DOI:
10.1039/D1LC00807B
(Paper)
Lab Chip, 2022,
22, 148-155
Fabrication of sharp-edged 3D microparticles via folded PDMS microfluidic channels†
Received
8th September 2021
, Accepted 17th November 2021
First published on 18th November 2021
Abstract
3D microparticles have promising applications in self-assembly, biomedical engineering, mechanical engineering, etc. The shape of microparticles plays a significant role in their functionalities. Although numerous investigations have been conducted to tailor the shape of microparticles, the diversity is still limited, and it remains a challenge to fabricate 3D microparticles with sharp edges. Here, we present a facile approach that combines a folded PDMS channel and orthogonal projection lithography for shaping sharp-edged 3D microparticles. By adjusting the number and the length of channel sides, both regular and irregular polyhedral cross-sections of the folded channel can be obtained. UV light with diverse patterns is applied vertically as the second shape controlling factor. A variety of 3D microparticles are obtained with sharp edges, which are potential templates for micromachining tools and abrasives. Some sharp-edged microparticles are assembled into 2D and 3D mesoscale structures, which demonstrates their prospective applications in self-assembly, tissue engineering, etc.
Introduction
Microparticles are widely used in plenty of fields such as self-assembly,1–6 drug delivery,7,8 biological diagnostics,9 tissue engineering,10,11 photonics,12,13 nuclear fuel,14,15etc. Their functionalities are determined by many factors, in which shape is a significant one. For example, a discal carrier for drug delivery has been proved to have longer half-lives in circulation and higher targeting specificity in mice than a spherical carrier.16 Octopus-shaped hydrogel particles have higher cell capture efficiency and more mechanical flexibility than similarly sized discal particles.17 Ellipsoidal particles avoid the coffee-ring effect by achieving a more uniform deposit in an evaporating aqueous solution than mere spherical particles.18 More complex 3D-shaped microparticles are required to be created to meet the emerging needs. Sharp-edged microparticles (SEMPs), an important category of 3D-shaped microparticles, are promising for diverse applications based on their shapes and size ranges. SEMPs with prismatic shapes and tapered shapes can be respectively used for micro-grinding19 and microhole machining.20,21 A SEMP with a single sharp cutting edge can be used for end milling.22 Different sized SEMPs can be applied to process machining parts with different size ranges and precisions.23 As for the self-assembly applications, many investigations have been conducted to study the shapes of SEMPs and the tissue engineering application of SEMP assemblies. SEMPs with different shapes can be assembled to form different tissue constructs (Table S1†), which has a significant impact on current bottom-up tissue engineering approaches. SEMPs with different sizes have influence on the size of the assembly24,25 and the self-assembly efficiency.26 After encapsulating cells, different sized SEMPs can be assembled to form 3D tissue constructs with different distributions of cell densities.27
Although numerous investigations have been conducted to tailor 3D microparticle shapes, the methods for preparing SEMPs with great shape fidelity are still limited. Habasaki and coworkers28 present vertical flow lithography and manage to fabricate SEMPs with shapes like a tetrahedron and a rectangular pyramid. However, the edges of these microparticles are not well defined due to the attenuation and nonuniformity of UV light along the vertical direction, which is mainly caused by a UV absorber. Yuan and coauthors29 use the channel structure and photomask pattern as biaxial control factors to fabricate SEMPs; nevertheless, the resolution of polymerized microshapes along sharp channel features (on the scale: ≤20 μm) is poor. Compressed emulsions are used as the templates for preparing a range of polyhedral microparticles,30 but the shape of the microparticles is spherical, SEMPs with a higher degree of anisotropy are not feasible. Surface tension induced self-folding has also been applied to fabricate SEMPs,31,32 while the shape fidelity of the obtained microparticles needs to be improved. In addition, SEMPs are too large for nanoscale methods, such as colloidal synthesis,2,33 while too small for millimeter-scale methods, such as machining and injection/cast molding.5,34 Microfluidic-based photolithography is a promising method.35–39 Microparticles fabricated using a PDMS microchannel tend to have higher resolution of sharp features when compared with those fabricated using a microchannel made of other materials such as cyclic olefin copolymer (COC), especially when the size scale is below 20 μm.29,36,40 To date, the PDMS microchannel for fabricating 3D microparticles has been usually manufactured based on SU-8 photolithography. The shape of the microchannel's cross-section is mostly restricted to rectangle41–43 or a rectangle-based shape.39,44–47 Due to the high standard of machining precision and great demolding difficulty, it remains challenging to fabricate PDMS microchannels with other cross-section shapes.48 Therefore, there is still an unmet need for a facile, universal, and precise approach to fabricate SEMPs.
Here, we present a novel method that combines the folded PDMS microchannel and orthogonal projection lithography (OPL) to fabricate SEMPs. By adjusting the number and length of channel sides, both regular and irregular polyhedral cross-sections of the folded channel are obtained. Besides the controlling factor of the channel structure, UV light projected from the replaceable mask with a tunable position is also applied to control the shape of the SEMPs. The resultant SEMPs have clear sharp edges and smooth surfaces, which are promising in many applications, such as machining tools and abrasives. Moreover, some of the SEMPs are used as building blocks for the assembly of 2D and 3D mesoscale structures, demonstrating the prospects in the fields of self-assembly, tissue engineering, etc.
Experimental
Fabrication of a microfluidic device
Convex 440C stainless steel metal molds (surface roughness Ra < 0.25 μm) were fabricated through slow-feeding EDM wire-cutting using a wire cut electric discharge digital control machine tool (DK7735, Shenzhen Wanqi CNC Equipment CO., Ltd.), and the machining process is shown schematically in Fig. S1B.† A SYLGARD® 184 silicone elastomer kit (Dow Corning) was used to fabricate a polydimethylsiloxane (PDMS) substrate. A PDMS prepolymer was prepared by mixing the base fluid and the crosslinking oligomers with a ratio of 5
:
1. The prepolymer was degassed first and then poured into the convex metal mold to fabricate the concave PDMS mold. The filled mold was degassed again, and then heated in an oven at 65 °C for 1.5 h. The concave PDMS mold was peeled off from the metal mold after it solidified. It was coated with trichloro(octadecyl)silane (OTS) as the release agent. The PDMS prepolymer was poured into the PDMS mold to fabricate a flat PDMS substrate. The designs of various flat PDMS substrates are shown in Fig. S2.† After being heated at 65 °C for 45 min, the flat PDMS substrate was demolded and then folded in a circle with polycarbonate (PC) clamps pressed on. The cross-section shape of the microchannel was adjusted by tuning the relative positions between the trapezoidal prisms and the PC clamps. After being cured for another 45 min, folded microchannels were obtained.
Materials and reagents
A solution was prepared by mixing poly(ethylene glycol)diacrylate (PEG-DA, Mn = 700 g mol−1, Sigma-Aldrich) and 3 wt% 2-hydroxy-2-methyl-propiophenone (PI, Darocur 1173, Sigma-Aldrich). A small amount of toluidine blue O was added to dye the precursor. Deionized (DI) water was used for washing, storing, and assembling microparticles.
Sharp-edged microparticle (SEMP) fabrication
A Teflon capillary tube was inserted into the folded microchannel to inject the precursor. The microchannel device was mounted on an upright microscope (Leica DM4B, Leica Microsystems CMS GmbH, Germany). Ultraviolet light (UV, 365 nm, 6212P30W WK2, Germany) passes through a ×10 objective to solidify the SEMPs. The exposure time for one single microparticle was set to 400 ms. The mask was designed through AutoCAD 2016 and printed via a high-resolution laser printer (25
400 dpi, Barco SilverWriter860).
Microparticle assembly
Microparticle assembly was conducted on a flat plate with 65% ambient humidity. A needle with a tip diameter of 10 μm was used to move and assemble microparticles under a stereomicroscope (SMZ85T, Weite Photoelectric). A small amount of water was stored beside the microparticles and was used to wet them. Then the assembled structure was air-dried at room temperature for 12 h.
Characterization of the microparticles and their assemblies
The cross-sections of the microchannels were observed through a stereomicroscope (SMZ85T, Weite Photoelectric). The morphologies of the microparticles and their assemblies were observed through a microscope (DM4 B, Leica Microsystems CMS GmbH) and a scanning electron microscope (SEM, FEI Quanta 200).
Software
3D modeling was accomplished with 3ds Max 2017 and PTC Creo Parametric 3.0 M040. Microsoft Excel, in conjunction with Origin 2018, was used to handle data organization, processing, and statistical analysis. ImageJ was used to analyze the photos.
Results and discussion
Folded PDMS microchannel fabrication and OPL
We present a folding method to precisely fabricate microchannels with various cross-section shapes. Taking a triangular microchannel as an example, it can be split and flattened into a substrate featuring three connected trapezoidal prisms, as shown in Fig. 1A. Every inner wall of the microchannel is exposed in an open space. The flat PDMS substrate is fabricated through casting on a concave PDMS mold. The PDMS mold is demolded from the reverse convex metal mold (Fig. 1B). The two-step molding process avoids the rounded features in the PDMS substrate that will lead to wedge-shaped channel corners, which can improve the precision of the microparticle features, as shown in Fig. S1.† The inherent limitations in machining a metal mold with concave features are overcome by replacing the metal mold with convex features. The release agent is applied on the surface of the concave PDMS mold to keep the flat PDMS substrate from bonding with the PDMS mold. After being cured at 65 °C for 45 min, the PDMS substrate is sufficiently strong to be peeled off from the mold without damaging its contact surface. The segmented prisms of the substrate are connected through a thin PDMS layer, the thickness of which influences the ease with which the substrate can be folded and secured in clamps (Fig. S3†). Afterwards, the flat PDMS substrate is folded in a circle. The adjacent angled surfaces of the partly cured PDMS substrate are in contact with each other and are sufficiently reactive for bonding to form a closed channel under appropriate compressional force and heat.49,50 Finally, the obtained triangular microchannel is shown in Fig. 1C, the side length of the cross-section is 320 μm, and every corner is sharp. The same procedure can also be applied for fabricating microchannels with other cross-section shapes, such as square, pentagon, hexagon, and so on. In addition, the inner walls of the folded channel are exposed in an open space, which is also beneficial for selective surface modification (Fig. S4†), enriching the applications of the folded microchannel.
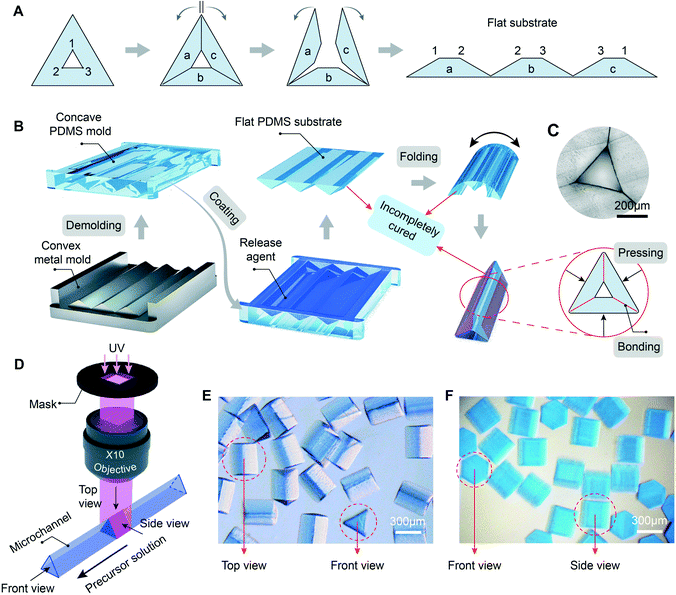 |
| Fig. 1 The fabrication process of the folded PDMS microchannel and the schematics of the orthogonal projection lithography (OPL). A) The triangular microchannel is split into three connected trapezoidal prisms and flattened to form a flat substrate. B) Schematics of the triangular PDMS microchannel fabrication process. C) Optical micrograph of the cross-section of a triangular PDMS microchannel. D) Schematic of the formation of a triangular prism by UV projecting through a square window mask to a triangular channel. E) Optical micrograph of the triangular prism microparticles. F) Optical micrograph of the hexagonal prism microparticles. | |
Stop-flow lithography36,51 is adopted for fabricating 3D microparticles. The photocurable precursor is mainly composed of poly(ethylene glycol)diacrylate (PEG-DA) and a photoinitiator (Darocur 1173). For better observation, toluidine blue O is added to dye the precursor. The precursor fills in the folded microchannel and flows forward intermittently. The UV light projected from the mask passes through the ×10 objective and reaches the microchannel. Once the precursor is exposed to the UV light, the light-initiated chain propagation reaction occurs at the intersection of the UV light and the channel, thus forming a 3D-shaped microparticle as shown in Fig. 1D. The microscopy image in Fig. 1E shows the top view and the front view of the microparticles, the profiles are clear and the corners are sharp. When combining the hexagonal folded microchannel and the mask with a rectangular window, hexagonal prism microparticles are obtained as shown in Fig. 1F. UV light projected from the mask forms a vertical light prism, whose height is in the range of depth of field. The folded microchannel can be regarded as a liquid prism that is projected from the inlet of the precursor. These two prisms intersect with each other orthogonally, producing the intersection-shaped microparticles after photopolymerization.29 Here we call this orthogonal projection lithography (OPL). The sharpness of the SEMP edge can be described by the ratio (R) of the edge-corner radius to the circumradius of the polygonal cross-section. R is mainly affected by two factors, namely the sharpness of the microchannel's edges and the thickness of the uncured inhibition layer (hi). The uncured inhibition layer exists in the interface between the microparticle's surface and the inner wall of the PDMS channel.52,53hi is greatly affected by the exposure energy. By adjusting the exposure time and the UV light intensity, we can reduce hi and obtain microparticles with ideal sharpness (Fig. S5 and S6†). Nowadays, the R of most microtools is below 15%,54–57 which is used as a quality standard in our fabrication process. Here, the R values of the triangular prism microparticles and hexagonal prism microparticles are 3.9% and 9.1%, respectively.
Sharp edge for micro cutting tool applications
SEMPs can be used as templates for fabricating microtools. Regular polygonal folded microchannels such as triangular and hexagonal microchannels can be used to fabricate more diverse 3D SEMPs by means of adjusting the mask shape. When the hexagonal mask window is applied to the triangular channel, the microparticle (SEMP-A) takes the shape of the orthogonal intersection of the hexagonal and triangular prisms as shown in Fig. 2A. When the hexagonal window is applied to the hexagonal channel, the resultant microparticle (SEMP-B) is constructed from two symmetrical frustums of the square pyramid (Fig. 2B). The cutting edges (CEs) of SEMP-A and SEMP-B are determined by the top corners of the triangular and hexagonal channels' cross-sections. The average cutting angles (θ) of the microparticles are based on the angles of the top corners (θd). They are measured to be 59.6° and 119.8°, respectively, as shown in Fig. 2K. The R values of the CEs are respectively measured to be 6.4% and 9.5%, which demonstrates the sharpness of CEs.
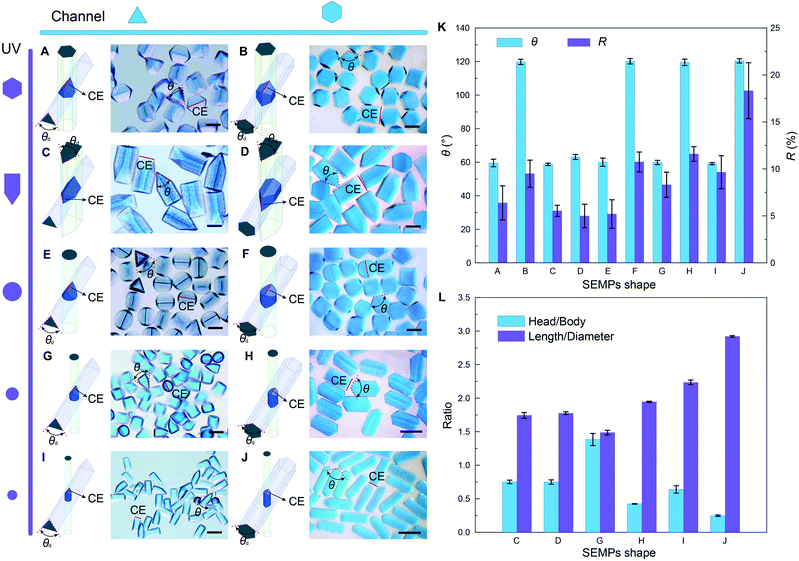 |
| Fig. 2 Sharp-edged microparticles (SEMPs) with different 3D microshapes formed in a regular polygonal folded microchannel through OPL and the data descriptions of the SEMP shapes. Combinations of mask window shapes and microchannel cross-section shapes: A) hexagon to triangle, B) hexagon to hexagon, C) triangle-rectangle to triangle, D) triangle-rectangle to hexagon, E) large circle (diameter ∼310 μm) to triangle, F) large circle (diameter ∼310 μm) to hexagon, G) medium circle (diameter ∼180 μm) to triangle, H) medium circle (diameter ∼180 μm) to hexagon, I) small circle (diameter ∼120 μm) to triangle, and J) small circle (diameter ∼120 μm) to hexagon. K) Histogram describing the obtained SEMPs with the average cutting angles (θ) and the ratios (R) of the edge-corner radius to the circumradius of the cutting edges (CEs). L) Ratio histogram of the SEMPs with different 3D shapes showing the ratio of the particle's cuspidal head to its prismatic body (Rh/b) and the ratio (Rl/d) of the particle's length to its diameter (circumcircle of the cross-section). All scale bars are 300 μm. | |
When the window with a triangle-rectangle-combined shape is applied to the triangular and hexagonal channels, respectively, the obtained microparticles (SEMP-C and SEMP-D) are both two-sectional with cuspidal heads and prismatic bodies (Fig. 2C and D). The cuspidal head can be used as the CE for machining while the prismatic body can be used as a tool shank for clamping.23,58 The CEs of SEMP-C and SEMP-D are both determined by the acute-angle corner of the mask as shown schematically in Fig. 2C and D. Their θ values are measured to be 58.7° and 63.1°, respectively, and their R values are 5.5% and 5.0%. The length ratio of the head and the body (Rh/b) is also determined by the triangle-rectangle-combined shape of the mask window. Rh/b is ∼0.75 for both triangular and hexagonal microchannels in our experiments. The ratio of the length to the diameter of the cross-section circumcircle (Rl/d) is 1.74 and 1.78 for SEMP-C and SEMP-D, respectively.
When a circular window is applied, a cylindrical microparticle with one sharp edge (SEMP-E) flows out from the triangular channel (Fig. 2E), while a cylinder microparticle with two symmetrical sharp edges (SEMP-F) flows out from the hexagonal channel (Fig. 2F). Moreover, by tuning the diameter of the UV light spot (d), SEMPs with different shapes are obtained accordingly. The CEs of this series of SEMPs are determined by the edge corners of the channel cross-sections. For the regular triangular microchannel, Rh/b ≈ d/(l − d) and Rl/d ≈
l/2d, and for the regular hexagonal microchannel, Rh/b ≈ d/( 2
l − d) and Rl/d ≈ 2l/d, where l is the side length of the microchannel cross-section. In our experiments, the side lengths of the triangular channel and the hexagonal channel are 320 μm and 180 μm, respectively. The diameters of the smaller UV light spots are set to 180 μm and 120 μm, respectively. The optical micrographs of the resultant SEMPs are shown in Fig. 2G–J. θ and R of the CEs are measured as shown in Fig. 2K. Fig. 2L shows Rh/b and Rl/d of the SEMPs with cuspidal heads and prismatic bodies, which are consistent with the calculation results. From above we can conclude that the side length of the microchannel cross-section and the diameter of the UV light spot can be used as biaxial control factors to fabricate SEMPs with specific 3D shapes. Moreover, the hydrogel material of these SEMPs can be transformed to hard materials such as ceramics through some specific approaches,51 which demonstrates their great potential as the templates for fabricating micromachining tools.
A family of truncated tetrahedra for self-assembly applications
Besides the regular polygonal shapes, the folded microchannel cross-sections can also be designed with irregular polygonal shapes, such as isosceles triangle, non-equilateral pentagon, and rhombus (Fig. S7†). Combining the shape variation caused by the replaceable mask, the 3D shapes of the SEMPs can be further enriched. A regular tetrahedron, one of the Platonic solids59 with four regular triangular faces, six straight edges, and four vertex corners, is a basic component in a unit cell of cubic crystal systems, such as diamond, and in saturated organic compounds, such as methane.60 A family of truncated tetrahedra, which are obtained by removing a certain edge length from each vertex of a Platonic tetrahedron, is an important subset of 3D SEMPs. There have been extensive studies on simulation of the self-assembly of polyhedral microparticles,3,61,62 and real particles in different scales are of significant importance to explore and verify the complex structure of the assembled materials.1,63 To date, it remains challenging to fabricate SEMPs with the shapes of truncated tetrahedra on the microscale; nevertheless, they can be fabricated by using the folded microchannel and OPL as shown in Fig. 3. The family of truncated tetrahedra is characterized with the truncation t = (a − a1)/a, where a is the edge length of the original Platonic tetrahedron and a1 is the remaining edge length after truncating, e.g., the Platonic tetrahedron with t = 0 in Fig. 3A, the truncated tetrahedron with t = 1/2 in Fig. 3B, the Archimedean truncated tetrahedron with t = 2/3 in Fig. 3C, and the Platonic octahedron with t = 1 in Fig. 3D. These shapes can be defined as the intersections of identical polygonal prism pairs as illustrated in Fig. 3E–G. Irregular polygonal microchannels are used here. The design of each microchannel begins with the design of the lengths and angles of the flat PDMS substrate, as shown in Fig. S2.† Fig. S7† shows the designs of both UV light spot shapes and the relative positions between the microchannels and spots. The SEM images of the resultant microparticles are presented in Fig. 3H–O. Both oblique views (Fig. 3H–K) and top views (Fig. 3L–O) are provided to illustrate the features on different faces. The resultant microparticles on the scale of ∼100 μm have straight sharp edges and smooth surfaces. The size and the shape of the microparticles can be well controlled because of the high resolution of the photomasks and the microchannels. The photomask is printed with 24
000 dpi, which corresponds to a resolution of ∼1 μm. The surface roughness of the inner surfaces of the microchannel is 0.04 μm.
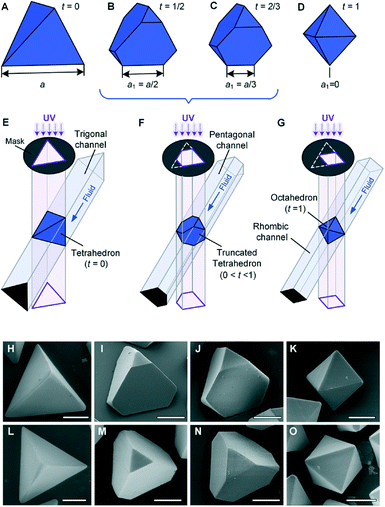 |
| Fig. 3 Fabrication of a family of truncated tetrahedra with 0 ≤ t ≤ 1. Definition of the geometries: A) Platonic tetrahedron with t = 0, B) truncated tetrahedron with t = 1/2, C) Archimedean truncated tetrahedron with t = 2/3, and D) Platonic octahedron with t = 1. Schematics of the orthogonal projection lithography method with polygonal photomasks applied to the UV-polymerized fluid in the polygonal microchannels for the fabrication of E) tetrahedral microparticles, F) truncated tetrahedral microparticles, and G) octahedral microparticles. H–K) SEM images of the oblique views of the resultant microparticles. L–O) SEM images of the corresponding top views of H–K, respectively. All scale bars are 200 μm. | |
Assembly of the truncated tetrahedral 3D microparticles
The throughput rate of the monodisperse SEMPs is 63 particles per min based on OPL. The truncated tetrahedra can be used as building blocks for 2D and 3D assembled structures. A 2D array of the Platonic tetrahedra is shown in Fig. 4A. The Platonic tetrahedron has a highly symmetric geometry,62 and it presents the same status no matter which face lies on the substrate. Therefore, the 2D array of the Platonic tetrahedra results in a uniform distribution of the particles in both shape and height. Such features are desired in coated abrasive products for polishing, grinding, and cutting in the abrasive industry.64,65 The Platonic tetrahedra will be a potential substitute for conventional random-shaped abrasive grains. A 2D-assembled structure of a regular triangle is created with 49 Archimedean truncated tetrahedra (t = 2/3), as shown in Fig. 4B. The assembled triangular plate is almost space-filling, while there are only small tetrahedral voids with an edge length of a/9, which matches well with the simulation results.61,62 In addition, a 3D-assembled Platonic tetrahedron is built with 55 Archimedean truncated tetrahedra, as shown in Fig. 4C and D. An Archimedean truncated tetrahedron has four regular hexagonal faces and four regular triangle faces, and identical Archimedean truncated tetrahedra can be assembled into a defect-free diamond lattice.61,62 The assembled mesoscale tetrahedron indicates that the building blocks are monodisperse. Besides, the mesoscale tetrahedron can also be assembled with binary building blocks: tetrahedral microparticles (a/2 side length) and octahedral microparticles (t = 1). As shown in Fig. 4E and F, the tetrahedral assembly is composed of four tetrahedra on four corners and one octahedron at the centre.
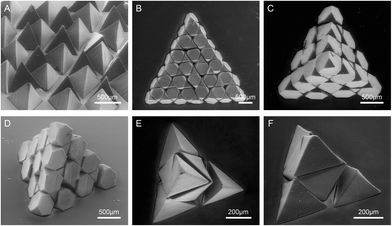 |
| Fig. 4 SEM images of the assembled structures of the truncated tetrahedral 3D microparticles. A) Oblique view of a 2D array of the Platonic tetrahedra. B) Top view of a planar structure of a regular triangle assembled from the Archimedean truncated tetrahedra. C) Top view and D) oblique view of a 3D tetrahedral structure assembled from the Archimedean truncated tetrahedra. E) Top view and F) oblique view of the assembled tetrahedral structure assembled from four tetrahedra (a/2 side length) and one octahedron (t = 1). | |
The SEMPs and their assemblies that we have prepared can be applied to encapsulate cells for tissue engineering, which was rarely reported before due to the difficulties in particle fabrication and sharp feature realization. Compared with the extensively researched hydrogel microparticles with shapes of spheres,66,67 deformed spheres,27,68,69 and 2D-extruded prisms,24,70,71 SEMPs have different remarkable advantages in engineering tissue constructs due to their 3D anisotropic shapes. The tetrahedral microparticles as shown in Fig. 4A exhibit a self-orientation behavior due to their special geometrical features, and the process of direction adjustment is of high efficiency.68 The structures of the 3D-shaped SEMPs and their assemblies enable the cells to distribute in 3D anisotropic spaces. Moreover, the binary assembly as shown in Fig. 4E can be used to co-culture at least two types of cells.27,67 Therefore, the SEMPs and their assemblies with combined outstanding properties have considerable application value in the tissue engineering biological field.
In addition, besides biocompatible materials, this approach can be applied to multiple materials provided that they can be dissolved or dispersed in a UV-curable solution. For example, the resultant microparticles can be used as the templates for ceramic, glass, and other solid particles.35,37,51 The combination of the folded microchannel and OPL is a quite universal approach. Besides the sixteen different shapes that we have successfully fabricated (Table S2†), it can also be applied to another sixteen different shapes listed in Table S3† from ref. 61, including Platonic solid (P03), polygonal prisms (O02 and O04–O07), rhombohedra (O18 and O19), square pyramids (O22 and J01), and the combinations of polygonal prisms and square pyramids (J08, J15, J26, J52, J54, and J55). By tuning the relative positions between the microchannel and UV light in terms of translation and rotation, a greater variety of SEMPs can be obtained. However, we found it very challenging to further reduce the side length of the SEMPs below ∼120 μm (Fig. S8†) due to the size limitation in the folded microchannel's cross-section. The limited machining precision of the wire-cutting equipment and the limited alignment precision of the PDMS substrate folding process are responsible for this size limitation. We adjust the position of the outer wall to tune the position of the microchannel's inner wall. Reducing the thickness of the PDMS substrate enables the inner wall to have a quicker and more precise response to the micromovement of the outer wall, which may be a possible solution to increase the alignment precision. Additionally, the efficiency of the SEMP assembly process can be improved. We may use the concave mold with a shape that is close to the assembly to assist the spatial positioning of the SEMPs. For example, we can use a tetrahedral concave mold to confine the positions of the SEMPs, thus resulting in the assembly of the structures shown in Fig. 4C and E.
Conclusions
In summary, we have developed a facile approach that combines the folded PDMS microchannel and orthogonal projection lithography to fabricate SEMPs, which have been difficult to obtain to date. The shapes of SEMPs are dependent on the cross-section shapes of both the folded microchannel and UV light. The cross-section of the folded microchannel can be processed into regular and irregular shapes by adjusting the number and the length of the channel sides, while the cross-section of the UV light can be tuned through the photomask. The obtained SEMPs are promising templates for micromachining tools and abrasives. In addition, the 2D and 3D assemblies of SEMPs indicate the prospects in self-assembly, tissue engineering, etc.
Author contributions
J. L. and H. C. designed the research; J. L., H. C., and S. L. supervised the work; C. Z. performed the experiments; S. L., Y. L., and J. L. analyzed the data; and C. Z., J. L., and H. C. wrote the paper.
Conflicts of interest
There are no conflicts to declare.
Acknowledgements
This work was supported by the National Natural Science Foundation of China (Grant No. 52025051), the Key Technologies Research and Development Program CN (Grant No. 2018YFE0114900), and the National Major Science and Technology Projects of China CN (Grant No. J2019-VII-0015-0155). The authors thank Ms. Wenyan Yang and Ms. Rong Wang for their help in taking the SEM images and Dr. Jia Man, Mr. Yuzhen Feng for the support with the experiments.
References
- G. M. Whitesides and M. Boncheva, Proc. Natl. Acad. Sci. U. S. A., 2002, 99, 4769–4774 CrossRef CAS PubMed.
- J. Henzie, M. Gruenwald, A. Widmer-Cooper, P. L. Geissler and P. Yang, Nat. Mater., 2012, 11, 131–137 CrossRef CAS PubMed.
- S. Torquato and Y. Jiao, Nature, 2009, 460, 876–879 CrossRef CAS PubMed.
- G. M. Whitesides and B. Grzybowski, Science, 2002, 295, 2418–2421 CrossRef CAS PubMed.
- T. L. Breen, J. Tien, S. R. J. Oliver, T. Hadzic and G. M. Whitesides, Science, 1999, 284, 948–951 CrossRef CAS PubMed.
- L. Cademartiri and K. J. M. Bishop, Nat. Mater., 2015, 14, 2–9 CrossRef CAS PubMed.
- Y. Zhang, H. F. Chan and K. W. Leong, Adv. Drug Delivery Rev., 2013, 65, 104–120 CrossRef CAS PubMed.
- J. A. Champion, Y. K. Katare and S. Mitragotri, J. Controlled Release, 2007, 121, 3–9 CrossRef CAS PubMed.
- S. Link, D. J. Hathcock, B. Nikoobakht and M. A. El-Sayed, Adv. Mater., 2003, 15, 393–396 CrossRef CAS.
- H. Geckil, F. Xu, X. Zhang, S. Moon and U. Demirci, Nanomedicine, 2010, 5, 469–484 CrossRef CAS PubMed.
- A. Khademhosseini, R. Langer, J. Borenstein and J. P. Vacanti, Proc. Natl. Acad. Sci. U. S. A., 2006, 103, 2480–2487 CrossRef CAS PubMed.
- J. Gao, C. Lan, Q. Zhao, B. Li and J. Zhou, Opt. Express, 2018, 26, 13001–13011 CrossRef CAS PubMed.
- X. Liu, Q. Zhao, C. Lan and J. Zhou, Appl. Phys. Lett., 2013, 103, 031910 CrossRef.
- D. P. Stinton, W. J. Lackey and R. D. Spence, J. Am. Ceram. Soc., 1982, 65, 321–324 CrossRef CAS.
- S. Li, J. Bai, S. Cao, X. Yin, C. Tan, P. Li, W. Tian, J. Wang, H. Guo and Z. Qin, Ceram. Int., 2018, 44, 2524–2528 CrossRef CAS.
- S. Muro, C. Garnacho, J. A. Champion, J. Leferovich, C. Gajewski, E. H. Schuchman, S. Mitragotri and V. R. Muzykantov, Mol. Ther., 2008, 16, 1450–1458 CrossRef CAS PubMed.
- L. Chen, H. Z. An, R. Haghgooie, A. T. Shank, J. M. Martel, M. Toner and P. S. Doyle, Small, 2016, 12, 2001–2008 CrossRef CAS PubMed.
- P. J. Yunker, T. Still, M. A. Lohr and A. G. Yodh, Nature, 2011, 476, 308–311 CrossRef CAS PubMed.
- A. Perveen, W. Y. San and M. Rahman, Int. J. Adv. Manuf. Technol., 2011, 61, 101–115 CrossRef.
- L. Zhang, H. Tong and Y. Li, Precis. Eng., 2015, 39, 100–106 CrossRef.
- H. S. Lim, Y. S. Wong, M. Rahman and M. K. Edwin Lee, J. Mater. Process. Technol., 2003, 140, 318–325 CrossRef.
- S. N. B. Oliaei and Y. Karpat, Precis. Eng., 2017, 49, 305–315 CrossRef.
- S. N. B. Oliaei, Y. Karpat, J. Paulo Davim and A. Perveen, J. Manuf. Process., 2018, 36, 496–519 CrossRef.
- Y. Du, E. Lo, S. Ali and A. Khademhosseini, Proc. Natl. Acad. Sci. U. S. A., 2008, 105, 9522–9527 CrossRef CAS PubMed.
- Y. L. Han, Y. Yang, S. Liu, J. Wu, Y. Chen, T. J. Lu and F. Xu, Biofabrication, 2013, 5, 035004 CrossRef CAS PubMed.
- F. Xu, C.-A. M. Wu, V. Rengarajan, T. D. Finley, H. O. Keles, Y. Sung, B. Li, U. A. Gurkan and U. Demirci, Adv. Mater., 2011, 23, 4254–4260 CrossRef CAS PubMed.
- Y. Li, P. Chen, Y. Wang, S. Yan, X. Feng, W. Du, S. A. Koehler, U. Demirci and B.-F. Liu, Adv. Mater., 2016, 28, 3543–3548 CrossRef CAS PubMed.
- S. Habasaki, W. C. Lee, S. Yoshida and S. Takeuchi, Small, 2015, 11, 6391–6396 CrossRef CAS PubMed.
- R. Yuan, M. B. Nagarajan, J. Lee, J. Voldman, P. S. Doyle and Y. Fink, Small, 2018, 14, 8 Search PubMed.
- J. Fan, S.-H. Kim, Z. Chen, S. Zhou, E. Amstad, T. Lin and D. A. Weitz, Small, 2017, 13, 1701256 CrossRef PubMed.
- D. H. Gracias, V. Kavthekar, J. C. Love, K. E. Paul and G. M. Whitesides, Adv. Mater., 2002, 14, 235–238 CrossRef CAS.
- M. Li, Q. Yang, H. Liu, M. Qiu, T. J. Lu and F. Xu, Small, 2016, 12, 4492–4500 CrossRef CAS PubMed.
- Y. Liu, J. Goebl and Y. Yin, Chem. Soc. Rev., 2013, 42, 2610–2653 RSC.
- M. Neudecker, S. Ulrich, S. Herminghaus and M. Schroeter, Phys. Rev. Lett., 2013, 111, 028001 CrossRef PubMed.
- J.-T. Wang, J. Wang and J.-J. Han, Small, 2011, 7, 1728–1754 CrossRef CAS PubMed.
- D. Dendukuri, S. S. Gu, D. C. Pregibon, T. A. Hatton and P. S. Doyle, Lab Chip, 2007, 7, 818–828 RSC.
- R. F. Shepherd, P. Panda, Z. Bao, K. H. Sandhage, T. A. Hatton, J. A. Lewis and P. S. Doyle, Adv. Mater., 2008, 20, 4734–4739 CrossRef CAS.
- N. Hakimi, S. S. H. Tsai, C.-H. Cheng and D. K. Hwang, Adv. Mater., 2014, 26, 1393–1398 CrossRef CAS PubMed.
- K. S. Paulsen, D. Di Carlo and A. J. Chung, Nat. Commun., 2015, 6, 6976 CrossRef PubMed.
- D. Dendukuri, D. C. Pregibon, J. Collins, T. A. Hatton and P. S. Doyle, Nat. Mater., 2006, 5, 365–369 CrossRef CAS PubMed.
- S. Q. Xu, Z. H. Nie, M. Seo, P. Lewis, E. Kumacheva, H. A. Stone, P. Garstecki, D. B. Weibel, I. Gitlin and G. M. Whitesides, Angew. Chem., Int. Ed., 2005, 44, 724–728 CrossRef CAS PubMed.
- B.-U. Moon, S. S. H. Tsai and D. K. Hwang, Microfluid. Nanofluid., 2015, 19, 67–74 CrossRef CAS.
- J. Lee, P. W. Bisso, R. L. Srinivas, J. J. Kim, A. J. Swiston and P. S. Doyle, Nat. Mater., 2014, 13, 524–529 CrossRef CAS PubMed.
- K. S. Paulsen and A. J. Chung, Lab Chip, 2016, 16, 2987–2995 RSC.
- K. S. Paulsen, Y. Deng and A. J. Chung, Adv. Sci., 2018, 5, 1800252 CrossRef PubMed.
- S. A. Lee, S. E. Chung, W. Park, S. H. Lee and S. Kwon, Lab Chip, 2009, 9, 1670–1675 RSC.
- S. E. Chung, W. Park, S. Shin, S. A. Lee and S. Kwon, Nat. Mater., 2008, 7, 581–587 CrossRef CAS PubMed.
- S. M. Nam, K. Kim, I.-S. Kang, W. Park and W. Lee, BioChip J., 2019, 13(3), 226–235 CrossRef CAS.
- Y. Chang and H. You, BioChip J., 2020, 14, 349–357 CrossRef CAS.
- L. Yu and A. L. Skov, Polym. J., 2014, 46, 123–129 CrossRef CAS.
- H. Chen, C. Zhou, Y. Li, S. Liang and J. Li, Sci. Rep., 2020, 10, 17134 CrossRef CAS PubMed.
- C. Decker and A. D. Jenkins, Macromolecules, 1985, 18, 1241–1244 CrossRef CAS.
- D. Dendukuri, D. C. Pregibon, J. Collins, T. A. Hatton and P. S. Doyle, Nat. Mater., 2006, 5, 365–369 CrossRef CAS PubMed.
- A. Perveen, W. Y. San and M. Rahman, Int. J. Adv. Manuf. Technol., 2012, 61, 101–115 CrossRef.
- H. Ohmori, K. Katahira, T. Naruse, Y. Uehara, A. Nakao and M. Mizutani, CIRP Ann. Manuf. Technol., 2007, 56, 569–572 CrossRef.
- Z. Zhang, H. Peng and J. Yan, Int. J. Mach. Tools Manuf., 2013, 65, 99–106 CrossRef.
- X. Lei, B. Shen, L. Cheng, F. Sun and M. Chen, Int. J. Refract. Met. Hard Mater., 2014, 43, 30–41 CrossRef CAS.
- R. Mathew and M. M. Sundaram, J. Mater. Process. Technol., 2012, 212, 1567–1572 CrossRef CAS.
-
D. Sutton, Platonic & Archimedean solids, Bloomsbury, USA, 2002 Search PubMed.
-
A. F. Wells, Structural Inorganic Chemistry, Oxford University Press, 2012 Search PubMed.
- P. F. Damasceno, M. Engel and S. C. Glotzer, Science, 2012, 337, 453–457 CrossRef CAS PubMed.
- P. F. Damasceno, M. Engel and S. C. Glotzer, ACS Nano, 2012, 6, 609–614 CrossRef CAS PubMed.
- M. A. Boles, M. Engel and D. V. Talapin, Chem. Rev., 2016, 116, 11220–11289 CrossRef CAS PubMed.
-
M. J. Jackson and M. P. Hitchiner, High Performance Grinding and Advanced Cutting Tools, Springer Science & Business Media, 2012 Search PubMed.
- W. Ding, B. Linke, Y. Zhu, Z. Li, Y. Fu, H. Su and J. Xu, Chin. J. Aeronaut., 2017, 30, 109–134 CrossRef.
- D. Ngoc-Duy, R. Luo, M. T. A. Christine, W. N. Lin, W.-C. Shih, J. C.-H. Goh and C.-H. Chen, Small, 2017, 13, 1700684 CrossRef PubMed.
- Z. Wu, Y. Zheng, L. Lin, S. Mao, Z. Li and J. M. Lin, Angew. Chem., 2020, 59, 2225–2229 CrossRef CAS PubMed.
- Q. Liu, M. Zhao, S. Mytnyk, B. Klemm, K. Zhang, Y. Wang, D. Yan, E. Mendes and J. H. van Esch, Angew. Chem., 2019, 58, 547–551 CrossRef CAS PubMed.
- J. Sun, D. Wei, Y. Zhu, M. Zhong, Y. Zuo, H. Fan and X. Zhang, Biomaterials, 2014, 35, 4759–4768 CrossRef CAS PubMed.
- F. Yanagawa, H. Kaji, Y.-H. Jang, H. Bae, Y. Du, J. Fukuda, H. Qi and A. Khademhosseini, J. Biomed. Mater. Res., Part A, 2011, 97, 93–102 CrossRef PubMed.
- Y. Du, M. Ghodousi, H. Qi, N. Haas, W. Xiao and A. Khademhosseini, Biotechnol. Bioeng., 2011, 108, 1693–1703 CrossRef CAS PubMed.
Footnote |
† Electronic supplementary information (ESI) available. See DOI: 10.1039/d1lc00807b |
|
This journal is © The Royal Society of Chemistry 2022 |