DOI:
10.1039/D1GC03827C
(Paper)
Green Chem., 2022,
24, 375-383
Selective degradation of hemicellulose into oligosaccharides assisted by ZrOCl2 and their potential application as a tanning agent
Received
16th October 2021
, Accepted 2nd December 2021
First published on 3rd December 2021
Abstract
Selective degradation of hemicellulose into value-added chemicals is promising for sustainable biorefinery, but the industrial application of these chemicals is limited. In this study, selective degradation of hemicellulose in pubescen was achieved with 97.3% conversion, and lower than 15% conversion of cellulose and lignin, via the assistance of ZrOCl2 at 120 °C. Oligosaccharides were predominant products after hemicellulose degradation, and the glycosidic bonds could be easily accessed and broken to obtain smaller molecules after ZrOCl2-assisted disruption of the hydrogen networks among the oligosaccharides. Moreover, ZrOCl2 inhibited the condensation of the dehydrated intermediates with xylo-oligosaccharides, preventing the repolymerization of xylo-oligosaccharides into macromolecules. The reaction liquid containing Zr-oligosaccharides was a complex tanning agent used for chrome-free tanning, where oligosaccharides acted as masking agents. By coordination in advance, the oxygen-rich oligosaccharides could help the penetration of Zr species into the leather matrix for further crosslinking with collagen fibers, thereby enlarging the tanning effect of Zr species and increasing the shrinkage temperature of the tanned leather to 13.8 °C. This work represented a step toward the application of biomass-derived chemicals to the sustainable leather industry.
Introduction
With the increasing depletion of fossil resources and the consequent increase in resource demand, lignocellulose has been considered as the most promising alternative for the production of fuels and chemicals because of its sustainability and abundance.1–4 Lignocellulose is the most important component of plant biomass; it consists of cellulose, hemicellulose, and lignin. Cellulose is made up of D-glucose units connected by β-1, 4-glycosidic bonds; the glucose chains are assembled through a large number of inter- and intra-molecular hydrogen bonds to form a 3D structure. Hemicellulose is a heteroglycan with side chains, which is composed of pentoses and doped with a small number of hexoses. The main component of hemicellulose is xylan, with 1,4-β-D-pyran-type xylose as the main chain, with short, branched side chains composed of acetate and other sugars. Different from the above two components of polysaccharides, lignin is a heteropolymer with aromatic structures and is principally composed of three aromatic alcohol structural units.5–9
Depolymerization of lignocellulose via solvothermal conversion benefits the mass and heat transfer of solid polymers during degradation treatment.10 However, due to the complexity and structural difference of the three components, simultaneous conversion produces a mixture that includes oligomers, monosaccharides, furans, carboxylic acids, and phenols; this conversion results in difficulty in separating the products.11,12 Alternatively, through the selective conversion of one component in lignocellulose, the complexity of raw materials can be minimized from the source to improve the selectivity of liquid products. Unlike cellulose with crystalline structure and lignin with wide degradation temperature, hemicellulose can be degraded at a lower temperature with an intensive range, making it more economical for industrial applications.10 Owing to the structural differences of the three components and the distinguishing characteristic temperature for their degradation, hemicellulose is potentially converted to valuable chemicals with high selectivity.9
The most common and mature technology to selectively convert hemicellulose involves the use of mineral acids and alkali salts.13,14 To avoid equipment corrosion from mineral acid and the separation of the dissolved alkali lignin from alkali treatment, inorganic salts are widely adopted for the catalytic degradation of hemicellulose.15–20 Liu et al. used Al2(SO4)3 as a catalyst to selectively convert hemicellulose from corncob to furfural in a γ-valerolactone/water system, and the furfural yield was approximately 50.2 mol% with 95.5 wt% xylan conversion.21 Li et al. used Fe2(SO4)3 and NaCl to selectively catalyze hemicellulose degradation of corncob, where hemicellulose conversion reached 92.4 wt% with a xylose yield of 22.0 wt%.22 Luo et al. achieved selective conversion of hemicellulose (72.6 wt%) from pubescen by using AlCl3 at 120 °C for 4 h, which yielded 36.1% small molecular products and 63.9% oligosaccharides.11 By controlling the heating parameter, hemicellulose in various lignocellulose could be selectively degraded by inorganic salts. Oligosaccharides were the main products in the hydrolysate, followed by different kinds of small molecules, such as acetic acid, glucose, and xylose. However, further utilization of oligosaccharides was restricted by the separation of oligosaccharides from the concentrated inorganic salt solution.10
In our previous work, 92.5% of hemicellulose conversion was achieved with high selectivity in an AlCl3–H2O system; oligosaccharides were the main products. Afterward, the Al-oligosaccharide complex was used as a tanning agent for leather tanning. Al3+ was the chrome-free tanning agent used to disperse and fix collagen fibers, and oligosaccharides were the masking agents for Al3+ penetration.23 However, the tanning effect of Al salt was limited and did not meet the requirement of leather for commercial use. Besides, the concentrated AlCl3 caused the repolymerization of the degraded intermediates, thereby increasing the molecule weight of oligosaccharides, which was unfavorable for the tanning effect. To strengthen the tanning effect of this Cr-free complex tanning agent, additional Zr salt was used during the leather tanning procedure in our following study, since Zr salt was widely reported with stronger crosslinking ability and better tanning performance,24,25 and the ‘Al–Zr-oligosaccharide’ tanning agent indeed showed a better tanning effect.10
In the present study, pubescen was used as the raw material, owing to its easy propagation, fast-growing rate, high productivity, and easy availability in China. The bifunctional effects of ZrOCl2 was combined and fully utilized. First, ZrOCl2 promoted the selective conversion of hemicellulose from pubescens to oligosaccharides, and the products in the hydrolysate and cellulose-lignin rich residue were fully analyzed. Accordingly, the effect of ZrOCl2 on hemicellulose degradation and the interaction between the intermediate products were discussed. Second, considering the excellent coordination ability of Zr species, the potential application of the prepared Zr-oligosaccharide complex was explored for leather tanning, avoiding additional use of the Zr tanning agent and eliminating separation of the degraded products from the reaction mixture.
Results and discussion
ZrOCl2-dependent degradation of hemicellulose in pubescen
ZrOCl2-assisted hemicellulose degradation was investigated at 120 °C for 60 min (Fig. 1A). Without the use of ZrOCl2, the conversions of the three main components in pubescen were relatively low. Only 42.1% of hemicellulose was converted in distilled water, whereas the conversion of cellulose and lignin were even lower than 10%. When the ZrOCl2 concentration was increased to 100 mM, the conversion of hemicellulose increased dramatically to 90%, and the conversions of cellulose and lignin slightly increased to about 20%. Further increase of the ZrOCl2 concentration to 125 mM showed an insignificant effect on hemicellulose conversion. The high selectivity of hemicellulose conversion could be attributed to two factors. The branched heteroglycan structure of hemicellulose was responsible for the easy degradation of hemicellulose at a mild reaction temperature. However, 120 °C was hardly enough to break the crystalline structure of cellulose connected by intensive hydrogen networks, and the acidic aqueous solution was unfavorable for the dissolution of lignin, which has aromatic features.
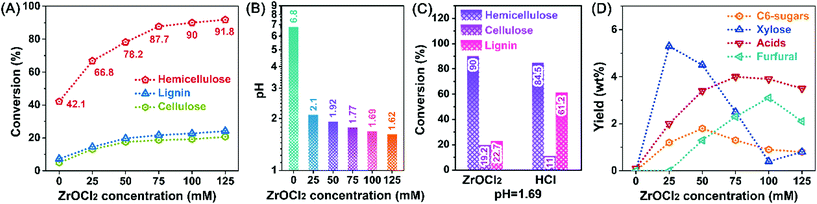 |
| Fig. 1 (A) Conversions of the three main components in pubescen; (B) initial pH values of the ZrOCl2 solutions; (C) conversion comparison of pubescen in ZrOCl2 and HCl solutions; and (D) yields of small molecular products. | |
ZrOCl2 would be partially hydrolyzed in water, generating ZrO(OH)2·xH2O (Lewis acid) and releasing H+ (Brønsted acid).26 Afterward, the ZrOCl2-induced variation of the pH value was measured. A ZrOCl2 dose-dependent decrease of the pH value was clearly observed in Fig. 1B, and only 1.69 of the initial pH value was obtained for a 100 mM ZrOCl2 reaction solution. In comparison, a control experiment for the conversion of pubescen was conducted using HCl solution with the same initial pH value (Fig. 1C). The conversion of hemicellulose in HCl solution was 84.5%, which was slightly lower than that obtained in ZrOCl2 solution. However, the conversion of lignin reached as high as 61.2% in HCl solution, exhibiting inferior selectivity of hemicellulose degradation. This was consistent with the result of our previous work; inorganic salt could inhibit lignin conversion, consequently improving the conversion selectivity of polysaccharides.27
After the selective degradation of hemicellulose in pubescen, the yields of small molecular products were determined (Fig. 1D). The main products from the degradation of pentose-predominant hemicellulose were xylose, acetic acid, and furfural. In addition, some C6-sugars were also obtained from the degradation of cellulose and the side chain of hemicellulose. Even though 42.1% of hemicellulose was degraded in the ZrOCl2-free system, some small molecular products were generated. The use of 25 mM ZrOCl2 solution induced the formation of small molecular products, especially xylose, the yield of which increased from 0.1 wt% to 5.4 wt%. Further increasing the ZrOCl2 concentration led to a rapid decrease in the yield of xylose, whereas the yields of acids and furfural increased first and then decreased. ZrOCl2 promoted the degradation of hemicellulose to oligosaccharides, hydrolysis of monosaccharides, decomposition of acids and furfural, and repolymerization of humin-like compounds. Moreover, the much lower total yields of the small molecular products compared with the converted hemicellulose verified that oligosaccharides were the absolute main products in the hydrolysate.
Variation of the pubescen structure after hemicellulose degradation
After the selective degradation of hemicellulose, the variation of the pubescen structure was measured. Compared with the pubescen raw material, the solid residues turned darker from light brown to brownish black with increasing ZrOCl2 concentration. The SEM images in Fig. 2A show that a wrapped hemicellulose–lignin–cellulose complex with a relatively smooth surface was observed for the raw material. Being treated with the hydrothermal system, the surface of the reaction residues became rougher. With the use of 100 mM ZrOCl2, the bundle structure of cellulose with 20 μm diameters appeared after the hemicellulose component was peeled off. Further increasing the ZrOCl2 concentration to 125 mM caused a slight breakage of the cellulose bundles. To reveal the crystalline structure of cellulose, XRD analysis was used, and the crystalline index (CI) was calculated accordingly (Fig. 2B). The peak positions of the typical crystal planes (101), (002), and (040) of the cellulose type I structure were 16.5°, 22.4°, and 34.6°, respectively.11 These characteristic peaks of crystalline cellulose in the post-treated pubescen became stronger and sharper with increasing ZrOCl2 concentration. In addition, the CI value of cellulose increased from 77.5% to 87.8%, thereby verifying that the crystalline structure became neater. This could be attributed to the dissolution of hemicellulose and amorphous cellulose by ZrOCl2, whereas the crystalline structure of cellulose was well reserved.
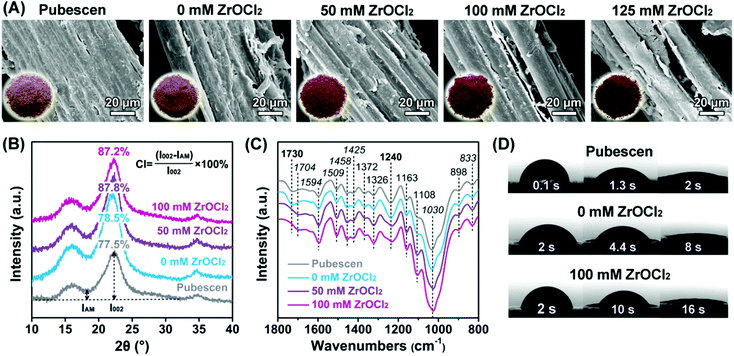 |
| Fig. 2 (A) SEM images, color changes, (B) XRD patterns, (C) FTIR spectra, and (D) contact angle test of pubescen and reaction residues. | |
From FTIR analysis (Fig. 2C), the characteristic peaks of hemicellulose at 1730 and 1240 cm−1 due to the C
O stretching vibration diminished with increasing ZrOCl2 concentration. Meanwhile, the peak intensities of cellulose (1372, 1326, 1163, 1108, and 898 cm−1) and lignin (1704, 1594, 1509, 1458, 1425, 1030, and 833 cm−1) were kept or even strengthened.28–33 This finding confirmed the selective degradation of hemicellulose and the high content of the remaining cellulose and lignin components. The varied contents of the three main components altered the hydrophobicity of the solid products. The contact angle test was conducted (Fig. 2D). The wetting of water droplet to the pubescen raw material was almost completed in about 2 s. The removal of hemicellulose prolonged the wetting time on the solid products, especially for the ZrOCl2-assisted system, in which 16 s was required for the wetting. The enhanced hydrophobicity of the solid products could be ascribed to the decreased content of hydroxyl-rich hemicellulose from 22 wt% to 3.4 wt%, as well as the increased content of aromatic lignin from 24.6 wt% to 29.4 wt%. Moreover, the rough surface of the solid products after hemicellulose removal also contributed to the improved hydrophobicity.
ZrOCl2 effect on the structural features of oligosaccharides
Considering the low yield of the small molecular products from hemicellulose degradation, the molecular weight distribution of the hydrolysate was determined by GPC analysis. As shown in Fig. 3, the weight average molecular weight (Mw) of the hydrolysate in the ZrOCl2-free system was only 339 g mol−1, and 55.3% of the hydrolysate was distributed below 300 g mol−1. The fragments with low molecular weight were possibly generated from the easily degraded hemicellulose and amorphous cellulose. The extensive degradation of hemicellulose by the assistance of ZrOCl2 brought about a higher proportion of oligosaccharides with larger molecular sizes. The molecular weights of the most degraded products ranged from 300 g mol−1 to 500 g mol−1, corresponding to 2 to 4 sugar units, which indicated the widely existed oligosaccharides in the hydrolysate. Furthermore, the over-concentrated ZrOCl2 system slightly reduced the Mw of the oligosaccharides, demonstrating that ZrOCl2 could promote the cleavage of the glycosidic bonds and cut down the oligosaccharides into smaller molecules.
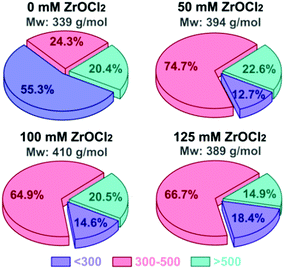 |
| Fig. 3 Molecular weight distribution of the oligosaccharides. | |
Hemicellulose is composed of diverse sugar units connected by glycosidic bonds. Then, the sugar units and the connecting types were characterized by 2D-HSQC NMR spectroscopy (Fig. 4A) to reveal the chemical structure of the oligosaccharides. The chemical shifts of C–H correlation for xylose units appeared at δC/δH 55–95/3.0–4.6 ppm, which could be assigned to the reducing end with the α-xylose unit (X-Rα), reducing end with β-xylose units (X-Rβ), acetic-substituted (X-Ac), and the non-reducing end (X-NR) xylose unit.34,35 Without ZrOCl2, few signals with low intensity could be detected, thereby indicating the insufficient degradation of pubescen. In the presence of ZrOCl2, abundant signals of xylose-based α/β reducing end and non-reducing end could be observed, confirming that xylo-oligosaccharides were the main products from the selective degradation of hemicellulose. Moreover, the signals at 95.0/4.52 ppm (C-1/H-1) and 73.1/4.38 ppm (C-2/H-2) were attributed to acetylated xylo-oligosaccharides, the structure of which was responsible for the formation of acetic acid.35,36 4-O-methyl glucuronic acid (U) connected with C-2 of the β-xylose unit was also detected with weak signal intensity.36,37 Both of the abovementioned structures with the C
O group and xylo-oligosaccharides with the abundant –OH group exerted potential coordination ability with metal ions and implied that the hydrolysate could be used as the masking agent.38,39
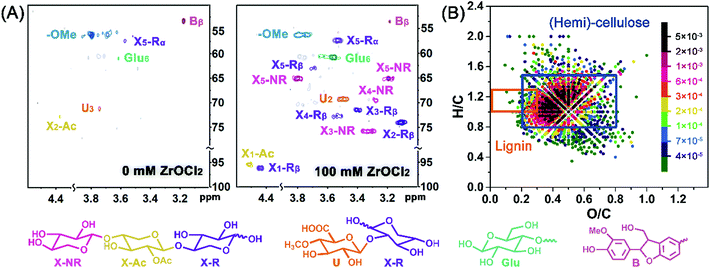 |
| Fig. 4 2D HSQC NMR spectra (A) and the van Krevelen diagram (B) of the liquid products. | |
The signals of gluco-oligomers and α-O-4/β-5 linkages with low intensity were also observed, verifying the slight degradation of cellulose and lignin.40 To reveal the purity of the oligosaccharides in the liquid products, an ultrahigh-resolution mass spectrometer was adopted, and the van Krevelen diagram (O/C vs. H/C ratios of elemental formulas) was analyzed accordingly. One chemical would have certain O/C and H/C ratios, and the same type of chemicals would be classified in a specific area of O/C and H/C ratios.41 For the reaction liquid, the signals with strong intensity were concentrated and distributed in the area standing for hemicellulose and cellulose (O/C 0.2–0.8; H/C 0.8–1.5), whereas scattered signals with low intensity were distributed in the area standing for lignin (O/C 0.0–0.3; H/C 1.0–1.3).42,43 This finding further proved the high purity of the oligosaccharides in the liquid products from ZrOCl2-assisted pubescen conversion.
ZrOCl2 effect on the interactions between the reaction intermediates
To investigate the effect of ZrOCl2 on the variation of the oligosaccharides, ESI-MS (Fig. 5) was conducted to determine the chemical structures. Apparently, various xylo-oligosaccharides and their derivatives, which had different side chains or substituted groups, were identified. Moreover, the dehydration products of xylo-oligosaccharides were obviously observed in the ZrOCl2-free reaction system. For example, the molecular weight of dehydrated xylopentaose (lost one molecule H2O) might have been generated via two ways; one was the dehydration of the end xylose (lost one molecule H2O) on xylopentaose, and the other was the hydrogen bonding interaction between xylotetraose and dehydrated xylose (lost two molecules H2O). However, the signal intensity of these chemicals were weakened in the presence of ZrOCl2. According to the experimental results combined with the literature, the proposed effect of ZrOCl2 is shown in Scheme 1. Xylose formed xylofuranose/xylopyranose via isomerization first and then was dehydrated to yield the two related intermediates (I and II); it was further dehydrated to yield the deep dehydration intermediate (III).40 ZrOCl2 inhibited the condensation or interaction of these intermediates with the xylo-oligosaccharides (Scheme 1A), thereby preventing the repolymerization of xylo-oligosaccharides into macromolecules.
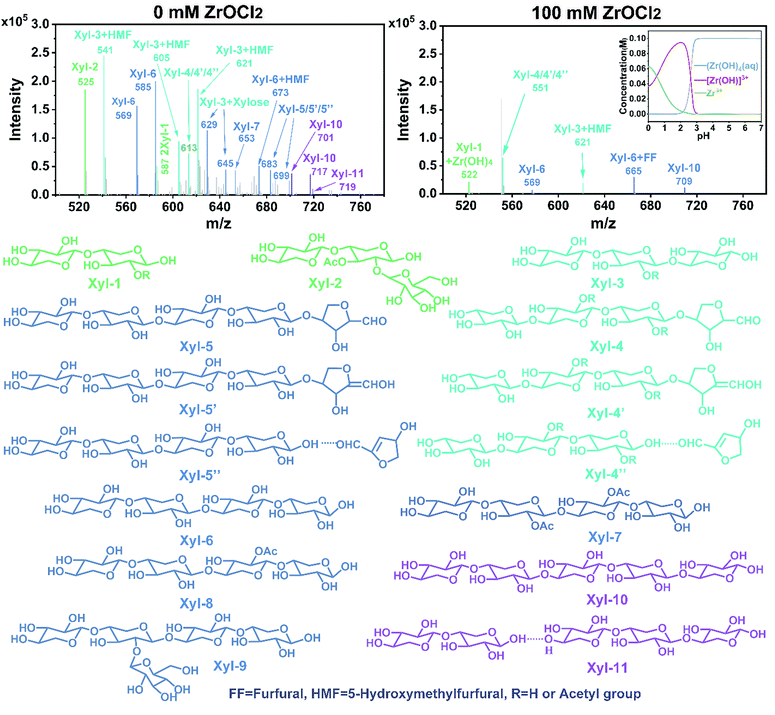 |
| Fig. 5 ESI-MS diagram and the possible structure of detected products. | |
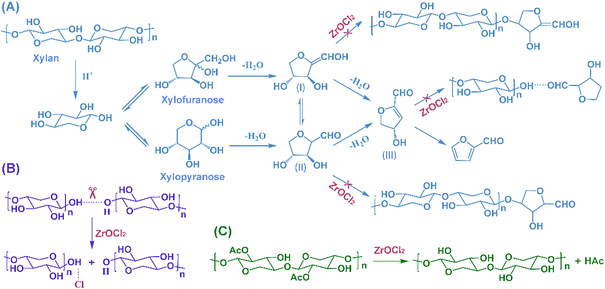 |
| Scheme 1 The mechanism that (A) xylan lost one water molecule or two water molecules, (B) Cl− destroyed the intermolecular hydrogen bonding and form a new hydrogen bond, and (C) ZrOCl2 promoted the abscission of the acetyl group and the formation of acetic acid. | |
The complex of the xylo-oligosaccharides interacted by hydrogen bonding networks could be also determined in the liquid products, especially for the pure water reaction system, whereas the use of ZrOCl2 substantially reduced the related signal intensity. Cl− could break the hydrogen bonds between the xylo-oligosaccharides by forming new hydrogen bonds with them (Scheme 1B), thereby exposing more active sites of the oligosaccharides to be accessed and cleaved for degradation. Compared with the intensive signals of the substituted acetyl xylo-oligosaccharides in the absence of ZrOCl2, the signals were greatly suppressed by ZrOCl2. This finding indicated that ZrOCl2 was conducive for the removal of the substituted acetyl group in xylo-oligosaccharides to form acetic acid (Scheme 1C), which was consistent with the HPLC analysis. However, the generated acid was favorable for the hydrolysis of xylo-oligosaccharides, thereby inducing the autocatalytic degradation of xylo-oligosaccharides.44
Application of the reaction liquid to leather tanning
Before the use of the reaction liquid, the reaction conditions were optimized to achieve the high selectivity of hemicellulose degradation (Fig. 6). The reaction temperature showed the greatest effect on the degradation selectivity of hemicellulose. The higher reaction temperature substantially facilitated the degradation of hemicellulose with loose structure. However, the relatively low-temperature range of 90 °C–130 °C was insufficient for the degradation of stubborn cellulose, because 180 °C was widely reported as the temperature for the softening of crystalline cellulose. The metal chloride solution without organic solvent was unsuitable for lignin degradation, and it even inhibited lignin degradation. Meanwhile, xylose decomposition into acids/furfural and the repolymerization into humins were accelerated at higher reaction temperatures. After optimization, 97.3% of hemicellulose was degraded with lower than 15% conversion of cellulose and lignin in 100 mM ZrOCl2 solution with 6 wt% pubescen feeding at 120 °C for 120 min.
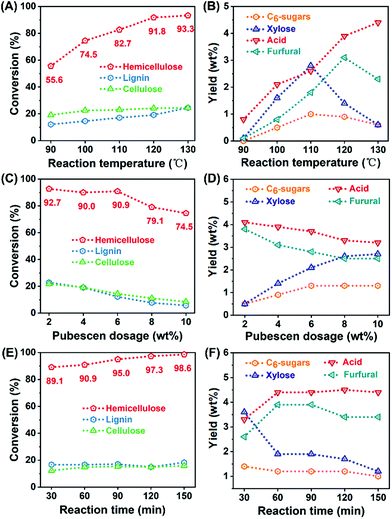 |
| Fig. 6 The effect of (A–B) reaction temperature, (C–D) pubescen dosage and (E–F) reaction time on the conversion of pubescen and the yields of small molecule products. | |
The obtained reaction liquid containing ZrOCl2 and oligosaccharides was used as a Zr-oligosaccharide complex tanning agent for leather tanning (Fig. 7). To measure the tanning effect of the reaction liquid (ii), a control experiment with ZrOCl2 dissolved in distilled water (i) was performed for comparison. The shrinkage temperature (Ts) of the leather tanned by the solution (i) was 61.4 °C, indicating that Zr was not fully penetrated into leather under experimental conditions. On the other hand, the Ts value of the leather tanned by the solution (ii) was as high as 75.2 °C, showing that the Zr-oligosaccharide complex in the reaction liquid exhibited satisfactory tanning effect and consequently endowed the leather with fascinating physicochemical properties. The results of SEM and EDS analyses of the cross section of the leather tanned by solution (i) showed that collagen fibers were compact and stacked with a low dispersion degree, and intensive Zr species were accumulated on the two outer layers. In comparison, a higher dispersion of the collagen fibers together with a uniform distribution of Zr in the grain, middle, and flesh sides of the leather tanned by the solution (ii) indicated the excellent tanning effect of the reaction liquid. Owing to the abundant oxygen-containing groups, such as hydroxyls, acetyls and carboxyls, the oligosaccharides from hemicellulose degradation could coordinate with Zr ions, decreasing the electropositivity of Zr species; the formed ‘Zr-oligosaccharide’ complex firstly penetrated into the skin matrix. Once inside, the Zr species were released from the complex and then reacted with the amino groups of collagen fibers, preventing the overload of Zr species on the leather surface, thereby exerting the tanning effect of Zr to the maximum extent. The oligosaccharides with long molecular chains, widely distributed molecular sizes and abundant oxygen-containing groups, exhibited diverse coordination modes and better molecular flexibility, thereby endowing the tanned leather with relatively satisfactory organoleptic properties, such as softness and smooth surface.24
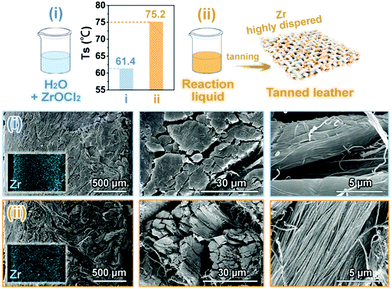 |
| Fig. 7 The SEM and Zr mapping images of tanned leather cross-sections. | |
Conclusions
ZrOCl2-assisted degradation of hemicellulose from pubescen was achieved with high selectivity; 97.3% of hemicellulose was degraded, and the cellulose and lignin degradation rates were only 14.9% and 15% when the process was conducted at 120 °C for 120 min. After removing most of the hemicellulose and amorphous cellulose, cellulose bundles were clearly observed, and the crystalline index of cellulose increased. For liquid products, ZrOCl2 promoted the oligosaccharide degradation to xylose and xylose decomposition to acid/furfural. The analysis of the obtained oligosaccharides showed that ZrOCl2 could promote the cleavage of glycosidic bonds of the oligosaccharides into smaller molecules, whereas ZrOCl2 could also prevent the condensation or interaction between the intermediates and oligosaccharides. Moreover, the hydrogen bonding between the oligosaccharides was efficiently broken by generating new hydrogen bonds of Cl−-oligosaccharides. The 2D HSQC NMR analysis and the van Krevelen diagram confirmed the high purity of the oligosaccharides in the reaction liquid. Finally, the reaction fluid was used as a Zr-oligosaccharide complex tanning agent, in which the oligosaccharides facilitated the penetration of Zr species into the leather matrix for further tanning behavior, and 13.8 °C of shrinkage temperature was increased. These results would benefit the development of a sustainable leather tanning industry from renewable biomass-derived chemicals.
Experimental
Materials
Pubescen samples were purchased from a local company. Before use, the pubescen samples were ground into powder with 80–200 meshes, washed with distilled water five times, and placed in an oven at 105 °C overnight. All the chemical reagents used during the experiments were purchased from Chengdu Kelong Co., Ltd, and Sigma-Aldrich without further treatment.
Selective degradation
The selective degradation of hemicellulose in pubescen was carried out in a 100 mL stainless steel autoclave (Parr) with mechanical stirring. The typical experimental procedure was as follows. First, 50 mL of distilled water, a certain amount of dry pubescen powder, and ZrOCl2 were placed in a reactor. After that, the reactor was heated from room temperature to the target temperature and then held for the designated reaction time. When the reaction was completed, the reactor was fetched out from the furnace and cooled down to room temperature. The reaction mixture was collected. Then, the liquid fraction and solid residue were separated by vacuum filtration. After drying the solid residue in an oven at 105 °C for 24 h, the conversion of pubescen was calculated.
Leather tanning process
Pickled cattle pelt was tailored into matching pieces and weighted. Then, the pelt and a solution (H2O or reaction liquid) were placed into a drum at a weight ratio of 2
:
1, along with a certain amount of ZrOCl2 (equivalent to 2 wt% ZrO2) and 6 wt% NaCl based on the wet weight of cattle pelt. The drum was first rotated at a speed of 19 rpm for 4 h at room temperature for tanning agent penetration. Then, NaHCO3 solution was added every 5 min to adjust the pH of the tanning liquor to around 4. Afterward, the drum was rotated for another 4 h at 40 °C and then left to stand for 12 h to achieve a complete crosslinking reaction. Finally, the obtained leather was washed with running water for 1 min and then dried at room temperature for further characterization.
Characterization of solid samples
The Vanset chemical titration was used to determine the contents of cellulose, hemicellulose and lignin in pubescen, and the specific operations were recorded in the literature.45 The crystal phase structure of cellulose in pubescen before and after the reaction was determined by X-ray diffraction (XRD) on a Rigaku Smartlab instrument. The crystalline index (CI) of cellulose in the sample was calculated using Segal's method.46 Fourier transform infrared spectroscopy (FTIR) was used to record the infrared spectrum of the solid residues with a resolution of 4 cm−1. The surface morphologies of pubescen, reaction residues, and the cross section of the tanned leathers were observed by scanning electron microscopy (SEM, JSM-7500F, JEOL). A contact angle tester was used to evaluate the hydrophobicity changes of pubescen before and after the reaction. The shrinkage temperature (Ts) of the tanned leather was tested using an MSW-YD4 digital shrinkage temperature instrument at a heating rate of 2 °C min−1.
Analysis of liquid products
The small molecular products were quantitatively analyzed by high-performance liquid chromatography (HPLC) equipped with an HPX-87H column (300 mm). H2SO4 solution (5 mM) was used as the mobile phase with a flow rate of 0.6 mL min−1, and the oven temperature was 50 °C. The molecular weight distribution of the oligomers was characterized with a gel permeation chromatography (GPC) analyzer using 0.1 M NaNO3 as flow phase with a flow rate of 50 mL min−1. The 2D HSQC NMR spectra of the liquid products were recorded using a 400 MHz Bruker Avance NMR instrument. For the analysis, 4 mL of the reaction liquid was freeze-dried and redissolved in 0.5 mL of deuterium oxide (D2O). The MS analysis of the reaction liquid was carried out on a 9.4 T Bruker Apex-Ultra FT-ICR mass spectrometer coupled with a negative-ion Apollo II ESI, and the van Krevelen diagram was drawn accordingly. The structure of the liquid products was analyzed by electrospray ionization tandem mass spectrometry (ESI-MS). NaCl solution (1%) was used as the mobile phase with a flow rate of 0.6 mL min−1. The flow rate of dry gas (N2) was 1.5 L min−1, and the spray voltage was 1.58 kV.
Conflicts of interest
There are no conflicts to declare.
Acknowledgements
This work is financially supported by the National Natural Science Foundation of China (22078211). We appreciate Hui Wang from the Analytical & Testing Center of Sichuan University to help with the SEM characterization. We also thank Dr Xiu He at the college of biomass science and engineering, Sichuan University, for experimental assistance.
References
- X. Shen and R. Sun, Carbohydr. Polym., 2021, 261, 117884 CrossRef CAS PubMed.
- J. Li, T. Baker, G. G. Sacripante, D. J. Lawton, H. S. Marway, H. Zhang and M. R. Thompson, Carbohydr. Polym., 2021, 270, 118361 CrossRef CAS PubMed.
- T. Wang, K. Sun, Z. Zhang, L. Zhang, Q. Li, S. Zhang, G. Hu and X. Hu, Green Energy Environ., 2021, 6, 557–566 CrossRef.
- J. Chen, W. Sun, Y. Wang and W. Fang, Green Energy Environ., 2021, 6, 546–556 CrossRef.
- Z. Zhou, D. Liu and X. Zhao, Renewable Sustainable Energy Rev., 2021, 146, 111169 CrossRef CAS.
- Z. Jiang, P. Zhao and C. Hu, Bioresour. Technol., 2018, 256, 466–477 CrossRef CAS PubMed.
- H. Su, Z. Bi, Y. Ni and L. Yan, Green Energy Environ., 2019, 4, 391–399 CrossRef.
- Z. Hui, X. Liu, J. Li, Z. Jiang and C. Hu, ChemSusChem, 2018, 11, 1494–1504 CrossRef PubMed.
- K. Dussan, S. Dooley and R. Monaghan, Chem. Eng. J., 2017, 328, 943–961 CrossRef CAS.
- Z. Jiang, M. Gao, W. Ding, C. Huang, C. Hu, B. Shi and D. C. W. Tsang, J. Hazard. Mater., 2021, 413, 125425 CrossRef CAS PubMed.
- Y. Luo, L. Hu, D. Tong and C. Hu, RSC Adv., 2014, 4, 24194–24206 RSC.
- Q. Xu, X. Hu, L. Zhang, K. Sun, Y. Shao, Z. Gao, Q. Liu and C. Z. Li, Green Energy Environ., 2021, 6, 138–149 CrossRef.
- W. Den, V. K. Sharma, M. Lee, G. Nadadur and R. S. Varma, Front. Chem., 2018, 6, 141 CrossRef PubMed.
- I. Dávila, J. Remón, P. Gullón, J. Labidi and V. Budarin, Bioresour. Technol., 2019, 289, 121726 CrossRef PubMed.
- Y. Luo, Z. Li, Y. Zuo, Z. Su and C. Hu, Sustainable Chem. Eng., 2017, 5, 8137–8147 CrossRef CAS.
- J. Li, Z. Liu, C. Feng, X. Liu, F. Qin, C. Liang, H. Bai, C. Qin and S. Yao, Bioresour. Technol., 2021, 333, 125107 CrossRef CAS PubMed.
- A. Ayoub, R. A. Venditti, J. J. Pawlak, A. Salam and M. A. Hubbe, Sustainable Chem. Eng., 2013, 1, 1102–1109 CrossRef CAS.
- X. Lyu and G. G. Botte, Chem. Eng. J., 2021, 403, 126271 CrossRef CAS.
- X. Luo, Z. Gong, G. Yang, L. Huang, L. Chen and L. Shuai, Chem. Eng. J., 2022, 429, 132365 CrossRef CAS.
- W. Zhang, S. Xu, Y. Xiao, D. Qin, J. Li and C. Hu, Chem. Eng. J., 2021, 421, 130014 CrossRef CAS.
- C. Liu, L. Wei, X. Yin, M. Wei, J. Xu, J. Jiang and K. Wang, Ind. Crops Prod., 2020, 147, 112248 CrossRef CAS.
- D. Li, Y. Sun, R. Li, T. Ao, X. Liu and Y. Luo, Biomass Convers. Biorefin., 2021, 4, 1–10 Search PubMed.
- Z. Jiang, W. Ding, S. Xu, J. Remón, B. Shi, C. Hu and J. H. Clark, Green Chem., 2020, 22, 316–321 RSC.
- Y. Yu, Y. Wang, W. Ding, J. Zhou and B. Shi, Carbohydr. Polym., 2017, 174, 823–829 CrossRef CAS PubMed.
- S. Cao, Y. Zeng, B. Cheng, W. Zhang and B. Liu, J. Am. Leather Chem. Assoc., 2016, 111, 242–249 CAS.
- J. He, Y. Xu, Z. Yu, H. Li, W. Zhao, H. Wu and S. Yang, Sustainable Energy Fuels, 2020, 4, 3102 RSC.
- J. Li, Z. Jiang, L. Hu and C. Hu, ChemSusChem, 2014, 7, 2482–2488 CrossRef CAS PubMed.
- A. M. Olsson and L. Salmén, Carbohydr. Res., 2004, 339, 813–818 CrossRef CAS PubMed.
- D. K. Shen, S. Gu and A. V. Bridgwater, J. Anal. Appl. Pyrolysis, 2009, 87, 199–206 CrossRef.
- S. Soares, N. Ricardo, S. Jones and F. Heatley, Eur. Polym. J., 2001, 37, 737–745 CrossRef CAS.
- Z. Jiang, Y. Ma, X. Guo, J. Remón, D. C. Tsang, C. Hu and B. Shi, J. Hazard. Mater., 2021, 403, 123701 CrossRef CAS PubMed.
- K. Umemura and S. Kawai, J. Appl. Polym. Sci., 2008, 108, 2481–2487 CrossRef CAS.
- D. Tian, F. Shen, J. Hu, M. Huang, L. Zhao, J. He, Q. Li, S. Zhang and F. Shen, Chem. Eng. J., 2022, 428, 131373 CrossRef CAS.
- N. Giummarella and M. Lawoko, ACS Sustainable Chem. Eng., 2017, 5, 5156–5165 CrossRef CAS.
- X. Xiao, J. Wen, Y. Wang, J. Bian, M. Li, F. Peng and R. Sun, Carbohydr. Polym., 2018, 195, 303–310 CrossRef CAS PubMed.
- R. Samuel, M. Foston, N. Jiang, L. Allison and A. J. Ragauskas, Polym. Degrad. Stab., 2011, 96, 2002–2009 CrossRef CAS.
- A. Teleman, J. Lundqvist, F. Tjerneld, H. Stålbrand and O. Dahlman, Carbohydr. Res., 2000, 329, 807–815 CrossRef CAS PubMed.
- R. Zhang and Y. Tian, J. Leather Sci. Eng., 2020, 2, 24 CrossRef.
- Y. Han, J. Hu and G. Sun, J. Leather Sci. Eng., 2021, 3, 4 CrossRef.
- Z. Jiang, Q. Fang, X. Liu, Z. Li, C. Hu and B. Shi, Cellulose, 2020, 27, 1951–1964 CrossRef CAS.
- C. He, Y. Zhang, Y. Li, X. Zhuo and Q. Shi, ACS Omega, 2020, 5, 11730–11736 CrossRef CAS PubMed.
- H. Yan, Z. Li, Z. Wang, Z. Lei, S. Ren, C. Pan, Y. Tian, S. Kang, J. Yan and H. Shui, Fuel, 2019, 246, 394–401 CrossRef CAS.
- Z. Li, Y. Luo, Z. Jiang, Q. Fang and C. Hu, Chin. J. Chem., 2020, 38, 178–184 CrossRef CAS.
- Z. Jiang, V. L. Budarin, J. Fan, J. Remón, T. Li, C. Hu and J. H. Clark, Sustainable Chem. Eng., 2018, 6, 4098–4104 CrossRef CAS.
- L. Hu, Y. Luo, B. Cai, J. Li, D. Tong and C. Hu, Green Chem., 2014, 16, 3107–3116 RSC.
- L. Segal, J. J. Creely, A. E. Martin and C. M. Conrad, Text. Res. J., 1959, 29, 786–794 CrossRef CAS.
|
This journal is © The Royal Society of Chemistry 2022 |