DOI:
10.1039/D2FO02812C
(Paper)
Food Funct., 2023,
14, 427-444
Alginate oligosaccharide ameliorates azithromycin-induced gut microbiota disorder via Bacteroides acidifaciens-FAHFAs and Bacteroides-TCA cycle axes†
Received
22nd September 2022
, Accepted 2nd December 2022
First published on 8th December 2022
Abstract
Alginate oligosaccharide is a kind of prebiotic with broad application prospects. However, little attention is paid to the recovery effect of alginate oligosaccharide on disordered intestinal microecology caused by azithromycin. Therefore, we evaluated the regulatory effect of alginate oligosaccharide and its compound on azithromycin-disturbed gut microbiota in mice via microbiome-metabolomics analysis. The gut microbiota analysis revealed that alginate oligosaccharide and its compound significantly increased the richness and diversity of the gut microbiota which were reduced by azithromycin, with an obvious enrichment of beneficial bacteria such as the Akkermansia genus and Bacteroides acidifaciens, and a remarkable decrease of pathogenic bacteria such as the Staphylococcus genus, which indicated its impact on the gut microbiota dysbiosis. Additionally, the effect of the alginate oligosaccharide compound on regulating the gut microbiota disorder is more significant than that of alginate oligosaccharide. The favorable effects of alginate oligosaccharide were confirmed by beneficial alterations in metabolic effector molecules, which indicated that alginate oligosaccharide and its compound improved metabolic homeostasis via the Bacteroides acidifaciens–fatty acid esters of hydroxy fatty acids (FAHFAs) axis and increasing the levels of the intermediate products of the tricarboxylic acid cycle (TCA cycle), such as citric acid, fumaric acid and α-ketoglutaric acid. Spearman correlation analysis showed that the contents of these three metabolites were also positively related to Bacteroides acidifaciens and Bacteroides sartorii populations, suggesting the potential regulatory role of the Bacteroides genus in energy balance through the TCA cycle. This study may provide an innovative dietary strategy for the regulation of intestinal microecological disorders caused by antibiotics, and reveal the prospect of alginate oligosaccharide as an intestinal microecological regulator.
1. Introduction
Prebiotics are substrates that are not digested and absorbed by the host, but can be decomposed and metabolized by the specific gut microbiota. They have the ability to stimulate the proliferation of specific microbial groups, and inhibit the reproduction of pathogenic bacteria.1–3 Furthermore, they play a positive role in regulating intestinal pH,4 repairing intestinal barriers,5 improving host metabolism6 and regulating immunity,7 thus contributing to host health. Most prebiotics are polysaccharides and oligosaccharides,8 such as alginate oligosaccharide.9 Alginate, a linear binary copolymer of β-D-mannuronate (ManA) and α-L-guluronate (GulA) joined by 1,4-glycoside bonds, widely exists in the cell walls of brown algae such as kelp, giant kelp and sargassum.10 Alginate oligosaccharides, the degradation products of alginate,11 show many physiological activities, such as anti-tumour activity,12 immunomodulatory activity,13 antioxidant activity,14 promoting plant growth15 and so on, and thus they have broad application prospects in biomedicine,16 food industry,17 agriculture18 and other fields.
Antibiotics refer to a class of secondary metabolites produced by microorganisms or other animals and plants in the process of growth and reproduction, which can inhibit the proliferation of microorganisms and other cells.19 Azithromycin, a kind of macrolide antibiotic, was designed to be more readily absorbed with a broad antibacterial spectrum against Gram-negative bacteria, especially Bordetella pertussis and Legionella species.20 Therefore, it has been extensively used for the treatment of bacterial infections such as pneumonia, chlamydia, and typhoid.21 Moreover, azithromycin was found to be the most commonly used antibiotic in a three-year cohort study involving more than 30 million people.22 However, because of its extensive application, there exist many cases of irrational use and even abuse, which might cause bacterial drug resistance,23 and even lead to gut microbiota imbalance.24,25 Severe gut microbiota imbalance is likely to bring about a series of clinical symptoms such as inflammatory bowel disease and antibiotic related diarrhea.26,27 A large number of beneficial bacteria become extinct, whereas specific harmful bacteria will take the opportunity to reproduce, then inducing double infections, which may cause serious adverse consequences.28 Studies have unveiled that prebiotics can promote the proliferation of intestinal probiotics and regulate the structure of the gut microbiota disturbed by antibiotics. For example, an in vivo study found that the richness and diversity of the gut microbiota as well as the production of short chain fatty acids in mice after oral administration of cefixime were significantly reduced, whereas the supplementation of tea polyphenols enhanced the richness and diversity of the gut microbiota reduced by antibiotic administration, and increased the relative abundance of probiotics such as Lactobacillus, Akkermansia, Blautia, Roseburia and Eubacterium.29 Another study revealed that after amoxicillin administration, Klebsiella, Escherichia–Shigella and other symbiotic–pathogenic bacteria were enriched, and probiotics such as Bifidobacterium and Lactobacillus were reduced, whereas inulin was observed to reverse the gut microbiota disorder induced by amoxicillin in mice.30
Although much attention has been paid to the intestinal microecological disorder caused by antibiotics, little information is available about the restoration of the azithromycin induced intestinal microecological disorder by alginate oligosaccharide. Therefore, we evaluated the disturbance effect of azithromycin on intestinal microecology in mice, as well as the repair effect of alginate oligosaccharide and its prebiotic compound on the disturbance with 16S rDNA sequencing and untargeted metabolomics analysis. The potential mechanism of the favourable effect of alginate oligosaccharide on the gut microbiota disorder was further revealed via microbiome and metabolome correlation analysis. This study may provide an innovative dietary strategy for the regulation of gut microbiota disorder.
2. Materials and methods
2.1. Animals and diets
SPF C57BL/6J mice (6-week-old, male), purchased from Jinan Pengyue Experimental Animal Technology Co., Ltd (Jinan, China), were housed in an environment at a temperature of 22 °C–25 °C, with a relative humidity of 50%–55% and a lighting cycle of 12 h day−1. After a week of accommodation, the mice (19.12 ± 0.12 g) were randomly divided into four groups with 11 mice per group, the control group (the NC group), azithromycin administration group (the AZ group), alginate oligosaccharide supplementation after azithromycin administration group (the AZ + AO group) and alginate oligosaccharide compound supplementation after azithromycin administration group (the AZ + Pre group). The mice were fed with common diets (Research Diets D12450B, HFK Bioscience Co., Ltd, Beijing, China). In the first week, the mice in the NC and the other three groups were given water and 50 mg kg−1 d−1 azithromycin (Macklin Biochemical Technology Co., Ltd, Shanghai, China, A832341), respectively (Fig. 1). In the second week, the mice in the NC, AZ and AZ + AO groups were given water, water and 10 g kg−1 d−1 alginate oligosaccharide with the degree of polymerization of 3–5 (Junlan Biotechnology Co., Ltd, Nanjing, China), respectively. The mice in the AZ + Pre group were given 3.75 g kg−1 d−1 alginate oligosaccharide with the degree of polymerization of 3–5, 3.75 g kg−1 d−1 inulin (Orafti®, Beneo, Chile), 1.25 g kg−1 d−1 resistant starch (Yuanye Biotechnology Co., Ltd, Shanghai, China, S24572) and 1.25 g kg−1 d−1 lactulose (J&K Scientific, Beijing, China, 598584) (Fig. 1). Diet and water were freely available for all mice, and body weight was recorded once a week. Animal experiments were carried out at Yantai University, and all procedures were approved by the Animal Ethics Association of Yantai University (YT2019-p-12).
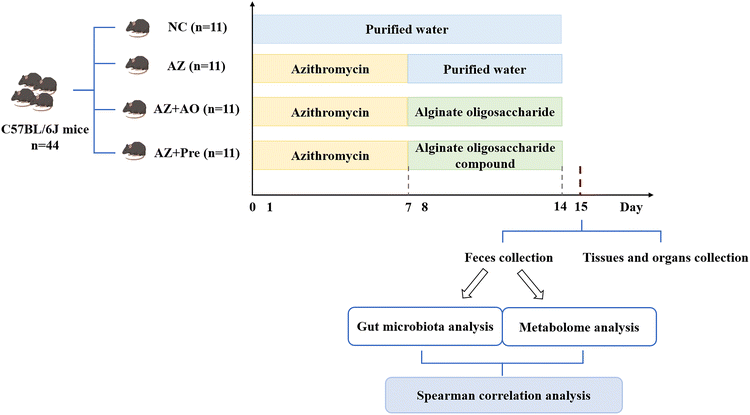 |
| Fig. 1 Experimental procedure. NC, normal chow diet; AZ, azithromycin administration; AZ + AO, azithromycin administration + alginate oligosaccharide supplementation; AZ + Pre, azithromycin administration + compound prebiotic supplementation. | |
2.2. Samples collection
The feces were collected at the end of the second week (Fig. 1) with the mice being separately placed in the sterilized cages. Fecal samples were collected individually into the sterile tubes and were snap-frozen in liquid nitrogen, then transferred to a −80 °C refrigerator. All operations were completed within 2 h. The blood samples were collected from mice orbits, and anticoagulated with EDTA, and the plasma was collected after centrifugation. Tissues and organs were collected freshly and weighed immediately after the mice were sacrificed at the end of the second week.
2.3. Metabolome analysis
The fecal samples ground with liquid nitrogen were extracted with 80% methanol and 0.1% formic acid, the supernatant was collected after centrifugation, and the samples were analyzed by LC-MS/MS,31 which was performed using a Vanquish UHPLC chromatograph (Thermo Fisher, Germany) coupled with a Q Exactive™ HF-X mass spectrometer (Thermo Fisher, Germany). The samples were gradient-eluted using a Hypesil Gold chromatographic column (100 × 2.1 mm, 1.9 μm, Thermo Fisher, USA) with a flow rate of 0.2 mL min−1 and a column temperature of 40 °C. The Q Exactive™ HF-X mass spectrometer was operated in the positive and negative polarity modes. The untargeted metabolomics raw data were submitted to the National Microbiology Data Center (NMDC) under the accession number NMDCX0000151. Statistical analysis of metabolites was performed using the statistical software R (R version R-3.4.3), Python (Python 2.7.6 version) and CentOS (CentOS release 6.6).
2.4. Gut microbiota analysis
DNA was extracted from the fecal samples, and the V3–V4 regions of 16S rRNA coding sequences were amplified by the primer of 515F (‘GTGCCAGCMGCCGCGGTAA’) and 806R (‘GGACTACHVGGGTWTCTAAT’). A TruSeq® DNA PCR-Free Sample Preparation Kit (Illumina, USA) was used to construct the library. The constructed library was quantified by Qubit and Q-PCR. After the library was quantified, it was sequenced via NovaSeq6000 (Illumina, USA) supported by Novogene Co., Ltd (Beijing, China). The 16S rDNA sequencing raw data were deposited in NCBI Sequence Read Archive (SRA) under the accession number PRJNA900557. OTU clustering and taxa annotation (taxa relative abundance analysis and genus evolutionary analysis), multi-sample comparative analysis (NMDS analysis and LEfSe analysis), the significance test of community structural differences among the groups (ANOSIM analysis) were used for sequence analysis. Taxa with significant differences among the groups were analyzed by MetaStat, and the correlations between microbiota and metabolites were analyzed by Spearman correlation analysis.
2.5. Statistical analysis
One-way analysis of variance (ANOVA) was performed using the SPSS software (version 24.0; SPSS Inc., IL, USA). Analysis of differences between groups was carried out using one-way analysis of variance (ANOVA). Data were presented as the mean ± SEM. p < 0.05 was considered to be statistically significant (* p < 0.05, ** p < 0.01).
3. Results
3.1. Body, tissues and organs weight
Body weight analysis showed that there were no significant differences in weight gain among the four groups within two weeks (Fig. 2A). Tissues and organs weight analysis showed that there was no significant difference in the mass of other tissues except colon. After two weeks of the experiment, the colon weight in the AZ group was 92.36 mg higher than that in the NC group, the colon weight in the AZ + Pre group was 134.82 mg higher than that in the AZ group, and the colon weight in the AZ + Pre group was 128.82 mg higher than that in the AZ + AO group, and there was no significant difference between the AZ + AO group and the AZ group (Fig. 2G).
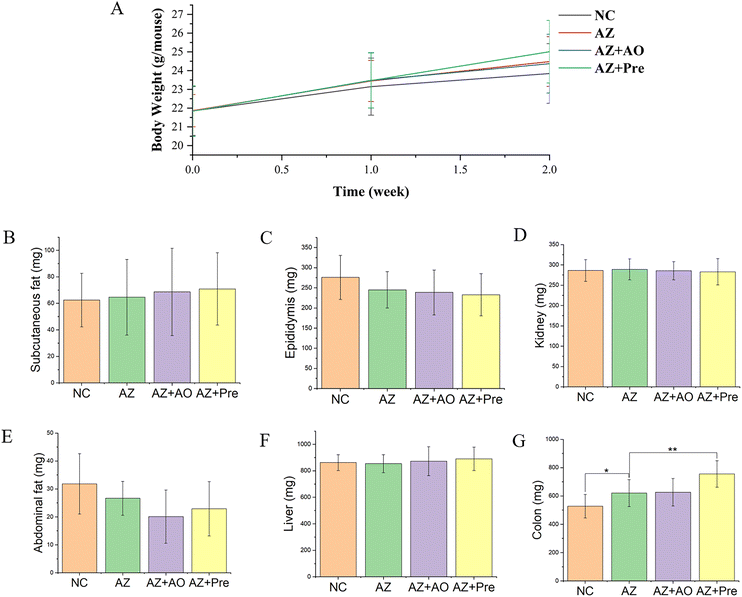 |
| Fig. 2 Body weight changes, tissues and organs weight of mice after two weeks. A. Body weight changes; Tissues and organs weight of B. subcutaneous fat weight; C. epididymis weight; D. kidney weight; E. abdominal fat weight; F. liver weight; G. colon weight. * p < 0.05, ** p < 0.01. NC, normal chow diet; AZ, azithromycin administration; AZ + AO, azithromycin administration + alginate oligosaccharide supplementation; AZ + Pre, azithromycin administration + compound prebiotic supplementation. | |
3.2. Changes in the gut microbiota structure and composition
The differences in the gut microbiota composition among the four groups were analysed with the NMDS (Non-Metric Multi-Dimensional Scaling) model (Fig. 3A), combined with Anosim analysis (Analysis of Similarities) (Fig. 3B). It demonstrated that there were distinct differences in the gut microbiota composition among the four groups (p < 0.05), and the differences were significant (R > 0).
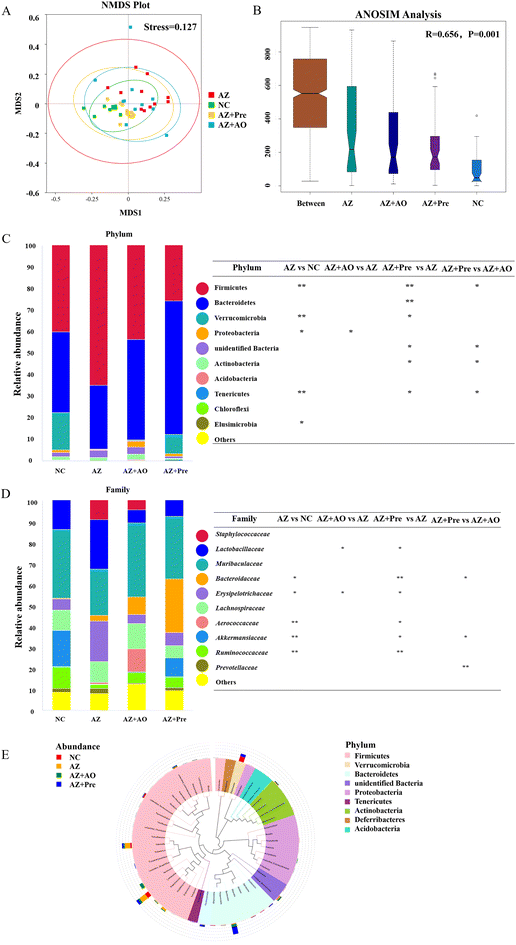 |
| Fig. 3 Gut microbiota composition and structure analysis. A. Non-metric dimensional scaling (NMDS) plots of the gut microbiota; B. Analysis of similarities (ANOSIM) among the NC, AZ, AZ + AO and AZ + Pre groups; The relative abundance of C. the top ten phyla and D. the top ten families; E. Phylogenetic tree at the genus level. * p < 0.05, ** p < 0.01. NC, normal chow diet; AZ, azithromycin administration; AZ + AO, azithromycin administration + alginate oligosaccharide supplementation; AZ + Pre, azithromycin administration + compound prebiotic supplementation. | |
The gut microbiota analysis at the phylum level (Fig. 3C) showed that the predominant microbial phyla of the four groups were Firmicutes and Bacteroidetes. In the NC group, the sum of Firmicutes (40.1%), Bacteroidetes (37.5%) and Verrucomicrobia (17.3%) accounted for about 94.9% of the community abundance. Compared with the NC group, the abundance of Firmicutes significantly increased (p < 0.01) to 65.0%, that of Bacteroidetes decreased to 29.5%, and that of Verrucomicrobia decreased significantly (p < 0.01) after azithromycin administration. After the supplementation of alginate oligosaccharide, the abundance of Firmicutes decreased back to 43.7%, that of Bacteroidetes increased to 46.6% and that of Verrucomicrobia increased to 0.28%, suggesting that alginate oligosaccharide was helpful to the recovery of the gut microbiota structure. Compared with the AZ group, the abundance of Firmicutes was significantly inhibited (p < 0.01), and that of Bacteroidetes (p < 0.01) and Verrucomicrobia (p < 0.05) was significantly increased after the supplementation of the alginate oligosaccharide compound. This indicated that the alginate oligosaccharide compound was favourable for the recovery of the gut microbiota structure. The gut microbiota analysis at the family level (Fig. 3D) showed that the predominant microbial families of the four groups were Lactobacillaceae and Muribaculaceae. In the NC group, Lactobacillaceae (13.9%) and Muribaculaceae (32.7%) accounted for about 46.6% of the community abundance. Compared with the NC group, the abundance of Lactobacillaceae increased to 23.7%, and the abundance of Muribaculaceae decreased to 22.0% after azithromycin administration. After the supplementation of alginate oligosaccharide, the abundance of Lactobacillaceae decreased significantly (p < 0.05) to 6.4%, and the abundance of Muribaculaceae increased to 35.2%, indicating that alginate oligosaccharide was helpful to the recovery of the gut microbiota structure. After the supplementation of the alginate oligosaccharide compound, the abundance of Lactobacillaceae was significantly inhibited (p < 0.05) and the abundance of Muribaculaceae was increased compared with the AZ group, which further showed that the alginate oligosaccharide compound was favourable for the recovery of the gut microbiota structure.
The evolutionary tree at genus level (Fig. 3E) showed that the top 50 genera in the four groups were mainly distributed in Firmicutes, followed by Bacteroidetes and Proteobacteria. Among all the top 50 genera, Akkermansia, Dubosiella, Lactobacillus and Bacteroides had higher relative abundance in the four groups.
3.3. Changes in the gut microbiota at different levels
MetaStat analysis of the microbial community structure at genus level (Fig. 4A) showed that in the AZ group, the relative abundance of Helicobacter, Alloprevotella, Akkermansia, Bifidobacterium, Candidatus Stoquefichus, Desulfovibrio, Parasutterella, unidentified Ruminococcaceae, Odoribacter, Erysipelatococcidium and Robinsoniella significantly reduced (p < 0.05), and the relative abundance of Dubosiella, Jeotgalicoccus, Roseburia, Staphylococcus and Aerococcus significantly increased (p < 0.05) compared with the NC group. This change was reversed by alginate oligosaccharide compound. After the supplementation of alginate oligosaccharide, the relative abundance of Dubosiella and Lactobacillus decreased significantly (p < 0.05), while the relative abundance of Acinetobacter, Alloprevotella, unidentified Ruminococcaceae, Odoribacter, Erysipelatococcidium, Ileibacterium and Parasutterella increased significantly (p < 0.05). Single alginate oligosaccharide could only partially reverse the gut microbiota disorder induced by azithromycin administration. MetaStat analysis of the microbial community structure at species level (Fig. 4B) showed that the relative abundance of Clostridiales bacterium 42-27, Helicobacter ganmani, Robinsoniella sp. KNHs210, Ruminococcus flavefaciens, Clostridium sp. MC 40 and Parabacteroides goldsteinii decreased significantly (p < 0.05), and the relative abundance of Staphylococcus xylosus, Staphylococcus lentus, Aerococcus viridans and Bradyrhizobium elkanii increased significantly (p < 0.05) after azithromycin administration. This change was significantly restored by alginate oligosaccharide compound (p < 0.05). 16 genera were decreased by azithromycin, and 14 of them were increased by alginate oligosaccharide compound, with a recovery rate of 87.5%. 19 genera were increased by azithromycin, and 13 of them were decreased by alginate oligosaccharide compound, with a recovery rate of 68.4%. Moreover, 16 species were decreased by azithromycin and 15 of them were increased by alginate oligosaccharide compound, and its recovery rate was 93.8%. 19 species were increased by azithromycin and 19 of them were decreased by alginate oligosaccharide compound, and its recovery rate was 100%. Additionally, azithromycin administration also significantly reduced (p < 0.05) the relative abundance of Lactobacillus reuteri, Lactobacillus intestinalis and Ileibacterium valens, and significantly increased (p < 0.05) the relative abundance of Lachnospiraceae bacterium DW12, Ruminococcus bromii, Clostridium sp. ASF356 and Lachnospiraceae bacterium 615. The alginate oligosaccharide compound also significantly increased (p < 0.05) the relative abundance of Bacteroides acidifaciens, Lachnospiraceae bacterium DW17, Enterococcus gallinarum and Enterobacter sp. FY-07, and significantly decreased (p < 0.05) the relative abundance of Lachnospiraceae bacterium A2. After the supplementation of alginate oligosaccharide, the relative abundance of Bacteroides acidifaciens, Lachnospiraceae bacterium DW12, Clostridiales bacterium 42-27, Ileibacterium valens, Burkholderiales bacterium YL45, Ruminococcus bromii and Enterobacter sp. FY-07 increased significantly (p < 0.05). Single alginate oligosaccharide could only partially reverse the gut microbiota disorder induced by azithromycin administration.
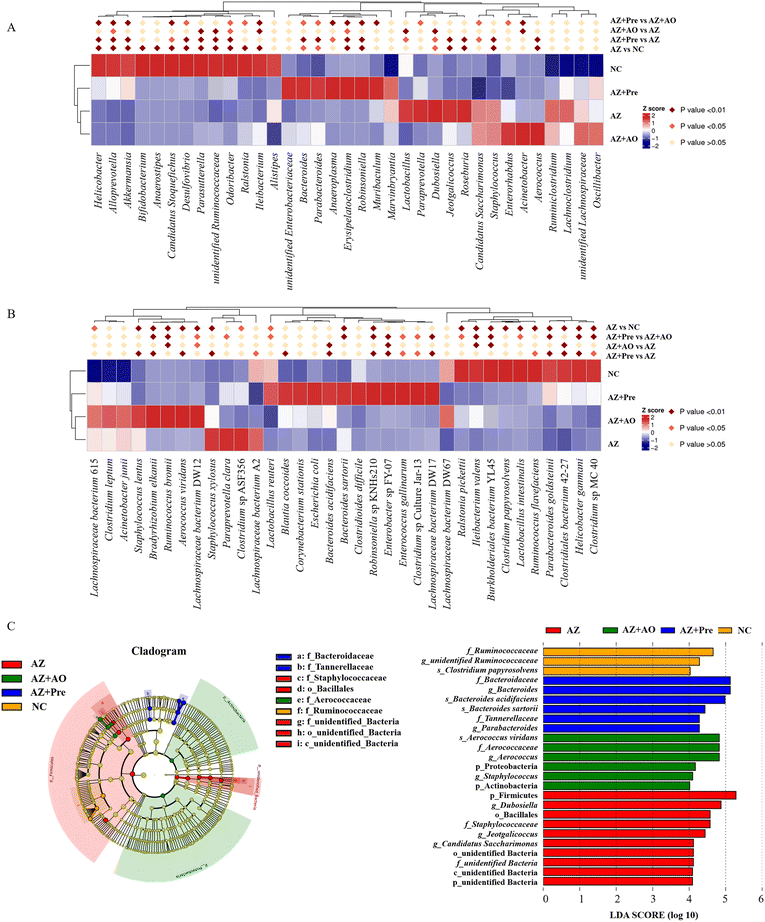 |
| Fig. 4 Changes of the gut microbiota at different levels. A. Statistical analysis of top thirty-five different genera in the four groups; B. Statistical analysis of top thirty-five different species in the four groups; C. LEfSe analysis among the four groups. * p < 0.05, ** p < 0.01. NC, normal chow diet; AZ, azithromycin administration; AZ + AO, azithromycin administration + alginate oligosaccharide supplementation; AZ + Pre, azithromycin administration + compound prebiotic supplementation. | |
LEfSe analysis (Fig. 4C) showed that there were 25 taxa with significant differences among the four groups (LDA score >4). Unidentified Ruminococcaceae, Clostridium papyrosolvens and other bacteria were enriched in the NC group. After azithromycin administration, Dubosiella, Jeotgalicocus, Candidatus Saccharimonas and other bacteria were enriched. After the supplementation of alginate oligosaccharide, Aerococcus viridans, Staphylococcus and other bacteria were enriched. After the supplementation of the alginate oligosaccharide compound, Bacteroides acidifaciens, Bacteroides sartorii, Parabacteroides and other bacteria were enriched, and these taxa demonstrated significant differences in the abundance among the four groups. Moreover, the t-test was conducted with 95% confidence interval among the four groups. The significantly different species were shown in Fig. 5.
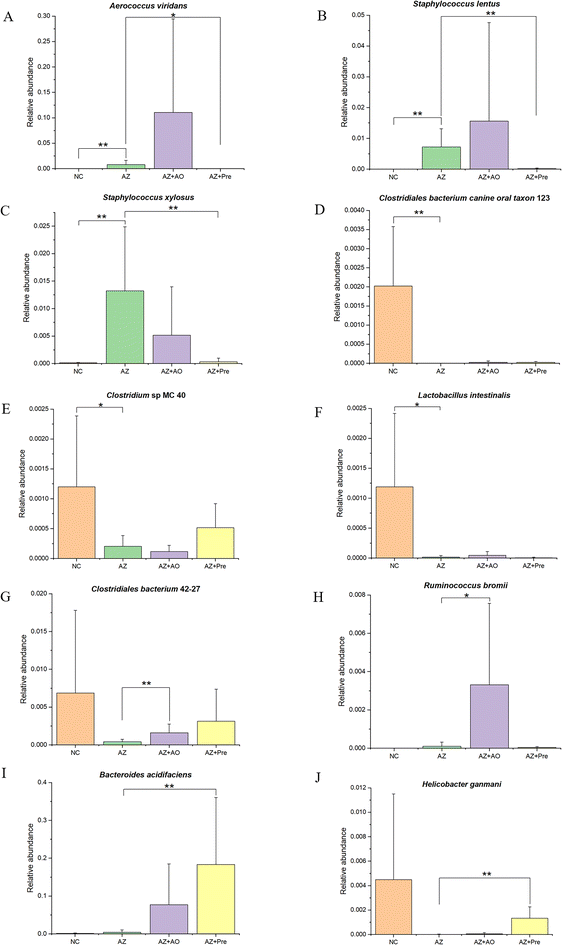 |
| Fig. 5 Differential species among the four groups. A.Aerococcus viridans; B.Staphylococcus lentus; C.Staphylococcus xylosus; D.Clostridiales bacterium canine oral taxon 123; E.Clostridium sp. MC 40; F.Lactobacillus intestinalis; G.Clostridiales bacterium 42-27; H.Ruminococcus bromii; I.Bacteroides acidifaciens; J.Helicobacter ganmani. * p < 0.05, ** p < 0.01. NC, normal chow diet; AZ, azithromycin administration; AZ + AO, azithromycin administration + alginate oligosaccharide supplementation; AZ + Pre, azithromycin administration + compound prebiotic supplementation. | |
3.4. Metabolites changes and related metabolic pathways
The volcano plot of the differential metabolites (Fig. 6A) showed that a total of 197 metabolites were up-regulated and 212 metabolites were down-regulated in the AZ vs. NC group. 183 metabolites were up-regulated and 254 metabolites were down-regulated in the AZ + AO vs. AZ group. A total of 178 metabolites were up-regulated and 196 metabolites were down-regulated in the AZ + Pre vs. AZ group.
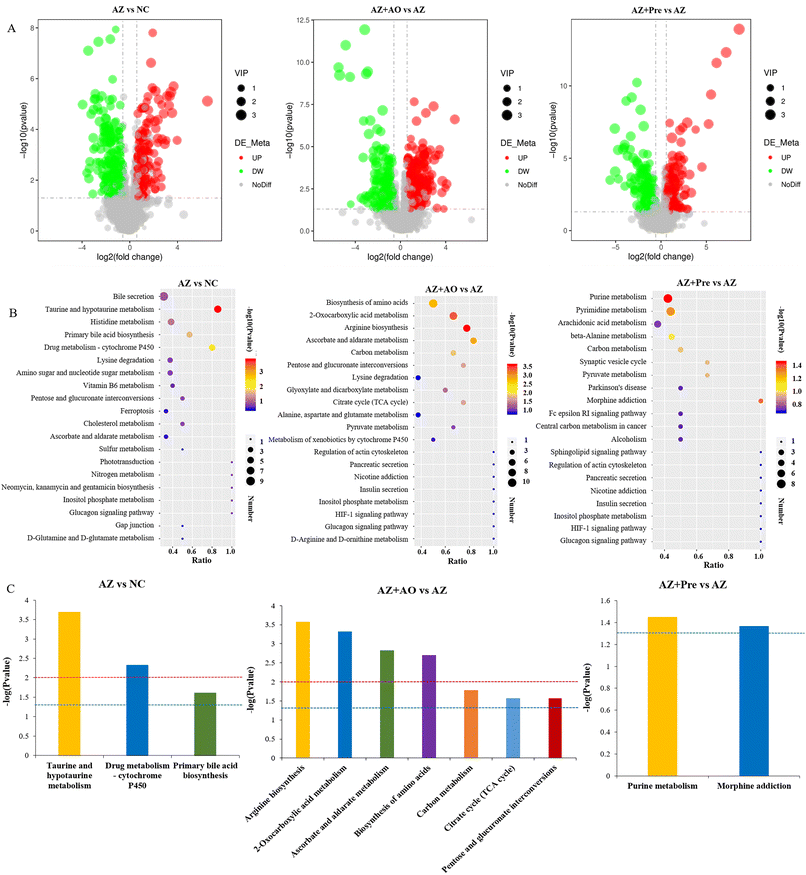 |
| Fig. 6 Azithromycin and alginate oligosaccharide changed the composition and pathways of metabolites. A. Differential metabolites volcano plot; B. KEGG enrichment bubble plot; C. KEGG enrichment pathways (p < 0.05). * p < 0.05, ** p < 0.01. NC, normal chow diet; AZ, azithromycin administration; AZ + AO, azithromycin administration + alginate oligosaccharide supplementation; AZ + Pre, azithromycin administration + compound prebiotic supplementation. | |
The KEGG pathways of the top 20 in the total number of differential metabolites were shown in Fig. 6B. After azithromycin administration, there were more differential metabolites in bile secretion, taurine and hypotaurine metabolism, histidine metabolism, primary bile acid biosynthesis, drug metabolism – cytochrome P450, lysine degradation, and amino sugar and nucleoside sugar metabolism. After alginate oligosaccharide supplementation, there were more differential metabolites in the biosynthesis of amino acids, 2-oxocarboxylic acid metabolism, arginine biosynthesis, ascorbate and aldarate metabolism, carbon metabolism, pentose and glutamate interconversion, lysine degradation, glyoxylate and dicarboxylate metabolism, citrate cycle (TCA cycle), and alanine, aspartate and glutamate metabolism. After the supplementation of the alginate oligosaccharide compound, there were more differential metabolites in purine metabolism, pyrimidine metabolism, arachidonic acid metabolism, beta alanine metabolism and carbon metabolism. The significantly differential KEGG pathways (p < 0.05) were shown in Fig. 6C. They showed that after azithromycin administration, taurine and hypotaurine metabolism, drug metabolism-cytochrome P450 and primary bile acid biosynthesis were significantly different from those in the NC group. After alginate oligosaccharide supplementation, arginine biosynthesis, 2-oxocarboxylic acid metabolism, ascorbate and aldarate metabolism, biosynthesis of amino acids, carbon metabolism, citrate cycle (TCA cycle), and pentose and gluconate interconversion were significantly different from those in the AZ group. Significant differences in purine metabolism and morphine addiction were observed in the AZ + Pre group compared with the AZ group.
3.5. Differential metabolites composition changes
Differential metabolites were classified as amino acids and their derivatives, nucleotide related metabolites, vitamins and their derivatives, bile acid related metabolites, unsaturated fatty acids, TCA cycle intermediates and their related metabolites, glucose derivatives, neuroactive substances and other organic acids metabolites (Fig. 7A). Hierarchical clustering analysis of differential metabolites (Fig. 7A, left) showed that alginate oligosaccharide and alginate oligosaccharide compound increased the contents of metabolites such as ursodeoxycholic acid, taurocholic acid, alpha-ketoglutaric acid, fumaric acid, citric acid, and ascorbic acid, and decreased the contents of fexofenadine, L-histidinol, L-ornithine, morphine, hypotaurine, sulfoacetic acid, citrulline, glucuronic acid and taurine. Spearman correlations analysis (Fig. 7A, right) showed that Bacteroides acidifaciens was significantly positively correlated with taurocholic acid, alpha-ketoglutaric acid and citric acid, and significantly negatively correlated with L-histidinol, 3-hydroxylidocaine and citrulline. Lachnospiraceae bacterium DW17 was positively correlated with 2′-deoxyguanosine, 2′-deoxyadenosine and inosine, and negatively correlated with sulfoacetic acid. There were significantly negative correlations between Clostridiales bacterium 42-27 and hypotaurine, L-cysteinesulfinic acid and taurine. Additionally, Lachnospiraceae bacterium DW12 was negatively correlated with citrullinne, L-histidinol and 3-hydroxylidocaine.
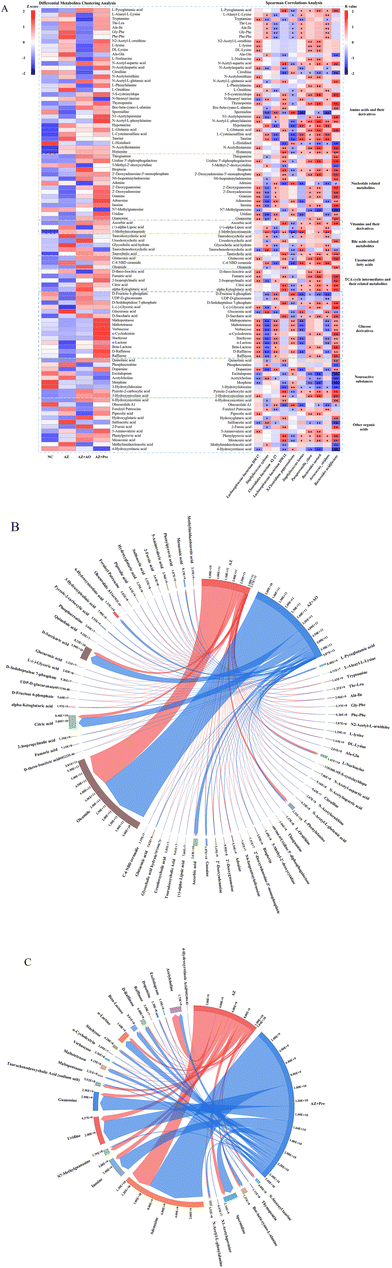 |
| Fig. 7 Differential metabolites analysis. A. Differential metabolites clustering analysis and spearman correlations analysis between the levels of differential metabolites and the top 10 gut bacterial species in abundance. B. Chord diagram of differential metabolites which were significantly regulated by alginate oligosaccharide compared with the AZ group. C. Chord diagram of differential metabolites which were significantly regulated by alginate oligosaccharide compound compared with the AZ group. * p < 0.05, ** p < 0.01. NC, normal chow diet; AZ, azithromycin administration; AZ + AO, azithromycin administration + alginate oligosaccharide supplementation; AZ + Pre, azithromycin administration + compound prebiotic supplementation. | |
We screened all differential metabolites affected by alginate oligosaccharide and its compound (AZ + AO vs. AZ, p < 0.05; AZ + Pre vs. AZ, p < 0.05). 64 and 25 differential metabolites were screened in the AZ + AO group and AZ + Pre group, respectively (Fig. 7B and C). The supplementation of alginate oligosaccharide mainly increased the content of oleamide. Alginate oligosaccharide compound supplementation mainly increased the content of adenosine.
3.6. FAHFAs, a series of novel lipids for maintaining glucose homeostasis, significantly increased
In the comparison between the NC group and the AZ group, the FAHFAs were significantly reduced (Fig. 8A). Compared with the AZ group, except that the content of FAHFA (18:1/20:2) was decreased by alginate oligosaccharide, other FAHFAs were significantly increased in the AZ + AO group. In the comparison between the AZ group and the AZ + Pre group, except that the contents of FAHFA (2:0/18:1) and FAHFA (4:0/24:2) were reduced by alginate oligosaccharide compound, other FAHFAs were significantly increased (Fig. 8A).
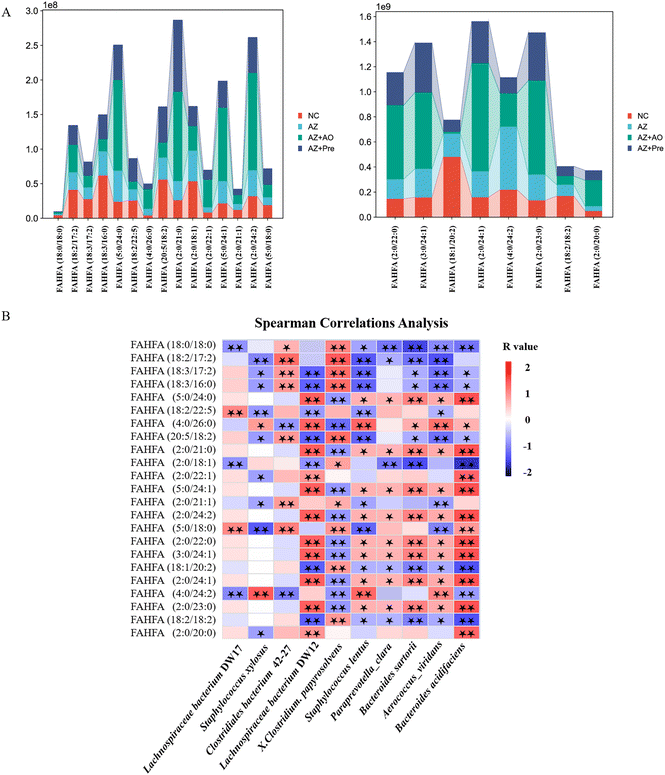 |
| Fig. 8 Effects of alginate oligosaccharide and its compound at the FAHFAs levels. A. Differential FAHFAs analysis; B. Spearman correlations analysis between the levels of FAHFAs and the top 10 gut bacterial species in abundance. * p < 0.05, ** p < 0.01. NC, normal chow diet; AZ, azithromycin administration; AZ + AO, azithromycin administration + alginate oligosaccharide supplementation; AZ + Pre, azithromycin administration + compound prebiotic supplementation. | |
Among the 23 differential FAHFAs, FAHFA (2:0/18:1), FAHFA (18:1/20:2) and FAHFA (18:2/18:2) levels were significantly negatively correlated with Bacteroides acidifaciens populations (p < 0.01) and FAHFA (5:0/24:0), FAHFA (2:0/21:0), FAHFA (5:0/24:1), FAHFA (2:0/24:2), FAHFA (2:0/22:0), FAHFA (3:0/24:1), FAHFA (2:0/24:1) and FAHFA (2:0/23:0) contents were significantly positively correlated with Bacteroides acidifaciens populations (p < 0.01) (Fig. 8B).
4. Discussion
In this experiment, mice treated with azithromycin were used as the intestinal microecology disorder model to explore the regulatory effect of alginate oligosaccharide and its compound on microecological dysbiosis (Fig. S1 and S2†). The analysis of gut microbiota composition in mice (Fig. 3A and B) showed that there were significant differences in the composition of gut microbiota among the four groups, indicating that the intervention of azithromycin, alginate oligosaccharide and its compound significantly changed the structure and composition of the gut microbiota. At the phylum level, after azithromycin administration, the proportion of Firmicutes and Bacteroidetes in the gut microbiota increased (Fig. 3C). Firmicutes and Bacteroidetes are the predominant microbial phyla in the intestine, whose increase is related to the increased incidence of obesity,32 diabetes,33 and gastrointestinal cancer.34 After the supplementation of alginate oligosaccharide and its compound, an obvious decrease of the Firmicutes and Bacteroidetes proportion was observed (Fig. 3C), indicating that alginate oligosaccharide and its compound reversed the gut microbiota imbalance by reducing the proportion of Firmicutes and Bacteroidetes. Therefore, they are expected to reduce the risk of obesity, diabetes and gastrointestinal cancer as a kind of food supplement.
Akkermansia is a representative genus of Verrucomicrobia phylum in the intestine. Akkermansia is known to play an essential role in maintaining intestinal health and host metabolic regulation,35 which can protect intestinal barrier integrity and lessen the symptoms of alcoholic liver disease.36 Therefore, it is expected to be a treatment target for the gut microbiota related diseases, such as metabolic syndrome, immune diseases and cancer.37 Hence, Akkermansia genus is considered as a kind of probiotic. In our study, MetaStat analysis (Fig. 4A) demonstrated that the abundance of Akkermansia decreased significantly (p < 0.01) after azithromycin administration, whereas the abundance of Akkermansia increased significantly (p < 0.01) after alginate oligosaccharide compound supplementation. Therefore, alginate oligosaccharide compound can not only restore the gut microbiota balance, but also potentially improve metabolic syndrome, immune diseases and cancer by increasing the abundance of Akkermansia. Besides the significant modulation on Akkermansia, alginate oligosaccharide compound almost completely restored the gut microbiota balance in mice, but single alginate oligosaccharide could only partially reverse the gut microbiota balance, which suggests that the effect of alginate oligosaccharide compound on restoring the gut microbiota balance is more significant than that of single alginate oligosaccharide. Therefore, diverse diets can better reduce the risk of intestinal microecology disorder compared with single diets.
Bifidobacterium decreased significantly after azithromycin administration (p < 0.01) (Fig. 4A). Bifidobacterium is a kind of intestinal probiotic, which contributes to alleviating obesity and inflammation outcomes,38 inhibiting tumor39 and oxidation.40 Additionally, azithromycin administration significantly increased the relative abundance of Staphylococcus (p < 0.01) (Fig. 4A). Staphylococcus is a group of Gram-positive pyococcus bacteria, which is a major cause of human and animal infections.41 However, alginate oligosaccharide compound significantly reduced the relative abundance of Staphylococcus (p < 0.01). Therefore, azithromycin administration increased the abundance of conditional pathogens, and reduced the abundance of probiotics, thus causing intestinal microecological disorders, and alginate oligosaccharide compound restored the intestinal microecology by reverse modulation of these taxa.
The gut microbiota analysis (Fig. 4B) demonstrated that the abundance of Bacteroides acidifaciens increased significantly after the supplementation of alginate oligosaccharide and alginate oligosaccharide compound (p < 0.01). Bacteroides acidifaciens, which belongs to Bacteroidetes, is classified as one of the dominant species of intestinal commensal bacteria.42 The relative abundance of Bacteroides genus enriched in the mice intestine fed with soluble fiber, namely Bacteroides acidifaciens,43 which was consistent with our research results. Moreover, Bacteroides acidifaciens was involved in the production of Immunoglobulin A (IgA) in the intestine.44 Therefore, alginate oligosaccharide and its compound supplementation potentially enhance the immune function and improve the intestinal microecology disorder by increasing the abundance of Bacteroides acidifaciens.
Untargeted metabolomics analysis of metabolites revealed that alginate oligosaccharide and its compound enhanced the TCA cycle (Fig. 6B and C), and remarkably increased (p < 0.05) the levels of citric acid, α-ketoglutaric acid and fumaric acid, intermediate products of the TCA cycle (Fig. 7A, left), specifying that the supplementation of alginate oligosaccharide and its compound promoted the TCA cycle in mice. The TCA cycle, a major metabolic pathway in the host and a hub for the connection and transformation of sugar, lipid and protein metabolism, is of vital significance in the metabolic cycle. A study found that enhancing the TCA cycle could inhibit tumour growth and metastasis. This finding might help to clarify the TCA cycle role in cancer progression and metastasis, and provide a potential strategy to address cancer metastasis.45
Moreover, the mice supplemented with alginate oligosaccharide and alginate oligosaccharide compound displayed a significant increase (p < 0.01) in ascorbic acid content. It was found that ascorbic acid could inhibit the biological activity of inflammatory T cells by altering cell metabolism, thereby attenuating inflammatory bowel disease,46 and thus alginate oligosaccharide may provide insight into relevant therapeutic approaches as a potential treatment. Additionally, the supplementation of alginate oligosaccharide and alginate oligosaccharide compound significantly increased the bile acid related metabolites, such as ursodeoxycholic acid and taurocholic acid (p < 0.05), which were disturbed by azithromycin. Ursodeoxycholic acid (UDCA) could attenuate the neonatal dairy calves’ diarrhea caused by extended-spectrum β-lactamase-producing enteroaggregative Escherichia coli infection.47 Taurocholic acid could be hydrolysed by bile salt hydrolases (BSHs) to cholic acid, and subsequently metabolized into deoxycholic acid, which potently inhibited the growth of Clostridium difficile.48 Hence, alginate oligosaccharide might exhibit anti-cancer, anti-inflammatory or intestinal barrier enhancing effects on the host by increasing the levels of citric acid, fumaric acid, α-ketoglutaric acid, ascorbic acid, ursodeoxycholic acid, taurocholic acid and other bile acid related metabolites.
Notably, spearman correlations analysis of the gut microbiota and its metabolites (Fig. 7A, right) showed that Bacteroides acidifaciens was significantly positively correlated with citric acid and α-ketoglutaric acid, the intermediate products of the TCA cycle. Citrate has been revealed to play a pivotal role in regulating energy balance by stimulating fatty acid synthesis, and inhibiting glycolysis and fatty acid β-oxidation pathways.49α-Ketoglutaric acid has been proved to have anti-inflammatory, reducing oxidative stress, anti-tumour, anti-aging and other effects.50 Furthermore, Bacteroides sartorii demonstrated a significantly positive correlation with fumaric acid, the intermediate product of the TCA cycle. Therefore, the significant stimulation of the TCA cycle may be related to the increase of Bacteroides acidifaciens and Bacteroides sartorii. Our previous study demonstrated that Bacteroides acidifaciens and Bacteroides ovatus were significantly positively correlated with the increase of intermediates of the TCA cycle, such as citrate, isocitrate and malate, with a significant enhancement of the TCA cycle.51 Moreover, other studies reported that the promotion of the TCA cycle was significantly positively correlated with the increase of Bacteroides genus.52 Therefore, Bacteroides genus may play a cardinal regulatory role in energy balance through the TCA cycle to a certain extent.
Fatty acid esters of hydroxy fatty acids (FAHFAs) play a considerable role in maintaining glucose homeostasis. FAHFAs could activate G protein coupled receptor 120 (GPR120) and promote the transport of glucose transporter GLUT-4, thereby enhancing the insulin-stimulated glucose uptake. Additionally, GPR120 activated by FAHFAs could reduce interleukin-1β (IL-1β) and tumour necrosis factor α (TNF-α) in macrophages, thus alleviating obesity related inflammation.53 Through the overall analysis of the obtained differential FAHFAs, it was observed that the contents of FAHFAs in mice after the supplementation of alginate oligosaccharide and alginate oligosaccharide compound increased significantly (Fig. 8A). Although we have not established the direct causal association between alginate oligosaccharide and the improved effects on glucose homeostasis and inflammation in the present study, there has been evidence that alginate oligosaccharide supplementation could improve the above metabolic parameters, including glucose homeostasis and inflammation in mice.54,55 Similarly, Bacteroides acidifaciens displayed a significantly positive correlation with a considerable part of FAHFAs through Spearman correlations analysis between the mice gut microbiota and the FAHFAs (Fig. 8B). Therefore, alginate oligosaccharide might play a crucial role in maintaining glucose homeostasis and alleviating inflammation through the Bacteroides acidifaciens–FAHFAs axis.
5. Conclusions
In conclusion, alginate oligosaccharide and its compound improved intestinal microecological disorder caused by azithromycin in mice via reducing the abundance of pathogenic bacteria such as Staphylococcus and increasing the abundance of beneficial bacteria, such as Akkermansia and Bacteroides acidifaciens. Additionally, alginate oligosaccharide compound had a better effect than alginate oligosaccharide on modulating intestinal homeostasis. Alginate oligosaccharide and its compound maintained the related metabolic homeostasis via increasing the contents of intermediate products of the TCA cycle, such as citric acid, fumaric acid and α-ketoglutaric acid. Moreover, the stimulation of the TCA cycle was related to the Bacteroides acidifaciens and Bacteroides sartorii populations increase, indicating that Bacteroides genus might play a regulatory role in energy balance through the TCA cycle. Furthermore, alginate oligosaccharide and its compound played crucial roles in maintaining intestinal homeostasis through the Bacteroides acidifaciens–FAHFAs axis (Fig. 9). Therefore, alginate oligosaccharide may become a kind of functional food additive and provide an innovative dietary strategy for the regulation of intestinal microecological disorders.
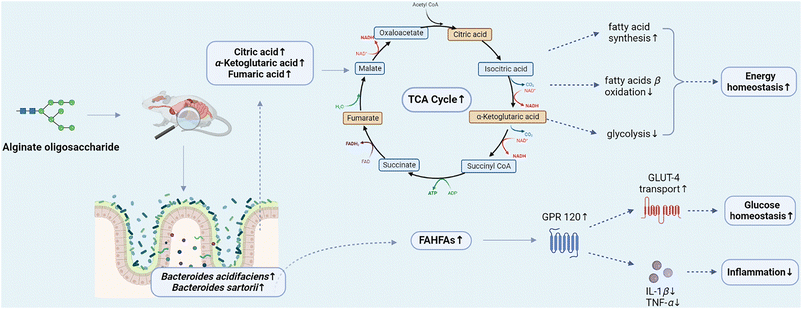 |
| Fig. 9 Alginate oligosaccharide ameliorates azithromycin-induced gut microbiota disorder via Bacteroides acidifaciens-FAHFAs and Bacteroides–TCA cycle axes. FAHFAs: fatty acid esters of hydroxy fatty acids; TCA cycle: tricarboxylic acid cycle; GPR120: G protein-coupled receptor 120; GLUT-4: glucose transporter 4; IL-1β: interleukin-1β; TNF-α: tumor necrosis factor-α. | |
Author contributions
Shuling Yan: methodology, data analysis, software, and writing – original draft. Yanhong Zhu: conceptualization, resources, supervision, writing – original draft, and project administration. Lili Li: conceptualization, data interpretation, supervision, funding acquisition, project administration, and writing – review & editing. Song Qin: supervision and project administration. Jingyi Yuan: conceptualization and methodology. Xiulian Chang: resources and project administration. Shanliang Hu: conceptualization and resources.
Conflicts of interest
The authors declared no competing interests.
Acknowledgements
This work was supported by the Science and Technology Program of Yantai (2020MSGY076).
References
- M. Roberfroid, Prebiotics: The concept revisited, J. Nutr., 2007, 137, 830S–837S CrossRef CAS PubMed.
- M. Blaut, Relationship of prebiotics and food to intestinal microflora, Eur. J. Nutr., 2002, 41(Supplement 1), I11–I16 Search PubMed.
- L. B. Bindels, N. M. Delzenne, P. D. Cani and J. Walter, Towards a more comprehensive concept for prebiotics, Nat. Rev. Gastroenterol. Hepatol., 2015, 12, 303–310 CrossRef CAS PubMed.
- R. J. Palframan, G. R. Gibson and R. A. Rastall, Effect of pH and dose on the growth of gut bacteria on prebiotic carbohydrates in vitro, Anaerobe, 2002, 8, 287–292 CrossRef CAS PubMed.
- J. Wan, J. Zhang, D. Chen, B. Yu, X. Mao, P. Zheng, J. Yu, J. Luo and J. He, Alginate oligosaccharide-induced intestinal morphology, barrier function and epithelium apoptosis modifications have beneficial effects on the growth performance of weaned pigs, J. Anim. Sci. Biotechnol., 2018, 9, 58 CrossRef PubMed.
- S. Li, N. He and L. Wang, Efficiently anti-obesity effects of unsaturated alginate oligosaccharides (UAOS) in high-fat diet (HFD)-fed mice, Mar. Drugs, 2019, 17, 540 CrossRef CAS PubMed.
- Y. Li, L. Elmen, I. Segota, Y. Xian, R. Tinoco, Y. Feng, Y. Fujita, R. R. S. Munoz, R. Schmaltz, L. M. Bradley, A. Ramer-Tait, R. Zarecki, T. Long, S. N. Peterson and Z. e. A. Ronai, Prebiotic-induced anti-tumor immunity attenuates tumor growth, Cell Rep., 2020, 30, 1753–1766 CrossRef CAS PubMed.
- M. Peng, Z. Tabashsum, M. Anderson, A. Truong, A. K. Houser, J. Padilla, A. Akmel, J. Bhatti, S. O. Rahaman and D. Biswas, Effectiveness of probiotics, prebiotics, and prebiotic-like components in common functional foods, Compr. Rev. Food Sci. Food Saf., 2020, 19, 1908–1933 CrossRef CAS PubMed.
- J. Liu, S. Yang, X. Li, Q. Yan, M. J. T. Reaney and Z. Jiang, Alginate oligosaccharides: production, biological activities, and potential applications, Compr. Rev. Food Sci. Food Saf., 2019, 18, 1859–1881 CrossRef CAS PubMed.
- T. Y. Wong, L. A. Preston and N. L. Schiller, Alginate lyase: Review of major sources and enzyme characteristics, structure-function analysis, biological roles, and applications, Annu. Rev. Microbiol., 2000, 54, 289–340 CrossRef CAS PubMed.
- F. Xu, P. Wang, Y. Z. Zhang and X. L. Chen, Diversity of three-dimensional structures and catalytic mechanisms of alginate lyases, Appl. Environ. Microbiol., 2018, 84, e02040–e02017 Search PubMed.
- X. K. Hu, X. L. Jiang, H. M. Hwang, S. L. Liu and H. S. Guan, Antitumour activities of alginate-derived oligosaccharides and their sulphated substitution derivatives, Eur. J. Phycol., 2004, 39, 67–71 CrossRef CAS.
- M. Otterlei, K. Ostgaard, G. Skjakbraek, O. Smidsrod, P. Soonshiong and T. Espevik, Induction of cytokine production from human monocytes stimulated with alginate, J. Immunother., 1991, 10, 286–291 CrossRef CAS PubMed.
- W. Feng, Y. Hu, N. An, Z. Feng, J. Liu, J. Mou, T. Hu, H. Guan, D. Zhang and Y. Mao, Alginate oligosaccharide alleviates monocrotaline-induced pulmonary hypertension via anti-oxidant and anti-inflammation pathways in rats, Int. Heart J., 2020, 61, 160–168 CrossRef CAS PubMed.
- J. Li, X. Wang, X. Lin, G. Yan, L. Liu, H. Zheng, B. Zhao, J. Tang and Y.-D. Guo, Alginate-derived oligosaccharides promote water stress tolerance in cucumber (Cucumis sativus L.), Plant Physiol. Biochem., 2018, 130, 80–88 CrossRef CAS PubMed.
- M. B. Labowska, I. Michalak and J. Detyna, Methods of extraction, physicochemical properties of alginates and their applications in biomedical field - a review, Open Chem., 2019, 17, 738–762 CAS.
- M. Nishizawa, M. Saigusa and H. Saeki, Conjugation with alginate oligosaccharide via the controlled Maillard reaction in a dry state is an effective method for the preparation of salmon myofibrillar protein with excellent anti-inflammatory activity, Fish. Sci., 2016, 82, 357–367 CrossRef CAS.
- Q. D. An, G. L. Zhang, H. T. Wu, Z. C. Zhang, G. S. Zheng, L. Luan, Y. Murata and X. Li, Alginate-deriving oligosaccharide production by alginase from newly isolated Flavobacterium sp. LXA and its potential application in protection against pathogens, J. Appl. Microbiol., 2009, 106, 161–170 CrossRef CAS PubMed.
- M. A. Kohanski, D. J. Dwyer and J. J. Collins, How antibiotics kill bacteria: from targets to networks, Nat. Rev. Microbiol., 2010, 8, 423–435 CrossRef CAS PubMed.
- A. Firth and P. Prathapan, Azithromycin: The first broad-spectrum therapeutic, Eur. J. Med. Chem., 2020, 207, 112739 CrossRef CAS PubMed.
- W. R. Taylor, T. L. Richie, D. J. Fryauff, C. Ohrt, H. Picarima, D. Tang, G. S. Murphy, H. Widjaja, D. Braitman, E. Tjitra, A. Ganjar, T. R. Jones, H. Basri and J. Berman, Tolerability of azithromycin as malaria prophylaxis in adults in northeast papua, indonesia, Antimicrob. Agents Chemother., 2003, 47, 2199–2203 CrossRef CAS PubMed.
- M. J. Durkin, S. R. Jafarzadeh, K. Hsueh, Y. H. Sallah, K. D. Munshi, R. R. Henderson and V. J. Fraser, Outpatient antibiotic prescription trends in the United States: A national cohort study, Infect. Control Hosp. Epidemiol., 2018, 39, 584–589 CrossRef PubMed.
- S. Becattini, Y. Taur and E. G. Pamer, Antibiotic-induced changes in the intestinal microbiota and disease, Trends Mol. Med., 2016, 22, 458–478 CrossRef CAS PubMed.
- C. Ubeda and E. G. Pamer, Antibiotics, microbiota, and immune defense, Trends Immunol., 2012, 33, 459–466 CrossRef CAS PubMed.
- J. Wu, Z. Lin, X. Wang, Y. Zhao, J. Zhao, H. Liu, L. J. Johnston, L. Lu and X. Ma, Limosilactobacillus reuteri SLZX19-12 protects the colon from infection by enhancing stability of the gut microbiota and barrier integrity and reducing inflammation, Microbiol. Spectr., 2022, 10, e0212421 CrossRef PubMed.
- Y. Zhang, H. Liu, Z. Yue, P. Tan, M. Sun, L. Ji, Y. Bai and X. Ma,
Wickerhamomyces anomalus relieves weaning diarrhea via improving gut microbiota and redox homeostasis using a piglet model, Food Funct., 2022, 13, 11223–11235 RSC.
- N. Ma, Y. Sun, J. Chen, Z. Qi, C. Liu and X. Ma, Micro-coevolution of genetics rather than diet with enterotype in pigs, Front. Nutr., 2022, 9, 846974 CrossRef PubMed.
- Q. Guo, J. Z. Goldenberg, C. Humphrey, R. El Dib and B. C. Johnston, Probiotics for the prevention of pediatric antibiotic-associated diarrhea, Cochrane Database Syst. Rev., 2019, 4, CD004827 Search PubMed.
- J. Li, C. Chen, H. Yang and X. Yang, Tea polyphenols regulate gut microbiota dysbiosis induced by antibiotic in mice, Food Res. Int., 2021, 141, 110153 CrossRef CAS PubMed.
- H. Lin, Q. Wang, M. Yuan, L. Liu, Z. Chen, Y. Zhao, R. Das, Y. Duan, X. Xu, Y. Xue, Y. Luo and D. Mao, The prolonged disruption of a single-course amoxicillin on mice gut microbiota and resistome, and recovery by inulin, Bifidobacterium longum and fecal microbiota transplantation, Environ. Pollut., 2020, 265, 114651 CrossRef CAS PubMed.
- E. J. Want, P. Masson, F. Michopoulos, I. D. Wilson, G. Theodoridis, R. S. Plumb, J. Shockcor, N. Loftus, E. Holmes and J. K. Nicholson, Global metabolic profiling of animal and human tissues via UPLC-MS, Nat. Protoc., 2013, 8, 17–32 CrossRef CAS PubMed.
- P. J. Turnbaugh, R. E. Ley, M. A. Mahowald, V. Magrini, E. R. Mardis and J. I. Gordon, An obesity-associated gut microbiome with increased capacity for energy harvest, Nature, 2006, 444, 1027–1031 CrossRef PubMed.
- J. Qin, Y. Li, Z. Cai, S. Li, J. Zhu, F. Zhang, S. Liang, W. Zhang, Y. Guan, D. Shen, Y. Peng, D. Zhang, Z. Jie, W. Wu, Y. Qin, W. Xue, J. Li, L. Han, D. Lu, P. Wu, Y. Dai, X. Sun, Z. Li, A. Tang, S. Zhong, X. Li, W. Chen, R. Xu, M. Wang, Q. Feng, M. Gong, J. Yu, Y. Zhang, M. Zhang, T. Hansen, G. Sanchez, J. Raes, G. Falony, S. Okuda, M. Almeida, E. LeChatelier, P. Renault, N. Pons, J.-M. Batto, Z. Zhang, H. Chen, R. Yang, W. Zheng, S. Li, H. Yang, J. Wang, S. D. Ehrlich, R. Nielsen, O. Pedersen, K. Kristiansen and J. Wang, A metagenome-wide association study of gut microbiota in type 2 diabetes, Nature, 2012, 490, 55–60 CrossRef CAS PubMed.
- J. D. Galley, M. C. Nelson, Z. Yu, S. E. Dowd, J. Walter, P. S. Kumar, M. Lyte and M. T. Bailey, Exposure to a social stressor disrupts the community structure of the colonic mucosa-associated microbiota, BMC Microbiol., 2014, 14, 189 CrossRef PubMed.
- L. R. Lopetuso, A. Quagliariello, M. Schiavoni, V. Petito, A. Russo, S. Reddel, F. D. Chierico, G. Ianiro, F. Scaldaferri, M. Neri, G. Cammarota, L. Putignani and A. Gasbarrini, Towards a disease-associated common trait of gut microbiota dysbiosis: The pivotal role of Akkermansia muciniphila, Dig. Liver Dis., 2020, 52, 1002–1010 CrossRef CAS PubMed.
- C. Grander, T. E. Adolph, V. Wieser, P. Lowe, L. Wrzosek, B. Gyongyosi, D. V. Ward, F. Grabherr, R. R. Gerner, A. Pfister, B. Enrich, D. Ciocan, S. Macheiner, L. Mayr, M. Drach, P. Moser, A. R. Moschen, G. Perlemuter, G. Szabo, A. M. Cassard and H. Tilg, Recovery of ethanol-induced Akkermansia muciniphila depletion ameliorates alcoholic liver disease, Gut, 2018, 67, 891–901 CrossRef PubMed.
- T. Zhang, Q. Li, L. Cheng, H. Buch and F. Zhang,
Akkermansia muciniphila is a promising probiotic, Microb. Biotechnol., 2019, 12, 1109–1125 CrossRef PubMed.
- Y. Liang, C. Lin, Y. Zhang, Y. Deng, C. Liu and Q. Yang, Probiotic mixture of Lactobacillus and Bifidobacterium alleviates systemic adiposity and inflammation in non-alcoholic fatty liver disease rats through Gpr109a and the commensal metabolite butyrate, Inflammopharmacology, 2018, 26, 1051–1055 CrossRef CAS PubMed.
- A. Sivan, L. Corrales, N. Hubert, J. B. Williams, K. Aquino-Michaels, Z. M. Earley, F. W. Benyamin, Y. M. Lei, B. Jabri, M. L. Alegre, E. B. Chang and T. F. Gajewski, Commensal Bifidobacterium promotes antitumor immunity and facilitates anti-PD-L1 efficacy, Science, 2015, 350, 1084–1089 CrossRef CAS PubMed.
- Z. Lin, S. Ku, T. Lim, S. Y. Park, M. S. Park, G. E. Ji, K. O'Brien and K. T. Hwang, Antioxidant and anti-inflammatory properties of recombinant Bifidobacterium bifidum BGN4 expressing antioxidant enzymes, Microorganisms, 2021, 9, 595 CrossRef CAS PubMed.
- S. Banaszkiewicz, J. K. Calland, E. Mourkas, S. K. Sheppard, B. Pascoe and J. Bania, Genetic diversity of composite enterotoxigenic Staphylococcus epidermidis pathogenicity islands, Genome Biol. Evol., 2019, 11, 3498–3509 CrossRef CAS PubMed.
- Y. Momose, S. H. Park, Y. Miyamoto and K. Itoh, Design of species-specific oligonucleotide probes for the detection of Bacteroides and Parabacteroides by fluorescence in situ hybridization and their application to the analysis of mouse caecal Bacteroides-Parabacteroides microbiota, J. Appl. Microbiol., 2011, 111, 176–184 CrossRef CAS PubMed.
- A. Nakajima, T. Sasaki, K. Itoh, T. Kitahara, Y. Takema, K. Hiramatsu, D. Ishikawa, T. Shibuya, O. Kobayashi, T. Osada, S. Watanabe and A. Nagahara, A soluble fiber diet increases Bacteroides fragilis group abundance and immunoglobulin a production in the gut, Appl. Environ. Microbiol., 2020, 86, e00405–e00420 CrossRef CAS PubMed.
- K. Usami, K. Niimi, A. Matsuo, Y. Suyama, Y. Sakai, S. Sato, K. Fujihashi, H. Kiyono, S. Uchino, M. Furukawa, J. Islam, K. Ito, T. Moriya, Y. Kusumoto, M. Tomura, R. C. Hovey, J. Sugawara, H. Yoneyama, H. Kitazawa, K. Watanabe, H. Aso and T. Nochi, The gut microbiota induces Peyer's-patch-dependent secretion of maternal IgA into milk, Cell Rep., 2021, 36, 109655 CrossRef CAS PubMed.
- H. Nie, H. Ju, J. Fan, X. Shi, Y. Cheng, X. Cang, Z. Zheng, X. Duan and W. Yi, O-GlcNAcylation of PGK1 coordinates glycolysis and TCA cycle to promote tumor growth, Nat. Commun., 2020, 11, 36 CrossRef CAS PubMed.
- J. C. Gomes-Neto and J. L. Round, Gut microbiota: a new way to take your vitamins, Nat. Rev. Gastroenterol. Hepatol., 2018, 15, 521–522 CrossRef CAS PubMed.
- Z. He, Y. Ma, S. Yang, S. Zhang, S. Liu, J. Xiao, Y. Wang, W. Wang, H. Yang, S. Li and Z. Cao, Gut microbiota-derived ursodeoxycholic acid from neonatal dairy calves improves intestinal homeostasis and colitis to attenuate extended-spectrum β-lactamase-producing enteroaggregative Escherichia coli infection, Microbiome, 2022, 10, 79 CrossRef CAS PubMed.
- B. H. Mullish, J. A. K. McDonald, A. Pechlivanis, J. R. Allegretti, D. Kao, G. F. Barker, D. Kapila, E. O. Petrof, S. A. Joyce, C. G. M. Gahan, I. Glegola-Madejska, H. R. T. Williams, E. Holmes, T. B. Clarke, M. R. Thursz and J. R. Marchesi, Microbial bile salt hydrolases mediate the efficacy of faecal microbiota transplant in the treatment of recurrent Clostridioides difficile infection, Gut, 2019, 68, 1791–1800 CrossRef CAS PubMed.
- R. Mancusso, G. G. Gregorio, Q. Liu and D. N. Wang, Structure and mechanism of a bacterial sodium-dependent dicarboxylate transporter, Nature, 2012, 491, 622–626 CrossRef CAS PubMed.
- B. Gyanwali, Z. X. Lim, J. Soh, C. Lim, S. P. Guan, J. Goh, A. B. Maier and B. K. Kennedy, Alpha-Ketoglutarate dietary supplementation to improve health in humans, Trends Endocrinol. Metab., 2022, 33, 136–146 CrossRef CAS PubMed.
- L. L. Li, Y. T. Wang, J. Y. Yuan, Z. Y. Liu, C. Q. Ye and S. Qin,
Undaria pinnatifida improves obesity-related outcomes in association with gut microbiota and metabolomics modulation in high-fat diet-fed mice, Appl. Microbiol. Biotechnol., 2020, 104, 10217–10231 CrossRef CAS PubMed.
- Z. Zhai, J. Liu, K. M. Niu, C. Lin, Y. Tu, Y. Liu, L. Cai, H. Liu and K. Ouyang, Integrated metagenomics and metabolomics to reveal the effects of policosanol on modulating the gut microbiota and lipid metabolism in hyperlipidemic C57BL/6 mice, Front. Endocrinol., 2021, 12, 722055 CrossRef PubMed.
- M. M. Yore, I. Syed, P. M. Moraes-Vieira, T. Zhang, M. A. Herman, E. A. Homan, R. T. Patel, J. Lee, S. Chen, O. D. Peroni, A. S. Dhaneshwar, A. Hammarstedt, U. Smith, T. E. McGraw, A. Saghatelian and B. B. Kahn, Discovery of a class of endogenous mammalian lipids with anti-diabetic and anti-inflammatory effects, Cell, 2014, 159, 318–332 CrossRef CAS PubMed.
- M. Xing, Q. Cao, Y. Wang, H. Xiao, J. Zhao, Q. Zhang, A. Ji and S. Song, Advances in research on the bioactivity of alginate oligosaccharides, Mar. Drugs, 2020, 18, 144 CrossRef CAS PubMed.
- P. Zhang, J. Liu, B. Xiong, C. Zhang, B. Kang, Y. Gao, Z. Li, W. Ge, S. Cheng, Y. Hao, W. Shen, S. Yu, L. Chen, X. Tang, Y. Zhao and H. Zhang, Microbiota from alginate oligosaccharide-dosed mice successfully mitigated small intestinal mucositis, Microbiome, 2020, 8, 112 CrossRef CAS PubMed.
|
This journal is © The Royal Society of Chemistry 2023 |