. P. Ostrowski, R. M. France, K. Zhu, J. van de Lagemaat, J. M. Luther and M. C. Beard, Nat. Photonics, 2015, 10, 53–59 CrossRef.
- J. Fu, Q. Xu, G. Han, B. Wu, C. H. A. Huan, M. L. Leek and T. C. Sum, Nat. Commun., 2017, 8, 1300 CrossRef PubMed.
- Z.-Y. Zhu, Q.-Q. Yang, L.-F. Gao, L. Zhang, A.-Y. Shi, C.-L. Sun, Q. Wang and H.-L. Zhang, J. Phys. Chem. Lett., 2017, 8, 1610–1614 CrossRef CAS PubMed.
- Y. Li, W. Zhou, Y. Li, W. Huang, Z. Zhang, G. Chen, H. Wang, G.-H. Wu, N. Rolston, R. Vila, W. Chiu and Y. Cui, Joule, 2019, 3, 2854–2866 CrossRef CAS PubMed.
- D. W. deQuilettes, W. Zhang, V. M. Burlakov, D. J. Graham, T. Leijtens, A. Osherov, V. Bulović, H. J. Snaith, D. S. Ginger and S. D. Stranks, Nat. Commun., 2016, 7, 11683–11692 CrossRef CAS PubMed.
- Y. Lu, J. Hu, Y. Ge, B. Tian, Z. Zhang and M. Sui, J. Mater. Chem. A, 2021, 9, 15059–15067 RSC.
- L. Piveteau, V. Morad and M. V. Kovalenko, J. Am. Chem. Soc., 2020, 142, 19413–19437 CrossRef CAS PubMed.
- J. Lee, W. Lee, K. Kang, T. Lee and S. K. Lee, Chem. Mater., 2020, 33, 370–377 CrossRef.
- B. A. Rosales, L. Men, S. D. Cady, M. P. Hanrahan, A. J. Rossini and J. Vela, Chem. Mater., 2016, 28, 6848–6859 CrossRef CAS.
- Z. Fan, H. Xiao, Y. L. Wang, Z. P. Zhao, Z. Y. Lin, H. C. Cheng, S. J. Lee, G. M. Wang, Z. Y. Feng, W. A. Goddard, Y. Huang and X. F. Duan, Joule, 2017, 1, 548–562 CrossRef CAS.
- L. Piveteau, V. Morad and M. V. Kovalenko, J. Am. Chem. Soc., 2020, 142, 19413–19437 CrossRef CAS PubMed.
- J.-C. Blancon, H. Tsai, W. Nie, C. C. Stoumpos, L. Pedesseau, C. Katan, M. Kepenekian, C. M. M. Soe, K. Appavoo, M. Y. Sfeir, S. Tretiak, P. M. Ajayan, M. G. Kanatzidis, J. Even, J. J. Crochet and A. D. Mohite, Science, 2017, 355, 1288–1292 CrossRef CAS PubMed.
- X. Jiang, J. Hoffman, C. C. Stoumpos, M. G. Kanatzidis and E. Harel, ACS Energy Lett., 2019, 4, 1741–1747 CrossRef CAS.
- O. F. Williams, N. Zhou, J. Hu, Z. Ouyang, A. Kumbhar, W. You and A. M. Moran, J. Phys. Chem. A, 2019, 123, 11012–11021 CrossRef CAS PubMed.
- C. Zhao, W. Tian, J. Leng, Y. Zhao and S. Jin, J. Phys. Chem. Lett., 2019, 10, 3950–3954 CrossRef CAS PubMed.
- C. Zhao, W. Tian, Q. Sun, Z. Yin, J. Leng, S. Wang, J. Liu, K. Wu and S. Jin, J. Am. Chem. Soc., 2020, 142, 15091–15097 CrossRef CAS PubMed.
- G. Divitini, S. Cacovich, F. Matteocci, L. Cinà, A. D. Carlo and C. Ducati, Nat. Energy, 2016, 1, 15012 CrossRef CAS.
- L. Wei-Chun, L. Wei-Chun, L. Jun-Xian, W. Yi-Kai, T. Jui-Fu and F. Zi-Yun, npj Mater. Degrad., 2021, 5, 13 CrossRef.
- M. Keisuke, M. Yu, K. Daisuke, I. Masashi, M. Tsutomu, Y. Tomoyuki and H. Kazuyuki, J. Appl. Phys., 2017, 121, 085501 CrossRef.
- H. J. Jung, D. Kim, S. Kim, J. Park, V. P. Dravid and B. Shin, Adv. Mater., 2018, 30, 1802769 CrossRef PubMed.
- J. S. Yoo, G. S. Han, S. Lee, M. C. Kim, M. Choi, H. S. Jung and J.-K. Lee, Nano Res., 2017, 10, 3885–3895 CrossRef CAS.
- D. G. Lee, M.-c. Kim, S. Wang, B. J. Kim, Y. S. Meng and H. S. Jung, ACS Appl. Mater. Interfaces, 2019, 11, 48497–48504 CrossRef CAS PubMed.
- C. Lan, Z. Zhou, R. Wei and J. C. Ho, Mater. Today Energy, 2019, 11, 61–82 CrossRef CAS.
- F. Zhang, H. Lu, J. Tong, J. J. Berry, M. C. Beard and K. Zhu, Energy Environ. Sci., 2020, 13, 1154–1186 RSC.
- Z. K. Tan, R. S. Moghaddam, M. L. Lai, P. Docampo, R. Higler, F. Deschler, M. Price, A. Sadhanala, L. M. Pazos, D. Credgington, F. Hanusch, T. Bein, H. J. Snaith and R. H. Friend, Nat. Nanotechnol., 2014, 9, 687–692 CrossRef CAS PubMed.
- Z. Chu, Q. Ye, Y. Zhao, F. Ma, Z. Yin, X. Zhang and J. You, Adv. Mater., 2021, 33, 2007169 CrossRef CAS PubMed.
- S. Chen and G. Q. Shi, Adv. Mater., 2017, 29, 1605448 CrossRef PubMed.
- B. Zhao, S. Bai, V. Kim, R. Lamboll, R. Shivanna, F. Auras, J. M. Richter, L. Yang, L. Dai, M. Alsari, X.-J. She, L. Liang, J. Zhang, S. Lilliu, P. Gao, H. J. Snaith, J. Wang, N. C. Greenham, R. H. Friend and D. Di, Nat. Photonics, 2018, 12, 783–789 CrossRef CAS.
- C. Sun, Y. Jiang, M. Cui, L. Qiao, J. Wei, Y. Huang, L. Zhang, T. He, S. Li, H.-Y. Hsu, C. Qin, R. Long and M. Yuan, Nat. Commun., 2021, 12, 2207 CrossRef CAS PubMed.
- Y. Ling, Z. Yuan, Y. Tian, X. Wang, J. C. Wang, Y. Xin, K. Hanson, B. Ma and H. Gao, Adv. Mater., 2016, 28, 305–316 CrossRef CAS PubMed.
- Z. Liu, W. Qiu, X. Peng, G. Sun, X. Liu, D. Liu, Z. Li, F. He, C. Shen, Q. Gu, F. Ma, H.-L. Yip, L. Hou, Z. Qi and S.-J. Su, Adv. Mater., 2021, 33, 2103268 CrossRef CAS PubMed.
- X. Xu, H. He, J. Li, Z. Fang, L. Gan, L. Chen and Z. Ye, ACS Appl. Mater. Interfaces, 2019, 11, 8436–8442 CrossRef CAS PubMed.
- J. Y. Jason, S. Gabkyung, R. C. Matthew, P. Tae Gwan, L. Yongli, R. Fabian, K. Young-Ki, M. Chan Su, J. Nam Joong, C.-B. Juan-Pablo, B. Vladimir, S. Seong Sik, G. B. Moungi and S. Jangwon, Nature, 2021, 590, 587–593 CrossRef PubMed.
- H. C. Duyen, C. S. Constantinos, K. F. Omar, T. H. Joseph and G. K. Mercouri, J. Am. Chem. Soc., 2015, 137, 7843–7850 CrossRef PubMed.
- P. Li, Y. Zhang, C. Liang, G. Xing, X. Liu, F. Li, X. Liu, X. Hu, G. Shao and Y. Song, Adv. Mater., 2018, 30, 1805323 CrossRef.
- L. Chao, G. Hao, X. Yingdong, W. Zhuo, L. Xiaotao, X. Junmin, Z. Shouwei, H. Yue, G. Xingyu, H. Wei, C. Lingfeng, N. Tingting, F. Min, L. Hui, D. Han, Y. Hui, C. Shi, R. Xueqin, S. Lin, L. Bixin, Z. Jing, P. Yong, S. Guosheng, W. Jianpu, C. Yonghua, X. Guichuan and H. Wei, Nat. Energy, 2020, 6, 38–45 Search PubMed.
- W. Zhiping, L. Qianqian, P. C. Francis, S. Nobuya, M. H. Laura and J. S. Henry, Nat. Energy, 2017, 2, 17135 CrossRef.
- G. Liu, H. Zheng, X. Xu, S. Xu, X. Zhang, X. Pan and S. Dai, Adv. Funct. Mater., 2019, 29, 1807565 CrossRef CAS.
- Z. Yang, W. Zeyang, H. Shu, Y. Pingyuan, L. Heng and S. ChuanXiang, ACS Appl. Mater. Interfaces, 2021, 13, 12042–12048 CrossRef.
- J. Yeoun-Woo, L. Seungmin, Y. Kyung Mun, J. Kiwan, C. Kwang, C. Mansoo and N. Jun Hong, Nat. Energy, 2021, 6, 63–71 CrossRef.
- Q. Zhang, Q. Shang, R. Su, T. T. H. Do and Q. Xiong, Nano Lett., 2021, 21, 1903–1914 CrossRef CAS PubMed.
- Q. Zhang, S. T. Ha, X. Liu, T. C. Sum and Q. Xiong, Nano Lett., 2014, 14, 5995–6001 CrossRef CAS PubMed.
- H. Zhang, Q. Liao, Y. Wu, Z. Zhang, Q. Gao, P. Liu, M. Li, J. Yao and H. Fu, Adv. Mater., 2018, 30, 1706186 CrossRef PubMed.
- S. Q. Li, D. Y. Lei, W. Ren, X. Y. Guo, S. F. Wu, Y. Zhu, A. L. Rogach, M. Chhowalla and A. K. Y. Jen, Nat. Commun., 2020, 11, 1192 CrossRef CAS PubMed.
- A. A. Balandin, Nat. Mater., 2011, 10, 569–581 CrossRef CAS PubMed.
- M. J. Meziani, W. L. Song, P. Wang, F. Lu, Z. Hou, A. Anderson, H. Maimaiti and Y. P. Sun, Chem. Phys. Chem., 2015, 16, 1339–1346 CrossRef CAS PubMed.
- Z. Fan, H. Xiao, Y. Wang, Z. Zhao, Z. Lin, H.-C. Cheng, S.-J. Lee, G. Wang, Z. Feng, W. A. Goddard, Y. Huang and X. Duan, Joule, 2017, 1, 548–562 CrossRef CAS.
- H. Yu, X. Cheng, Y. Wang, Y. Liu, K. Rong, Z. Li, Y. Wan, W. Gong, K. Watanabe, T. Taniguchi, S. Wang, J. Chen, Y. Ye and L. Dai, ACS Photonics, 2018, 5, 4520–4528 CrossRef CAS.
- H. Yu, X. Xu, H. Liu, Y. Wan, X. Cheng, J. Chen, Y. Ye and L. Dai, ACS Nano, 2020, 14, 552–558 CrossRef CAS PubMed.
- J. Chen, P. Chábera, T. Pascher, M. E. Messing, R. Schaller, S. Canton, K. Zheng and T. Pullerits, J. Phys. Chem. Lett., 2017, 8, 5119–5124 CrossRef CAS PubMed.
- J. Li, S. Zhang, H. Dong, X. Yuan, X. Jiang, J. Wang and L. Zhang, CrystEngComm, 2016, 18, 7945–7949 RSC.
- W. Zhang, L. Peng, J. Liu, A. Tang, J.-S. Hu, J. Yao and Y. S. Zhao, Adv. Mater., 2016, 28, 4040–4046 CrossRef CAS PubMed.
- L. Li, X. Shang, S. Wang, N. Dong, C. Ji, X. Chen, S. Zhao, J. Wang, Z. Sun, M. Hong and J. Luo, J. Am. Chem. Soc., 2018, 140, 6806–6809 CrossRef CAS PubMed.
- Y. T. Li, L. Ding, J. Z. Li, J. Kang, D. H. Li, L. Ren, Z. Y. Ju, M. X. Sun, J. Q. Ma, Y. Tian, G. Y. Gou, D. Xie, H. Tian, Y. Yang, L. W. Wang, L. M. Peng and T. L. Ren, ACS Cent. Sci., 2019, 5, 1857–1865 CrossRef CAS PubMed.
- E. Ercan, J. Y. Chen, C. C. Shih, C. C. Chueh and W. C. Chen, Nanoscale, 2018, 10, 18869–18877 RSC.
- T. Leydecker, M. Herder, E. Pavlica, G. Bratina, S. Hecht, E. Orgiu and P. Samori, Nat. Nanotechnol., 2016, 11, 769–775 CrossRef CAS PubMed.
- M. Y. Liao, Y. C. Chiang, C. H. Chen, W. C. Chen and C. C. Chueh, ACS Appl. Mater. Interfaces, 2020, 12, 36398–36408 CrossRef CAS PubMed.
- J. Wang, J. Li, Q. Tan, L. Li, J. Zhang, J. Zang, P. Tan, J. Zhang and D. Li, J. Phys. Chem. Lett., 2017, 8, 6211–6219 CrossRef CAS PubMed.
- J. Wang, J. Li, S. Lan, C. Fang, H. Shen, Q. Xiong and D. Li, ACS Nano, 2019, 13, 5473–5484 CrossRef CAS PubMed.
- E. S. Shi, B. Yuan, S. B. Shiring, Y. Gao, Akriti, Y. F. Guo, C. Su, M. L. Lai, P. D. Yang, J. Kong, B. M. Savoie, Y. Yu and L. T. Dou, Nature, 2020, 580, 614–620 CrossRef CAS PubMed.
- Y. Chen, Y. Sun, J. Peng, J. Tang, K. Zheng and Z. Liang, Adv. Mater., 2018, 30, 1703487 CrossRef PubMed.
- Y. Wei, G. Feng, P. Mao, Y. Luan, J. Zhuang, N. Chen, H. Yang, W. Li, S. Yang and J. Wang, ACS Appl. Mater. Interfaces, 2020, 12, 8826–8834 CrossRef CAS PubMed.
- R. Dong, C. Lan, F. Li, S. Yip and J. C. Ho, Nanoscale Horiz., 2019, 4, 1342–1352 RSC.
- M. Min, R. F. Hossain, N. Adhikari and A. B. Kaul, ACS Appl. Mater. Interfaces, 2020, 12, 10809–10819 CrossRef CAS PubMed.
- X. Li, D. Yu, J. Chen, Y. Wang, F. Cao, Y. Wei, Y. Wu, L. Wang, Y. Zhu, Z. Sun, J. Ji, Y. Shen, H. Sun and H. Zeng, ACS Nano, 2017, 11, 2015–2023 CrossRef CAS PubMed.
- W. Deng, H. Huang, H. Jin, W. Li, X. Chu, D. Xiong, W. Yan, F. Chun, M. Xie, C. Luo, L. Jin, C. Liu, H. Zhang, W. Deng and W. Yang, Adv. Opt. Mater., 2019, 7, 1801521 CrossRef.
- Y. Shen, D. Yu, X. Wang, C. Huo, Y. Wu, Z. Zhu and H. Zeng, Nanotechnology, 2018, 29, 085201 CrossRef PubMed.
- A. Yang, J. C. Blancon, W. Jiang, H. Zhang, J. Wong, E. Yan, Y. R. Lin, J. Crochet, M. G. Kanatzidis, D. Jariwala, T. Low, A. D. Mohite and H. A. Atwater, Nano Lett., 2019, 19, 4852–4860 CrossRef CAS PubMed.
- L. Zhao, H. Tian, S. H. Silver, A. Kahn, T.-L. Ren and B. P. Rand, Joule, 2018, 2, 2133–2144 CrossRef CAS.
- K.-G. Lim, T.-H. Han and T.-W. Lee, Energy Environ. Sci., 2021, 14, 2009–2035 RSC.
- L. Kai, F. Wei, L. Yanpeng, C. Manish and L. Kian Ping, Nat. Rev. Mater., 2020, 5, 482–500 CrossRef.
- P. A. Franken, A. E. Hill, C. W. Peters and G. Weinreich, Phy. Rev. Lett., 1961, 7, 118–119 CrossRef.
- S. Weili, C. Jun, W. Jinqiang, L. Xiaoming and Z. Haibo, ACS Photonics, 2020, 8, 113–124 Search PubMed.
- X. Zheng, B. Jia, X. Chen and M. Gu, Adv. Mater., 2014, 26, 2699–2703 CrossRef CAS PubMed.
- X. Yin, Z. Ye, D. A. Chenet, Y. Ye, K. O’Brien, J. C. Hone and X. Zhang, Science, 2014, 344, 488–490 CrossRef CAS PubMed.
- Y. Li, Y. Rao, K. F. Mak, Y. You, S. Wang, C. R. Dean and T. F. Heinz, Nano Lett., 2013, 13, 3329–3333 CrossRef CAS PubMed.
- T. Yang, I. Abdelwahab, H. Lin, Y. Bao, S. J. Rong Tan, S. Fraser, K. P. Loh and B. Jia, ACS Photonics, 2018, 5, 4969–4977 CrossRef CAS.
- Q.-Q. Yang, R.-T. Liu, C. Huang, Y.-F. Huang, L.-F. Gao, B. Sun, Z.-P. Huang, L. Zhang, C.-X. Hu, Z.-Q. Zhang, C.-L. Sun, Q. Wang, Y.-L. Tang and H.-L. Zhang, Nanoscale, 2018, 10, 21106–21115 RSC.
- N. Li, Q. Wang and H.-L. Zhang, Chem. Rec., 2020, 20, 413–428 CrossRef CAS PubMed.
- Z.-T. Zhang, Q.-Q. Yang, X.-J. Zhen, Z.-Z. Feng, X.-P. Zhai, X.-D. Zhang, Y.-F. Huang, Q. Wang and H.-L. Zhang, ACS Appl. Mater. Interfaces, 2021, 13, 21626–21634 CrossRef CAS PubMed.
- I. Abdelwahab, G. Grinblat, K. Leng, Y. Li, X. Chi, A. Rusydi, S. A. Maier and K. P. Loh, ACS Nano, 2018, 12, 644–650 CrossRef CAS PubMed.
- C. Yuan, X. Li, S. Semin, Y. Feng, T. Rasing and J. Xu, Nano Lett., 2018, 18, 5411–5417 CrossRef CAS PubMed.
- W.-J. Wei, X.-X. Jiang, L.-Y. Dong, W.-W. Liu, X.-B. Han, Y. Qin, K. Li, W. Li, Z.-S. Lin, X.-H. Bu and P.-X. Lu, J. Am. Chem. Soc., 2019, 141, 9134–9139 CrossRef PubMed.
- X. Han, Y. Zheng, S. Chai, S. Chen and J. Xu, Nanophotonics, 2020, 9, 1787–1810 CrossRef CAS.
- Y. Zhou, Y. Huang, X. Xu, Z. Fan, J. B. Khurgin and Q. Xiong, Appl. Phys. Rev., 2020, 7, 041313 CAS.
- P. Li, Y. Chen, T. Yang, Z. Wang, H. Lin, Y. Xu, L. Li, H. Mu, B. N. Shivananju, Y. Zhang, Q. Zhang, A. Pan, S. Li, D. Tang, B. Jia, H. Zhang and Q. Bao, ACS Appl. Mater. Interfaces, 2017, 9, 12759–12765 CrossRef CAS PubMed.
- S. Hong, F. Lédée, J. Park, S. Song, H. Lee, Y. S. Lee, B. Kim, D.-I. Yeom, E. Deleporte and K. Oh, Laser Photonics Rev., 2018, 12, 1800118 CrossRef.
- A. Mushtaq, D. Kushavah, S. Ghosh and S. K. Pal, Appl. Phys. Lett., 2019, 114, 051902 CrossRef.
- S. J. Varma, J. Cherusseri, J. Li, J. Kumar, E. Barrios and J. Thomas, AIP Adv., 2020, 10, 045130 CrossRef CAS.
- A. Al-Ashouri, E. Köhnen, B. Li, A. Magomedov, H. Hempel, P. Caprioglio, J. A. Márquez, A. B. M. Vilches, E. Kasparavicius, J. A. Smith, N. Phung, D. Menzel, M. Grischek, L. Kegelmann, D. Skroblin, C. Gollwitzer, T. Malinauskas, M. Jošt, G. Matič, B. Rech, R. Schlatmann, M. Topič, L. Korte, A. Abate, B. Stannowski, D. Neher, M. Stolterfoht, T. Unold, V. Getautis and S. Albrecht, Science, 2020, 370, 1300–1309 CrossRef CAS PubMed.
-
M. Yu, M. Ikegami, T. Miyasaka, T. Ohshima and K. Hirose, Evaluation of radiation tolerance of perovskite solar cell for use in space, 2015 IEEE 42nd Photovoltaic Specialist Conference (PVSC), 2015, pp. 1–4 DOI:10.1109/PVSC.2015.7355859.
- Z. Song, C. Li, C. Chen, J. McNatt, W. Yoon, D. Scheiman, P. P. Jenkins, R. J. Ellingson, M. J. Heben and Y. Yan, J. Phys. Chem. C, 2020, 124, 1330–1336 CrossRef CAS.
- Y. Miyazawa, M. Ikegami, H.-W. Chen, T. Ohshima, M. Imaizumi, K. Hirose and T. Miyasaka, iScience, 2018, 2, 148–155 CrossRef CAS PubMed.
- F. Lang, N. H. Nickel, J. Bundesmann, S. Seidel, A. Denker, S. Albrecht, V. V. Brus, J. Rappich, B. Rech, G. Landi and H. C. Neitzert, Adv. Mater., 2016, 28, 8726–8731 CrossRef CAS PubMed.
- S. Yang, Z. Xu, S. Xue, P. Kandlakunta, L. Cao and J. Huang, Adv. Mater., 2019, 31, 1805547 CrossRef PubMed.
- K. Yang, K. Huang, X. Li, S. Zheng, P. Hou, J. Wang, H. Guo, H. Song, B. Li, H. Li, B. Liu, X. Zhong and J. Yang, Org. Electron., 2019, 71, 79–84 CrossRef CAS.
- G. M. Paternò, V. Robbiano, L. Santarelli, A. Zampetti, C. Cazzaniga, V. Garcìa Sakai and F. Cacialli, Sustainable Energy Fuels, 2019, 3, 2561–2566 RSC.
- Y. Tu, J. Wu, G. Xu, X. Yang, R. Cai, Q. Gong, R. Zhu and W. Huang, Adv. Mater., 2021, 33, 2006545 CrossRef CAS PubMed.
- H. Wei, Y. Fang, P. Mulligan, W. Chuirazzi, H.-H. Fang, C. Wang, B. R. Ecker, Y. Gao, M. A. Loi, L. Cao and J. Huang, Nat. Photonics, 2016, 10, 333–339 CrossRef CAS.
- Y. Zhang, R. Sun, X. Ou, K. Fu, Q. Chen, Y. Ding, L.-J. Xu, L. Liu, Y. Han, A. V. Malko, X. Liu, H. Yang, O. M. Bakr, H. Liu and O. F. Mohammed, ACS Nano, 2019, 13, 2520–2525 CrossRef CAS PubMed.
- H. Wei, D. DeSantis, W. Wei, Y. Deng, D. Guo, T. J. Savenije, L. Cao and J. Huang, Nat. Mater., 2017, 16, 826–833 CrossRef CAS PubMed.
- Y. Zhou, J. Chen, O. M. Bakr and O. F. Mohammed, ACS Energy Lett., 2021, 6, 739–768 CrossRef CAS.
- A. Xie, C. Hettiarachchi, F. Maddalena, M. E. Witkowski, M. Makowski, W. Drozdowski, A. Arramel, A. T. S. Wee, S. V. Springham, P. Q. Vuong, H. J. Kim, C. Dujardin, P. Coquet, M. D. Birowosuto and C. Dang, Commun. Mater., 2020, 1, 37 CrossRef.
- B. Jean-Christophe, E. Jacky, C. S. Costas, G. K. Mercouri and D. M. Aditya, Nat. Nanotechnol., 2020, 15, 969–985 CrossRef PubMed.
- R. Paul, C. E. Katherine, D. F. A. Philip, F. Casey, B. W. Malia, M. Aurelio, Z. Matthias, A. F. Sorelle, S. Joshua and J. N. Alexander, Nature, 2016, 533, 73–76 CrossRef PubMed.
- E. Andre, K. Brett, A. N. Roberto, K. Justin, M. S. Susan, M. B. Helen and T. Sebastian, Nature, 2017, 542, 115–118 CrossRef PubMed.
- G.-B. Rafael, A.-I. Jorge, D. H. Timothy, D. David, M. Dougal, A. B.-F. Martin, C. Hyun Sik, E. Markus, H. Dong-Gwang, W. Tony, M. Georgios, J. Soonok, K. Hosuk, M. Hiroshi, N. Masaki, K. Sunghan, H. Wenliang, H. Seong Ik, B. Marc, P. A. Ryan and A.-G. Alán, Nat. Mater., 2016, 15, 1120–1127 CrossRef PubMed.
- X. Fu, B. Chen, J. Tang, M. T. Hassan and A. H. Zewail, Science, 2017, 355, 494–498 CrossRef CAS PubMed.
- G. Cao, S. Sun, Z. Li, H. Tian, H. Yang and J. Li, Sci. Rep., 2015, 5, 8404 CrossRef CAS PubMed.
- J. Ge, Z. Ma, W. Chen, X. Cao, J. Yan, H. Fang, J. Qin, Z. Liu and S. Pan, Nanoscale, 2020, 12, 13558–13566 RSC.
- T. Chang, S.-H. Jo and W. Lu, ACS Nano, 2011, 5, 7669–7676 CrossRef CAS PubMed.
- Z. Liu, H. Yang, J. Wang, Y. Yuan, K. Hills-Kimball, T. Cai, P. Wang, A. Tang and O. Chen, Nano Lett., 2021, 21, 1620–1627 CrossRef CAS PubMed.
- H. Wang, H. Zhang, J. Wang, Y. Gao, F. Fan, K. Wu, X. Zong and C. Li, Angew. Chem., Int. Ed., 2021, 60, 7376–7381 CrossRef CAS PubMed.
- X. Wang, J. He, J. Li, G. Lu, F. Dong, T. Majima and M. Zhu, Appl. Catal., B, 2020, 277, 119230 CrossRef CAS.
- H. Huang, B. Pradhan, J. Hofkens, M. B. J. Roeffaers and J. A. Steele, ACS Energy Lett., 2020, 5, 1107–1123 CrossRef CAS.
- B. Sun, X.-F. Liu, X.-Y. Li, Y. Zhang, X. Shao, D. Yang and H.-L. Zhang, Chem. Mater., 2020, 32, 8914–8920 CrossRef CAS.
- H. Wang, J. Ma and D. Li, J. Phys. Chem. Lett., 2021, 12, 8178–8187 CrossRef CAS PubMed.
- B. Sun, X.-F. Liu, X.-Y. Li, Y. Cao, Z. Yan, L. Fu, N. Tang, Q. Wang, X. Shao, D. Yang and H.-L. Zhang, Angew. Chem., Int. Ed., 2020, 59, 203–208 CrossRef CAS PubMed.
- C. Xu, L. Dongyu, P. Gencai, Z. Donglei, X. Wen, Z. Jinyang, W. He, C. Cong and S. Hongwei, Nanoscale, 2018, 10, 10505–10513 RSC.
- P. Nikolaou, A. Vassilakopoulou, D. Papadatos, E. Topoglidis and I. Koutselas, Mater. Chem. Front., 2018, 2, 730–740 RSC.
- C. Daqin, C. Xiao, L. Xiaoyue, G. Hai, L. Shen and L. Xinyue, Opt. Lett., 2017, 42, 4950 CrossRef PubMed.
- C. Katan, N. Mercier and J. Even, Chem. Rev., 2019, 119, 3140–3192 CrossRef CAS PubMed.
- X.-F. Liu, L. Zou, C. Yang, W. Zhao, X.-Y. Li, B. Sun, C.-X. Hu, Y. Yu, Q. Wang, Q. Zhao and H.-L. Zhang, ACS Appl. Mater. Interfaces, 2020, 12, 43073–43082 CrossRef CAS PubMed.
- J. Peng, C. Q. Xia, Y. Xu, R. Li, L. Cui, J. K. Clegg, L. M. Herz, M. B. Johnston and Q. Lin, Nat. Commun., 2021, 12, 1531 CrossRef CAS PubMed.
- A. Pramanik, S. Patibandla, Y. Gao, K. Gates and P. C. Ray, JACS Au, 2021, 1, 53–65 CrossRef CAS PubMed.
- G. M. Biesold, S. Liang, B. K. Wagner, Z. Kang and Z. Lin, Nanoscale, 2021, 13, 13108–13115 RSC.
|
This journal is © The Royal Society of Chemistry 2022 |
Click here to see how this site uses Cookies. View our privacy policy here.