DOI:
10.1039/D1MA00743B
(Paper)
Mater. Adv., 2022,
3, 312-317
Amphiphilic γ-cyclodextrin–fullerene complexes with photodynamic activity†
Received
20th August 2021
, Accepted 17th October 2021
First published on 18th October 2021
Abstract
Amphiphilic γ-cyclodextrin–fullerene 2
:
1 complexes (CLFCH complexes) were prepared by high-speed vibration milling of lipophilic tail-grafted γ-cyclodextrin (γ-CD), hydrophilic tail-grafted γ-CD and fullerene C60. The transamidation of γ-CD–fullerene complexes having two amino groups with lipophilic and hydrophilic activated esters also afforded amphiphilic CLFCH complexes. Self-assemblies consisting of amphiphilic CLFCH complexes efficiently generated singlet oxygen under photoirradiation. Under visible light irradiation conditions, CLFCH complexes bearing a vitamin E moiety as a lipophilic tail showed high photodynamic activity toward cancer cells.
Introduction
Photodynamic therapy (PDT) is one of the most promising and minimally invasive cancer therapies.1 Photosensitizers which efficiently generate cytotoxic reactive oxygen species (ROS), such as singlet oxygen (1O2) and superoxide radical anions (O2˙−), under light irradiation are suitable as PDT agents.1,2 Fullerenes have been extensively investigated as PDT agents, because the quantum yield of ROS generation from excited fullerenes is very high.3 With the goal of practical use of fullerenes as a PDT agent, hydrophilic substituent-grafted fullerenes and water-soluble fullerene-containing nanocarriers have been explored.3,4 Although functionalized fullerenes having hydrophilic substituents show low IC50 values, they cannot actively accumulate in a tumour site. For the passive accumulation5 of fullerene-based PDT agents in a tumour site, the preparation of fullerene-containing nanocarriers with photodynamic activity has become a research topic of growing interest.4 Ikeda and co-workers have recently reported the synthesis of fullerene-containing liposomes, in which the ROS was efficiently generated from fullerenes localized in the lipophilic segment of the lipid bilayer.4a,6 However, the PDT properties of nanoparticles consisting of fullerene-containing amphiphiles have been less investigated.
γ-Cyclodextrin (γ-CD) is a macrocyclic oligosaccharide that can include fullerene (C60) in its lipophilic cavity leading to a water-soluble complex, the γ-CD–fullerene–γ-CD (CFC) complex, with a bicapped structure (Fig. 1a).7 The synthesis and application of CFC containing functionalized γ-CD derivatives have been barely investigated probably because of their low accessibility.8 We assumed that amphiphilic CFCs consisting of γ-CDs with lipophilic and hydrophilic tails can form PDT-active self-assemblies in water (Fig. 1b). To develop unsymmetric lipophilic γ-CD–fullerene–hydrophilic γ-CD (CLFCH) complexes, we envisioned two approaches, mechanochemical high-speed-vibration milling (HSVM, Method A in Fig. 2)9 and post-complexation functionalization (Method B in Fig. 2). Komatsu, Murata and co-workers reported that the HSVM technique affords symmetrically functionalized CFC complexes efficiently.10 Recently, Hoogenboom and co-workers reported the HSVM-assisted preparation of water-soluble self-assemblies consisting of functionalized γ-CD and C60.11 However, there are no reports of unsymmetrically functionalized CFC complexes synthesized through HSVM of two γ-CD derivatives and C60. In the course of our study, we found that amphiphilic unsymmetric CLFCH complexes can be obtained by HSVM and size-selective filtration. We also found that the conjugation of lipophilic and hydrophilic substituents with the preformed CFC affords amphiphilic unsymmetric CLFCH complexes. We here report the preparation of self-assemblies of amphiphilic CLFCH complexes and their photoinduced cytotoxicity.
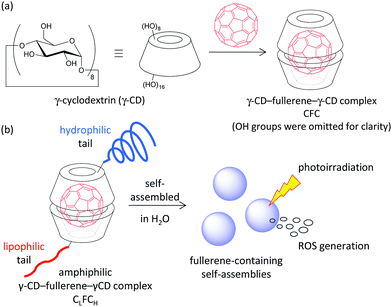 |
| Fig. 1 (a) γ-CD–fullerene–γ-CD complex CFC. (b) Amphiphilic γ-CD–fullerene–γ-CD complex CLFCH (this study). | |
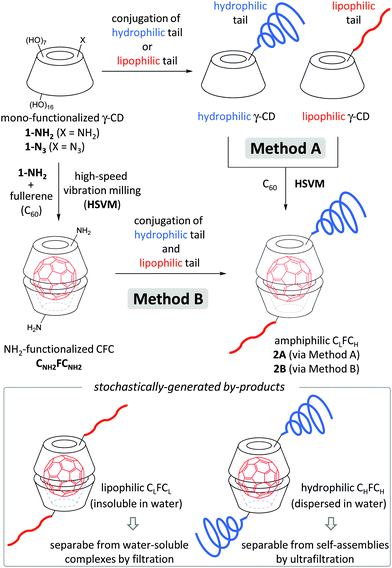 |
| Fig. 2 Preparation of amphiphilic CLFCH through HSVM of C60 with lipophilic and hydrophilic γ-CDs [Method A] and functionalization of amino-functionalized CFC CNH2FCNH2 [Method B]. | |
Results and discussion
Functionalized γ-CD derivatives 1 (Fig. 3) were obtained from accessible 6-monoazido-6-deoxy-γ-cyclodextrin 1-N312 (see the ESI†). The γ-CD derivative 1-P5K having poly(ethylene glycol) (PEG, average molecular weights: 5000) as a hydrophilic tail was synthesized by the copper-mediated [3+2] cyclization reaction. The γ-CD derivatives 1-VE and 1-ICG having a lipophilic tocopherol (vitamin E; VE) and near-infrared indocyanine green (ICG)13 dye were synthesized by transamidation of 6-monoamino-6-deoxy-γ-cyclodextrin 1-NH2.12
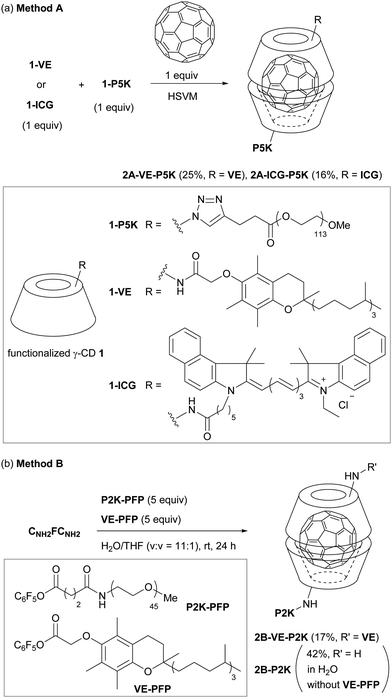 |
| Fig. 3 Synthesis of (a) CLFCH2A through HSVM with mono-functionalized γ-CD derivatives 1 [Method A] and (b) CLFCH2B through functionalization of CNH2FCNH2 [Method B]. PFP: pentafluorophenoxy group. | |
We examined HSVM of C60 with 1-P5K and lipophilic tail-grafted γ-CD derivatives by an in-house built vibrating machine at 3500 rotations per minute (rpm) or a commercially available machine at 1800 rpm (Fig. 3a, Method A). Under HSVM conditions for 30 min, amphiphilic CLFCH complexes 2A were produced. CLFCH complexes 2A could be purified from an aqueous suspension of the crude product using sequential filtration.14 Water-insoluble unreacted C60 and stochastically generated symmetric CLFCL complexes were removed by filtration using a syringe filter (pore size: 0.45 μm). Amphiphilic CLFCH complexes 2A, which form nanometer-sized self-assemblies in water, could be separated from unreacted γ-CDs and water-soluble symmetric CHFCH complexes using ultrafiltration (MWCO: 50 K) as a size-specific separation method. It was confirmed that CLFCH complexes 2A were composed of γ-CDs having lipophilic and hydrophilic tails in a ratio of ca. 1
:
1 in 1H NMR measurement (Fig. 4a and Fig. S1 in the ESI†). In the MALDI-TOF mass spectrum of CLFCH complex 2A-ICG-P5K, the parent signal was detected together with lipophilic and hydrophilic tail-grafted γ-CDs (Fig. 4b and Fig. S2 in the ESI†). In the UV-vis absorption spectra of aqueous solutions of 2A in H2O, a sharp signal at 330–340 nm and a broad signal at 400–700 nm attributed to C60 were observed (Fig. 5). Broadened signals at around 450 nm point out that CLFCH complexes 2A contain non-capsulated C60 like γ-CD–C60 aggregates.15 HSVM of C60 with excess amounts of 1-P5K, followed by sequential filtration, afforded a small amount of water-soluble C60-containing aggregates. The results from this control experiment indicate that the contamination of the γ-CD–C60 aggregate is not avoidable in Method A. Although the contamination was not negligible, it was estimated that molar extinction coefficients (at 332 nm) of 2A-VE-P5K and 2A-ICG-P5K in H2O are 2.9 × 104 and 3.2 × 104 M−1 cm−1, respectively. These extinction coefficient values are similar to the reported values (1.2–4.3 × 104 M−1 cm−1) of CFC complexes and C60-containing liposomes.4a,6,7b In the case of 2A-ICG-P5K, the broad absorption signal of an ICG tail was detected in the near-infrared region. This suggests that lipophilic ICG moieties interact with each other and form stacked aggregates in a lipophilic core.16
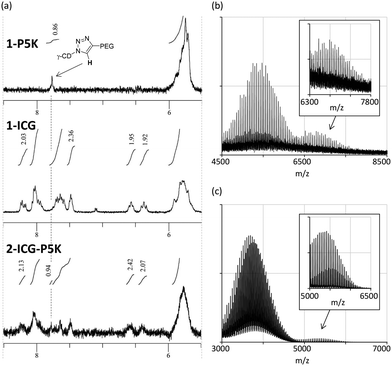 |
| Fig. 4 (a) 1H NMR spectra (d6-DMSO, 25 °C, 400 MHz) of 1-P5K, 1-ICG and 2A-ICG-P5K. MALDI-TOF mass spectra of (b) 2A-ICG-P5K and (c) 2B-VE-P2K. | |
To avoid the contamination of non-capsulated C60, we next examined the functionalization of preformed CFC complex CNH2FCNH2 (Fig. 3, Method B). Among several activated esters, we found that pentafluorophenyl esters VE-PFP and P2K-PFP bearing VE and P2K (PEG, average molecular weights: 2000) are suitable for the functionalization of CNH2FCNH2. In H2O/THF, amphiphilic CLFCH complex 2B-VE-P2K could be synthesized from CNH2FCNH2, P2K-PFP and VE-PFP in a moderate yield. The parent signals of 2B-VE-P2K could be detected by MALDI-TOF mass spectroscopy (Fig. 4c). Under the identical conditions, the treatment of the activated ester bearing P5K moiety did not afford amphiphilic CLFCH complexes, probably because of low reactivity at the long polymer chains. The reaction of CNH2FCNH2 with P2K-PFP afforded PEG-grafted CFC 2B-P2K in moderate yield (Fig. S4 in the ESI†). In UV-vis absorption spectra of 2B, the shoulder signal around 450 nm was not observed (Fig. 5). The molar extinction coefficients of 2B-VE-P2K (2.1 × 104 M−1 cm−1) and 2B-P2K (1.8 × 104 M−1 cm−1) are slightly lower than those of 2A.
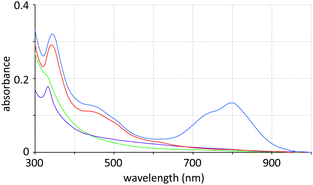 |
| Fig. 5 UV-vis absorption spectra of an aqueous solution of 2A-VE-P5K (red), 2A-ICG-P5K (blue), 2B-VE-P2K (green) and 2B-P2K (purple). Concentration: 1.0 × 10−5 M. | |
The hydrodynamic diameters of self-assemblies of CLFCH complexes determined by dynamic light scattering were 107 ± 28 (2A-VE-P5K), 87 ± 24 (2A-ICG-P5K), 194 ± 44 (2B-VE-P2K), and 322 ± 54 nm (2B-P2K), respectively (Fig. S6 in the ESI†). The diameters of self-assemblies in water and phosphate buffered saline (pH 7.4) were not changed for 10 days. The absorbance of self-assemblies was not decreased under visible light and/or under an oxygen atmosphere for several days.17 This indicates that self-assemblies are stable enough to be handled in both solid and solution states. The particle morphology of CFCs was explored by transmission electron microscopy (TEM). In light of TEM images, spherical self-assemblies of 2A-VE-P5K, 2A-ICG-P5K, and 2B-VE-P2K with average diameters (Da) of 100–200 nm were thought to be multimicellar aggregates (Fig. 6a–c). In the case of 2B-P2K without a lipophilic tail, the formation of vesicles (Da = ∼35 nm) was observed (Fig. 6d).
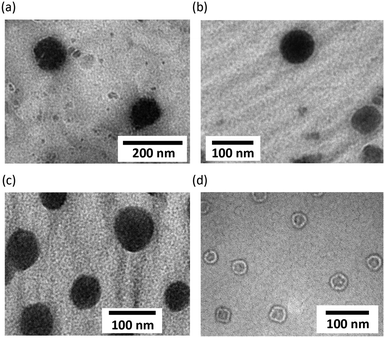 |
| Fig. 6 Selected TEM images of (a) 2A-VE-P5K, (b) 2A-ICG-P5K, (c) 2B-VE-P2K and (d) 2B-P2K. | |
We next examined the generation of ROS from CLFCH complexes under photoirradiation. By monitoring the 1O2-mediated conversion of 9,10-anthracenedipropionic acid (ADPA) to its endoperoxide in UV-vis spectra,6c,18 we observed that the photoenergy transfer from the excited CFC to oxygen molecules took place in all cases to generate reactive 1O2 efficiently (Fig. 7a). The 1O2 generation efficiency of CLFCHs (Abs/Abs0 = 0.43–0.56 after photoirradiation for 10 min) is slightly better than that of the reported C60-containing liposomes (Abs/Abs0 = 0.8 after photoirradiation for 60 min).6,19 From the titration analysis, the effect of O2˙− generation is negligible (Fig. S7 in the ESI†).
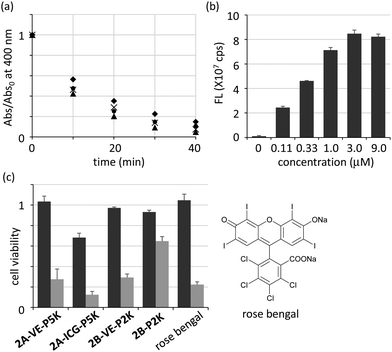 |
| Fig. 7 (a) Time-dependent absorbance (Abs) changes of ADPA at 400 nm with 2A-VE-P2K (circle), 2A-ICG-P2K (diamond), 2B-VE-P2K (triangle) and 2B-P2K (cross) under photoirradiation (17 mW cm−2, λ = 400–800 nm). Abs0: Abs of ADPA before irradiation. Abs and Abs0 are the mean value of two independent experiments. (b) Fluorescence intensities of HeLa cells (1.0 × 103 cells per well) after incubation with 2A-ICG-P5K for 24 h. Error bars indicate s.d. (n = 3). (c) Cell viability of amphiphilic CLFCH complexes and rose bengal (control) after 24 h from the irradiation of visible light (5.1 mW·cm−2, λ = 400–800 nm) for 1 h. Dark grey/pale grey: without/with photoirradiation. Error bars indicate s.d. (n = 3). | |
The uptake of self-assemblies of 2A-ICG-P5K by HeLa cells was examined by utilizing near-infrared fluorescence imaging (Fig. 7b and Fig. S8 in the ESI†). After the treatment of HeLa cells with 2A-ICG-P5K for 24 h, it was confirmed that the fluorescence intensity from HeLa cells was significantly increased as its initial concentration increased. The fluorescence intensities from HeLa cells reached a plateau when its concentration was more than 3 μM, which implies that the intracellular concentration of 2A-ICG-P5K was saturated after incubation for 24 h. Although the localization of the ICG moiety in the cell membrane may induce fluorescence increment, these results suggest that CFC derivatives have an ability to internalize into living cells.
Finally, we investigated the photoinduced cytotoxicity after 24 h from photoirradiation by using HeLa cells cultured with amphiphilic CLFCH complexes in Dulbecco's modified Eagle's medium (Fig. 7c). 75% of cells treated with 2A-VE-P5K died after photoirradiation, while almost all of the cells survived without photoirradiation. 2A-ICG-P5K which has a little inherent toxicity also showed photoinduced cytotoxicity. 2B-VE-P2K, whose structure is similar to 2A-VE-P5K, exhibits similar photoinduced cytotoxicity, indicating that the photodynamic activity of the contaminated non-capsulated C60 is negligible. Vesicles consisting of PEGylated complex 2B-P2K were photodynamically less active than VE-grafted CFCs in cell experiments, probably because of low cellular uptake of large vesicles. Compared with rose bengal20 which is one of the most effective photosensitizers in the visible region, the cytotoxicity of 2A-VE-P5K and 2B-VE-P2K was comparable under the conditions. By considering the advantage of nanoparticles in passive accumulation,5 VE-conjugated CFCs with high photodynamic activity will be a valuable candidate as a PDT drug for cancer.
Conclusions
We succeeded in the preparation of amphiphilic γ-CD–C60 2
:
1 complexes, CLFCHs, having both lipophilic and hydrophilic tails by HSVM and sequential filtration. We also demonstrated the alternative synthesis of amphiphilic CLFCH through the conventional condensation reaction of CFCs having amino groups with activated esters. We demonstrated that self-assemblies consisting of VE-grafted CLFCH complexes showed high photodynamic activity and high photoinduced cytotoxicity. By considering that nanoparticles can accumulate in a tumour site through the enhanced permeability and retention effect,5 nanoparticles consisting of CLFCH complexes are assumed to be one of the promising PDT drugs for malignant cancers. Advanced application in cancer PDT using VE-grafted CLFCH having tumour-targeting molecules is underway.
Experimental section
General
The preparation and characterization of functionalized γ-CDs 1 are summarized in the ESI.† The details of the measurement of hydrodynamic diameters, the detection of singlet oxygen and superoxide radical anions under photoirradiation, the cell uptake of 2A-ICG-P2K, and cytotoxicity evaluation are summarized in the ESI.† UV-vis absorption spectra were recorded using an UV-vis spectrophotometer (V-570, JASCO Co., Japan). Transmission electron microscopy (TEM, JEM-1400, JEOL Ltd, Japan) was used to visualize the morphology of dried self-assemblies. Samples were dropped onto a TEM copper grid covered with a carbon film (200 mesh, Nisshin EM, Japan) and dried for 3 h.
Synthesis of 2A
Throughout the present study, we used an in-house built mill which consisted of a capsule and a milling ball made of stainless steel (Fe–Cr–Ni with a composition of 74
:
18
:
8 by weight). The capsule containing the milling ball was fixed in a vibrating machine so that the capsule could be shaken along its long axis horizontally at a rate of 3500 cycles min−1 (rotations per minute (rpm)). Alternatively, HSVM utilizing a commercial apparatus, mixer mill MM400 (Retsch, Germany, 1800 rpm), with a stainless grinding jar and a mixing ball also afforded the corresponding CFCs. UV-vis absorbance measurement suggests that the crude product is a mixture of the CLFCH complex (∼20%), the CHFCH complex (<10%), and water-insoluble materials containing the CLFCL complex and unreacted fullerene (50–60%). The signal of fullerene was not clearly detected in 13C NMR measurement. X-ray photoelectron spectroscopy (XPS) analysis suggested the existence of C
C bonds (Fig. S5 in the ESI†).
A typical procedure is as follows: fullerene C60 (2.5 mg, 3.5 μmol), 1-P5K (22 mg, 3.5 μmol) and 1-ICG (6.6 mg, 3.5 μmol) were weighed into a stainless capsule together with a mixing ball. The materials were thoroughly mixed by the HSVM technique for 30 min. The mixture was suspended in ca. 2 mL water, and the resulting suspension was filtered through a syringe filter (pore size: 0.45 μm, PVDF) to give a clear green solution. After the ultrafiltration of the resulting green solution with a membrane filter (VIVASPIN 20, MWCO: 50 K, PES, Sartorius Stedim Biotech (Germany)), followed by lyophilization of the residue, CFC 2A-ICG-P5K (5.0 mg, 0.56 μmol, 16% yield, MW(theor) = 8.9 K) was obtained as a green solid. The yield was calculated by using the theoretical molecular weight of CLFCH2A-ICG-P5K. 2A-ICG-P5K: IR (KBr) 527, 666, 720, 843, 926, 963, 1009, 1031, 1061, 1109, 1149, 1242, 1281, 1343, 1360, 1421, 1467, 1655, 2886, 3400 cm−1. 1H NMR (400 MHz, DMSO-d6, 25 °C) δ 1.30–1.75 (m, 10H), 1.91 (br s, 12H), 3.21–3.62 (overlapped with HDO, m, 11383H), 4.09–4.28 (m, 4H), 4.35–4.55 (m, 48H), 4.86–4.89 (m, 16H), 5.70–5.98 (m, 32H), 6.38–6.42 (m, 2H), 6.52–6.58 (m, 2H), 7.45–7.71 (m, 9H), 7.81–8.27 (m, 8H).
CLFCH2A-VE-P5K was similarly prepared. 2A-VE-P5K (a brown solid, 7.7 mg, 0.87 μmol, 25% yield, MW(theor) = 8.8 K): IR (KBr) 527, 709, 760, 744, 942, 1003, 1029, 1084, 1107, 1154, 1252, 1343, 1416, 1458, 1542, 1561, 1655, 2875, 2895, 2926, 3369 cm−1. 1H NMR (400 MHz, DMSO-d6, 25 °C) δ 0.82 (br s, 12H), 1.07–1.52 (m, 28H), 1.97 (s, 3H), 2.04 (s, 3H), 2.07 (s, 3H), 3.18–3.62 (overlapped with HDO, m, 1206H), 3.97–4.11 (m, 4H), 4.35–4.60 (m, 24H), 4.38–4.58 (m, 8H), 5.70–6.01 (m, 16H), 7.74 (s, 2H).
Synthesis of 2B-VE-P2K
To a solution of CNH2FCNH2 (16 mg, 3.7 μmol) in water (5 mL) were added VE-PFP (12 mg, 19 μmol) in THF (0.5 mL) and P2K-PFP (41 mg, 19 μmol) in water (0.5 mL) at room temperature. After stirring for 24 h, the insoluble materials were removed by filtration by using a syringe filter (pore size: 0.45 μm, PVDF). After the ultrafiltration of the resulting brown solution with the membrane filter (VIVASPIN 20, MWCO: 50K, PES, Sartorius Stedim Biotech (Germany)), followed by lyophilization of the residue, CLFCH complex 2B-VE-P2K (3.8 mg, 0.63 μmol, 17% yield, containing ∼10% of the inseparable γ-CD derivative conjugated with P2K) was obtained as a brown solid. IR (ATR) 604, 720, 730, 898, 1207, 989, 1006, 1100, 1027, 1134, 1474, 1520, 1654, 1777, 2850, 2917, 3365 cm−1. 1H NMsR (400 MHz, DMSO-d6, 25 °C) δ 0.80–0.89 (m, 12H), 1.05–1.95 (m, 33H), 2.02–2.20 (m, 6H), 2.90–3.90 (overlapped with HDO, m, 15001H), 4.51–4.58 (m, 16H), 4.85–5.01 (m, 16H), 5.75–6.05 (m, 32H), 7.97 (s, 1H). The signal of fullerene was not clearly detected in 13C NMR measurement. XPS analysis suggested the existence of C
C bonds (Fig. S5 in the ESI†).
Synthesis of 2B-P2K
A solution of CNH2FCNH2 (14 mg, 2.1 μmol) and P2K-PFP (24 mg, 11 μmol) in water (2 mL) was stirred at room temperature for 24 h. After the ultrafiltration of the reaction mixture with a membrane filter (MWCO: 50K), followed by lyophilization of the residue, CFC 2B-P2K (4.7 mg, 0.88 μmol, 42% yield, MW(theor) = 5.3 K) was obtained as a pale brown solid. IR (ATR) 578, 843, 940, 1024, 1078, 1106, 1343, 1641, 3338 cm−1. 1H NMR (400 MHz, D2O, 25 °C) δ 3.21–3.85 (m, 17169H), 4.44–4.60 (m, 16H), 4.82–4.91 (16H), 5.75–6.05 (m, 32H). The signal of fullerene was not clearly detected in 13C NMR measurement. XPS analysis suggested the existence of C
C bonds (Fig. S5 in the ESI†).
Author contributions
KM, ZDZ, KKa, YK and YM synthesized CFC complexes. KM, ZDZ, KKa, YK, KKo and AE evaluated the optical properties and ROS generation. Cell experiments were conducted by KM, KKa, MO and HH. TEM observation was done by KM and KN. KO directed the project. The manuscript was written by KM and KO. All authors discussed and commented on the manuscript.
Conflicts of interest
There are no conflicts to declare.
Acknowledgements
This work was supported by JSPS KAKENHI Grant Numbers 26708024, 15H00739, 15H00939, and 18H04404, and the Research program for Precursory Research for Embryonic Science and Technology (PRESTO) from Japan Science and Technology Agency (JST), Japan. A part of this study was conducted through the Joint Usage Program of the Radiation Biology Center, Kyoto University. K. M. thanks the Takeda Science Foundation for financial support. We acknowledged Prof. Jun Terao in the University of Tokyo and Assistant Prof. Susumu Tsuda in Osaka Dental University for their support to prepare γ-CD derivatives, and Prof. Teruyuki Kondo and Associate Prof. Yu Kimura in Kyoto University for TEM measurement. We are indebted to CycloChem Co., Ltd, for generous gifts of γ-CD.
Notes and references
-
(a) X. Zhao, J. Liu, J. Fan, H. Chao and X. Peng, Chem. Soc. Rev., 2021, 50, 4185–4219 RSC;
(b) F. Wei, T. W. Rees, X. Liao, L. Ji and H. Chao, Coord. Chem. Rev., 2021, 432, 213714 CrossRef CAS;
(c) P.-C. Lo, M. S. Rodríduez-Morgade, R. K. Pandey, D. K. P. Ng, T. Torres and F. Dumoulin, Chem. Soc. Rev., 2020, 49, 1041–1056 RSC;
(d) X. Dai, T. Du and K. Han, ACS Biomater. Sci. Eng., 2019, 5, 6342–6354 CrossRef CAS PubMed;
(e) M. Yang, T. Yang and C. Mao, Angew. Chem., Int. Ed., 2019, 58, 14066–14080 CrossRef CAS.
-
(a) Á. Juarranz, P. Jaén, F. Sanz-Rodríguez, J. Cuevas and S. González, Clin. Transl. Oncol., 2008, 10, 148–154 CrossRef;
(b) Y.-Y. Wang, Y.-C. Liu, H. Sun and D.-S. Guo, Coord. Chem. Rev., 2019, 395, 46–62 CrossRef CAS;
(c) V.-N. Nguyen, Y. Yan, J. Zhao and J. Yoon, Acc. Chem. Res., 2021, 54, 207–220 CrossRef CAS PubMed.
-
(a)
M. Wang, Y. Huang, F. F. Sperandio, L. Huang, S. K. Sharma, P. Mroz, M. R. Hamblin, L. Y. Chiang, in Carbon Nanomaterials for Biomedical Applications, ed. M. Zhang, R. R. Naik and L. Dai, Springer, Switzerland, 2016, pp. 145–200 Search PubMed;
(b) M. R. Hamblin, Photochem. Photobiol. Sci., 2018, 17, 1515–1533 CrossRef CAS;
(c) J. W. Arbogast, A. P. Darmanyan, C. S. Foote, Y. Rubin, F. N. Diederich, M. M. Alvarez, S. J. Anz and R. L. Whetten, J. Phys. Chem., 1991, 95, 11 CrossRef CAS.
-
(a) D. Antoku, K. Sugikawa and A. Ikeda, Chem. – Eur. J., 2019, 25, 1854–1865 CrossRef CAS PubMed;
(b) T. Eom, V. Barát, A. Khan and M. C. Stuparu, Chem. Sci., 2021, 12, 4949–4957 RSC;
(c) R. Kawasaki, D. Antoku, R. Ohdake, K. Sugikawa and A. Ikeda, Nanoscale Adv., 2020, 2, 4395–4399 RSC;
(d) A. Y. Rybkin, A. Y. Belik, O. A. Kraevaya, E. A. Khakina, A. V. Xhilenkov, N. S. Goryachev, D. Volyniuk, J. V. Grazulevicius, P. A. Troshin and A. I. Kotelnikov, Dyes Pigm., 2019, 160, 457–466 CrossRef CAS;
(e) A. Narumi, T. Nakazawa, K. Shinohara, H. Kato, Y. Iwaki, H. Okimoto, M. Kikuchi, S. Kawaguchi, S. Hino, A. Ikeda, M. S. A. Shaykoon, X. Shen, Q. Duan, T. Kakuchi, K. Yasuhara, A. Nomoto, Y. Mikata and S. Yano, Chem. Lett., 2019, 1209–1212 CrossRef CAS;
(f) Y. Zhang, H. Zhang, Q. Zou, R. Xing, T. Jiao and X. Yan, J. Mater. Chem. B, 2018, 6, 7335–7342 RSC;
(g) K. Mizuki, S. Matsumoto, T. Honda, K. Maeda, S. Toyama, D. Iohara, F. Hirayama, S. Okazaki, K. Takeshita and T. Hatta, Chem. Pharm. Bull., 2018, 66, 822–825 CrossRef CAS.
- Y. Matsumura and H. Maeda, Cancer Res., 1986, 46, 6387–6392 CAS.
-
(a) D. Antoku, S. Satake, T. Mae, K. Sugikawa, H. Funabashi, A. Kuroda and A. Ikeda, Chem. – Eur. J., 2018, 24, 7335–7339 CrossRef CAS PubMed;
(b) A. Ikeda, T. Mae, M. Ueda, K. Sugikawa, H. Shigeto, H. Funabashi, A. Kuroda and M. Akiyama, Chem. Commun., 2017, 53, 2966–2969 RSC;
(c) A. Ikeda, T. Iizuka, N. Maekubo, K. Nobusawa, K. Sugikawa, K. Koumoto, T. Suzuki, T. Nagasaki and M. Akiyama, Chem. Asian J., 2017, 12, 1069–1074 CrossRef CAS PubMed;
(d) A. Ikeda, Chem. Rec., 2016, 16, 249–260 CrossRef CAS PubMed and references therein.
-
(a) T. Andersson, K. Nilsson, M. Sundhal, G. Westman and O. Wenneström, J. Chem. Soc., Chem. Commun., 1992, 28, 604–606 RSC;
(b) Z.-i. Yoshida, H. Takekuma, S.-i. Takekuma and Y. Matsubara, Angew. Chem., Int. Ed. Engl., 1994, 33, 1597–1599 CrossRef.
-
(a) X. Zhu, S. Xiao, D. Zhou, M. Sollogoub and Y. Zhang, Eur. J. Med. Chem., 2018, 146, 194–205 CrossRef CAS PubMed;
(b) X. Zhu, A. Quaranta, R. V. Bensasson, M. Sollogoub and Y. Zhang, Chem. – Eur. J., 2017, 23, 9462–9466 CrossRef CAS PubMed;
(c) K. Nobusawa, D. Payra and M. Naito, Chem. Commun., 2014, 50, 8339–8342 RSC;
(d) Y. Takeda, T. Nagamachi, K. Nishikori and S. Minakata, Asian J. Org. Chem., 2013, 2, 69–73 CrossRef CAS;
(e) H. M. Wang and G. Wenz, Beilstein J. Org. Chem., 2012, 8, 1644–1651 CrossRef CAS;
(f) C. O. Mellet, J. M. Benito and J. M. G. Fernández, Chem. – Eur. J., 2010, 16, 6728–6742 CrossRef.
-
(a) K. Komatsu, Top. Curr. Chem., 2005, 254, 185–206 CrossRef CAS;
(b) S.-E. Zhu, F. Li and G.-W. Wang, Chem. Soc. Rev., 2013, 42, 7535–7570 RSC;
(c) G.-W. Wang, Chin. J. Chem., 2021, 39, 1797–1803 CrossRef CAS.
- K. Komatsu, K. Fujiwara, Y. Murata and T. Braun, J. Chem. Soc., Perkin Trans. 1, 1999, 2963–2966 RSC.
-
(a) J. F. R. Van Guyse, V. R. de la Rosa, R. Lund, M. De Bruyne, R. De Rycke, S. K. Filippov and R. Hoogenboom, ACS Macro Lett., 2019, 8, 172–176 CrossRef CAS;
(b) J. F. R. Van Guyse, V. R. de la Rosa and R. Hoogenboom, Chem. – Eur. J., 2018, 24, 2758–2766 CrossRef CAS.
- W. Tang and S.-C. Ng, Nat. Protoc., 2008, 3, 691–697 CrossRef CAS.
- B. E. Schaafsma, J. S. D. Mieog, M. Hutteman, J. R. van der Vorst, P. J. K. Kuppen, C. W. G. M. Löwik, J. V. Frangioni, C. J. H. van de Velde and A. L. Vahrmeijer, J. Surg. Oncol., 2011, 104, 323–332 CrossRef CAS.
- Although CLFCHs were supposed
to be a mixture of diastereoisomers related to the position of lipophilic and hydrophilic tails, they could not be assigned by variable-temperature 1H NMR in D2O.
- Because fullerene derivatives and γ-CD can form a water-soluble aggregate, the production of this aggregate could not be excluded.
(a) H. Jiao, S. H. Goh and S. Valiyaveettil, Macromolecules, 2002, 35, 1399–1402 CrossRef CAS;
(b) D. Iohara, F. Hirayama, K. Higashi, K. Yamamoto and K. Uekama, Mol. Pharm., 2011, 8, 1276–1284 CrossRef CAS;
(c) D. Iohara, F. Hirayama, M. Anraku and K. Uekama, ACS Appl. Nano Mater., 2019, 2, 716–725 CrossRef CAS.
-
(a) K. Takechi, P. K. Sudeep and P. V. Kamat, J. Phys. Chem. B, 2006, 110, 16169–16173 CrossRef CAS;
(b) F. Rotermund, R. Weigand and A. Penzkofer, Chem. Phys., 1997, 220, 385–392 CrossRef CAS.
- Because ICG is known to be decomposed by photoirradiation under air, the absorbance of 2A-ICG-P5K was gradually decreased under visible light and oxygen atmosphere. W. Holzer, M. Mauerer, A. Penzkofer, R.-M. Szeimies, C. Abels, M. Landthaler and W. Bäumler, J. Photochem. Photobiol., B, 1998, 47, 155–164 CrossRef CAS.
- B. A. Lindig, M. A. J. Rodgers and A. P. Schaap, J. Am. Chem. Soc., 1980, 102, 5590–5593 CrossRef CAS.
-
(a) A. Ikeda, M. Akiyama, T. Ogawa and T. Takeya, ACS Med. Chem. Lett., 2010, 1, 115–119 CrossRef CAS PubMed;
(b) A. Ikeda, T. Iizuka, N. Maekubo, R. Aono, J.-I. Kikuchi, M. Akiyama, T. Konishi, T. Ogawa, N. Ishida-Kitagawa, H. Tatebe and K. Shiozaki, ACS Med. Chem. Lett., 2013, 4, 752–756 CrossRef CAS PubMed.
-
(a) R. W. Redmond and J. N. Gamlin, Photochem. Photobiol., 1999, 70, 391–475 CrossRef CAS;
(b) E. Gandin, Y. Lion and A. van de Vorst, Photochem. Photobiol., 1983, 37, 271–278 CrossRef CAS.
Footnote |
† Electronic Supplementary Information (ESI) available. See DOI: 10.1039/d1ma00743b |
|
This journal is © The Royal Society of Chemistry 2022 |