DOI:
10.1039/D2FO02370A
(Paper)
Food Funct., 2023,
14, 445-456
Antigen presentation induced variation in ovalbumin sensitization between chicken and duck species
Received
12th August 2022
, Accepted 25th October 2022
First published on 13th December 2022
Abstract
The difference in the allergenicity of chicken ovalbumin (C-OVA) and duck ovalbumin (D-OVA) can be related to their differences in antigen presentation. This study explored the differences in uptake between C-OVA and D-OVA through fluorescence dye-labeling, DC antigen presentation, and the immune response of T cells by using C-OVA and D-OVA allergic animal and cell models. The ileum DCs of mice in the C-C group took up more C-OVA than that of D-D and C-D groups through in vivo imaging. Furthermore, C-OVA induced the maturation of DCs in mice in the C-C group as shown in the up-regulation of the expressions of MHC II, CD86 and CD80 on the surface of DCs, and enhanced the ability of antigen presentation. In addition, C-OVA induced the maturation of DCs, promoted the differentiation of T cells into Th2 cells, increased the secretion of the cytokine IL-4 and specific antibody s-IgE, and thus generated an immune response. However, sensitized and cross sensitized D-OVA (D-D and C-D groups) couldn't induce the maturation of DCs, and induced less differentiation of T cells and lower secretion of cytokines compared to C-OVA. In conclusion, the differences in antigen presentation was one of the important factors resulting in the differences in the sensitization between C-OVA and D-OVA.
1. Introduction
Chicken ovalbumin (C-OVA) is one of the most abundant allergenic proteins in chicken egg, which has resulted in severe allergy in people around the world. As a substitute of chicken egg, duck egg has also been widely consumed in China. However, the allergenicity of duck ovalbumin (D-OVA) is still unknown. Therefore, our previous study investigated the sensitization of D-OVA through an animal model and we found that D-OVA showed less sensitization than C-OVA through BALB/c mice.1
The differences in the sensitization between C-OVA and D-OVA can be caused by the amino acid sequence, post-translational modification, and structural differences between the two species that induce differences in antigen presentation.2–6 The amino acid sequences of C-OVA and D-OVA had 30% non-homology (data from the uniprot protein data framework: https://www.uniprot.org/). The 30% differences in the amino acids may result in the differences in both conformational and linear epitopes. A previous study showed that exchange of a single amino acid may lead to the disappearance of IgE binding or result in discernible of the general conformational pattern.7 Therefore, amino acid sequences could induce differences in the sensitization of C-OVA and D-OVA. Furthermore, LC-MS/MS analysis showed that C-OVA and D-OVA had 1 and 4 N-glycosides and 2 and 12 phosphorylation sites, respectively.8–11 Moreover, western blot studies have identified the differences between C-OVA and D-OVA, including the differences in electrophoretic mobility and unique epitopes.12
In addition, the entry of allergens into the immune system is critical for the development of allergy as the intestinal epithelial barrier plays an important role in controlling the translocation of different types of luminal antigens to maintain homeostasis and guide mucosal immune responses in tolerance and sensitization.13 However, the absorption mechanism of allergens and the regulation of allergen presentation are largely unknown. Visualization can help us further understand antigen uptake and presentation, and thus help us learn more about the occurrence of food allergies. Noah et al.14 have visualized the transfer of food allergens from the epithelial surface of the intestinal mucosa to the subepithelial immune compartment during food sensitization. The antigen delivery mechanism of lamina propria (LP)-DCs is proposed.
Intestinal luminal antigens are transported across the interstitial space to dendritic cells (DCs) by epithelial cells, presented to T cells and induce an immune response. Dendritic cells, known as “immune sentinels”, are the most efficient and professional antigen-presenting cells in the immune system, and are widely present in immune tissues and organs.15 When the gut encounters invading pathogens and antigens, immature dendritic cells (imDCs) derived from myeloid progenitor cells capture and process antigens, promote their surface co-stimulation and up-regulation of MHC molecules, produce inflammatory cytokines, and gradually mature.16 While migrating to the surrounding lymphoid organs, maturation of DCs is a prerequisite for the induction of adaptive immune responses. The maturation of DCs can promote the selective proliferation and differentiation of T cells into Th1, Th2 or Treg cells. That is, after the body is sensitized, mature dendritic cells (mDCs) will be recognized by T cell receptors through antigenic peptides that highly express MHC II and are loaded with food allergens on their surface. They highly express CD80 and CD86 costimulatory molecules on their surface to stimulate T cells to differentiate into Th2 cells. IL-4, IL-5, and IL-6 secreted by Th2 cells induce B lymphocytes to produce IgE antibodies, which ultimately trigger allergic reactions.17 While people mostly use DCs and T cells of normal mice to explore food allergens,3,6 we want to better simulate the immune cells after sensitization. Therefore, the DCs and T cells of mice after sensitization for 5 weeks were used for exploration.
Therefore, in order to explore the antigen presentation differences between C-OVA and D-OVA, the visible light volume imaging technology was applied to check the differences in the uptake of fluorescent dye-labeled C-OVA and D-OVA in the sensitized mice. Furthermore, DCs from bone marrow-derived dendritic cells (BMDCs) with C-OVA and D-OVA sensitized mice were isolated and cultured in vitro to explore the differences in the maturation and differentiation of DCs stimulated by C-OVA and D-OVA, respectively. In addition, high-purity naïve CD4+ T cells were isolated from mouse splenocytes by an immunomagnetic negative selection method, and co-cultured with antigen-induced DCs to explore the activation and differentiation of T cells stimulated by antigen-presenting DCs. The results in this study could provide evidence to better understand the differences in the antigen presentation between C-OVA and D-OVA.
2. Materials and methods
2.1. Materials
C-OVA was purchased from Sigma-Aldrich (A5503, Saint-Louis, USA). The fluorescent dye was obtained from PerkinElmer (VivoTag 680 XL, Waltham, USA). RPMI 1640 medium, recombinant mouse GM-CSF and IL-4 were purchased from Absin (Shanghai, China). Fetal bovine serum was obtained from Gibco (California, USA). The EasySep™ Mouse Naïve CD4+ T Cell Isolation Kit was obtained from Stemcell Technologies (Australia). Anti-mouse CD11c, Fixable Viability Stain 780, Ms CD11c BV421 N418, Ms I-A/I-E PE M5/114.15.2, Ms CD86 PE-Cy7 GL1, Ms CD80 APC 16-10A1, 7-AAD staining solution, Ms CD4 BV510 RM4-5, Ms IFN-Gma PE-Cy7 XMG1.2, Ms IL-4 PE 11B11, and Ms IL-4 PE 11B11 were purchased from BD Biosciences (New Jersey, USA). Elisa kits of IL-4 and IFN-γ were obtained from Mlbio (Shanghai, China).
2.2. Preparation of OVA from chicken and duck
C-OVA was purchased from Sigma. The preparation of duck ovalbumin (D-OVA) was according to a previous method.1 Briefly, the pH of the duck egg white was adjusted to 6.5, and NaCl was added to make the final concentration of 100 mmol L−1. Then 15% (w/w) PEG-8000 was added, and after stirring at 10 °C for 30 minutes (1500g), the solution was centrifuged at 10
000g for 15 minutes. The supernatant was taken and the pH was adjusted to 4.92. The solution was centrifuged at 10
000g for 15 min and the precipitate was collected. The above isoelectric precipitation operation was repeated after dissolving the precipitate in PBS. Distilled water was dialyzed for 48 h to remove PEG and salt ions, and the obtained D-OVA was stored at −20 °C for later use.
2.3. Murine allergy model
The mice were obtained from Charles River (Suzhou, Jiangsu, China) with the license number JN.No20210630b0750824[237]. All animal procedures were performed in accordance with the Guidelines for Care and Use of Laboratory Animals of Jiangnan University and experiments were approved by the Animal Ethics Committee of Jiangnan University. Three-week-old female BALB/c mice were randomly divided into 5 groups (n = 10): control group, Vibrio cholerae toxin (CT) adjuvant group (CT group), and three sensitized groups: C-OVA sensitized and C-OVA challenged group (C-C group, positive), D-OVA sensitized and D-OVA challenged group (D-D group, positive), and C-OVA sensitized and D-OVA challenged group (C-D cross group, cross). Sensitization and challenge were performed as before with slight modifications. As shown in Fig. 1, mice in the sensitized group were subjected to intragastric administration (i.g.) of 0.2 mL of PBS containing C-OVA or D-OVA (100 μg per g bw) and CT (0.5 μg per g bw) on day 0, day 7, day 14, day 21 and day 28. On day 35, mice were challenged by i.g. of 20 mg C-OVA or D-OVA. The CT group (0.5 μg per g bw CT in PBS) and naive mice (PBS) were used as controls. Serum protein-specific IgE (s-IgE) levels were determined by ELISA using the previous method.
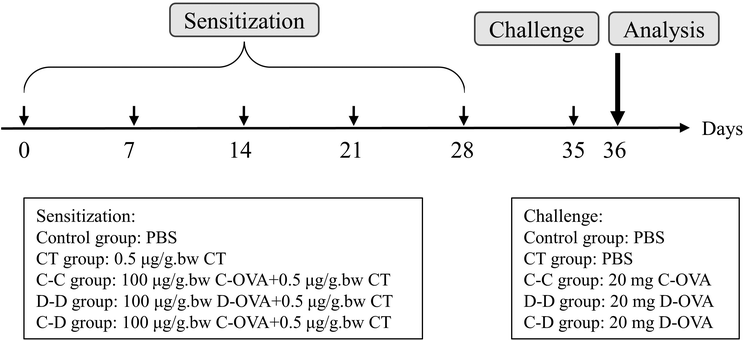 |
| Fig. 1 Animal experimental design for the food allergy model: control group, CT group, C-OVA sensitized and C-OVA challenged group (C-C group), D-OVA sensitized and D-OVA challenged group (D-D group), and the C-OVA sensitized and D-OVA challenged group (C-D group). | |
2.4. Fluorescent dye-labeled allergens
The concentration of C-OVA and D-OVA solution was determined using the BCA protein quantification kit and adjusted to 1 mg mL−1 in 50 mM carbonate/bicarbonate binding buffer (pH 8.5). 5 mg fluorescent dye (VivoTag 680 XL) was dissolved in 500 μL DMSO. 10 μL of dye per mL of protein solution was added. After mixing, the solution mixture was incubated at room temperature for 1 h in the dark and then centrifuged at 6000g for 20 min at 4 °C using a 10 kDa ultrafiltration centrifuge tube. The buffer was added and reconstitution was performed twice to remove the unreacted fluorescent dye from the mixture. The fluorescent dye-labeled allergen solution was stored in a refrigerator at 4 °C away from light.
2.5. Analysis of in vivo and in vitro imaging
The mice were challenged by i.g. of 20 mg protein on the 35th day. On the 36th day, the control group and CT group were given 0.5 mL of PBS by i.g., the control group and CT group were given 0.5 mL of fluorescent dye by i.g. to obtain the control-V group and CT-V group, the C-C group was given 0.5 mL of fluorescent dye-labeled C-OVA by i.g., and the D-D group and C-D group were given 0.5 mL of fluorescent dye-labeled D-OVA by i.g. In vivo imaging of mice was performed at 0 h, 1 h, 2 h, 3 h, and 4 h using an IVIS Lumina XRMS series III small animal imager (PerkinElmer, Waltham, USA). In vitro imaging of mouse gastrointestinal tract, kidneys, liver, spleen and mesenteric lymph nodes (MLNs) was performed at 4 hours with λex/em = 630/688 nm.
2.6. Analyses of immunological responses
Analyses of immunological responses were performed according to the method of Zhou et al.18 Briefly, the ileum that absorbed the fluorescent dye-labeled protein was sectioned with paraffin, incubated with the CD11c primary antibody at 4 °C for 2 h, evaluated by immunofluorescence staining and observed using a TCS SP8 confocal microscope (Leica, Solms, Germany).
2.7. Preparation of mouse BMDCs
2.7.1. Isolation and in vitro induction culture of BMDCs.
The preparation of mouse BMDCs was according to the method of Zheng et al.19 In brief, femur and tibia were isolated from BALB/c mice after sensitization on the 28th day, and bone marrow cells were obtained from the bone marrow cavity. The cells were added to RPMI 1640 complete medium (RPMI 1640 medium
:
fetal bovine serum
:
penicillin–streptomycin double antibody = 100
:
10
:
1) containing 20 ng mL−1 GM-CSF and 4 ng mL−1 IL-4. 106 cells per mL were cultured in 6-well plates for 6 days to obtain imDCs.
2.7.2. Stimulation of imDCs by antigenic proteins.
Different antigen proteins were added to the corresponding cells for stimulation after culturing for 6 days. For instance, 50 μg mL−1 of C-OVA antigen protein was added to the C-C group, 50 μg mL−1 of D-OVA antigen protein was added to the D-D group and the C-D group, and the cells were mixed by pipetting gently. Then, they were placed in an incubator to continue culturing for 24 h.
2.7.3. Detection of DC phagocytosis by flow cytometry.
The same concentration of fluorescent dye-labeled sensitized protein was added to the cells and incubated in the dark for 1 h. PBS was added to the control group and the CT group, the fluorescent dye was added to the control-V group and the CT-V group, C-OVA was added to the C-C group, and D-OVA was added to the D-D group and the C-D group. The cells were detected by the BD FACSArica II system (BD Biosciences, New Jersey, USA) and the data were analyzed using FlowJo software 10 (Tree Star, Inc., San Carlos, CA, USA).
2.7.4. Detection of DC maturation and differentiation by flow cytometry.
The DCs stimulated by antigen proteins were added with 1.5 μL of extracellular fluorescent conjugated antibodies CD11c-BV421, MHC II-PE, CD86-PE-Cy7, and CD80-APC, and incubated at room temperature for 20 min in the dark. After washing with PBS, 1.5 μL of 7-AAD staining solution was added and incubated at room temperature for 10 min in the dark. The cells were detected by the BD FACSArica II system.
2.7.5. Preparation of naïve CD4+ T cells and co-culture with DCs.
Naïve CD4+ T cells were isolated from the spleen of day 28 sensitized BALB/c mice using immunomagnetic negative selection according to the instructions of the EasySep™ Mouse Naïve CD4+ T Cell Isolation Kit. The cells were incubated overnight in 12-well plates. The sorted naïve CD4+ T cells and the DCs stimulated by antigen proteins were co-cultured in a 12-well plate at a ratio of 1
:
10 for 72 h.
2.7.6. Flow cytometry analysis.
The cells (106 mL−1) co-cultured with DCs were added with 1 μL stimulus-blocker for 4 h. Then the cells were washed with PBS and 1 μL Fixable Viability Stain 780 was added to stain dead and living cells. After washing with PBS, 1.5 μL of CD4-BV510 was added for extracellular staining. After fixation, 1.5 μL of IFN-γ-PE-Cy7 and IL-4-PE were added for intracellular staining. The cells were detected by the BD FACSArica II system.
2.7.7. Cytokine evaluation by ELISA.
The content of IL-4 and IFN-γ in culture supernatants was determined using a commercial ELISA kit, according to the manufacturer's instructions.
2.8. Statistical analysis
All the data were analyzed using SPSS 25.0 software. Means were compared among the groups using one-way analysis of variance (ANOVA) followed by a least significant difference (LSD) test. The p-values as follows were considered statistically significant: *p < 0.05, **p < 0.01, compared with the control group; # p < 0.01, ## p < 0.05, compared with the C-C group.
3. Results
3.1. The concentration of s-IgE challenged by C-OVA and D-OVA
Compared with the control group, the D-D group and the C-D group, serum s-IgE, a marker of allergic reactions, was significantly increased in the C-C group (p < 0.01) (Fig. 2).
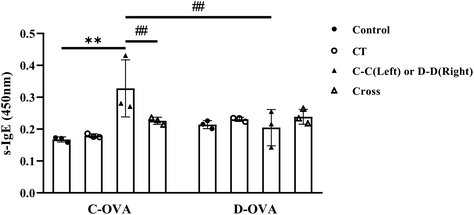 |
| Fig. 2 Serum levels of s-IgE in mice after challenge. *p < 0.05, **p < 0.01, compared with the control group; # p < 0.01, ## p < 0.05, compared with the C-C group. | |
3.2.
In vivo and in vitro imaging of uptake of C-OVA and D-OVA
The fluorescence monitoring process from 0 h to 4 h is shown in Fig. 3. In the control-V group and the CT-V group, some fluorescent dyes reached the small intestine at about 2 h. However, the fluorescent dye labeled allergens reached the intestinal tract at about 1 h in the C-C, D-D and C-D groups, and the fluorescence signal gradually decreased in the small intestinal tract of mice with the increase of time.
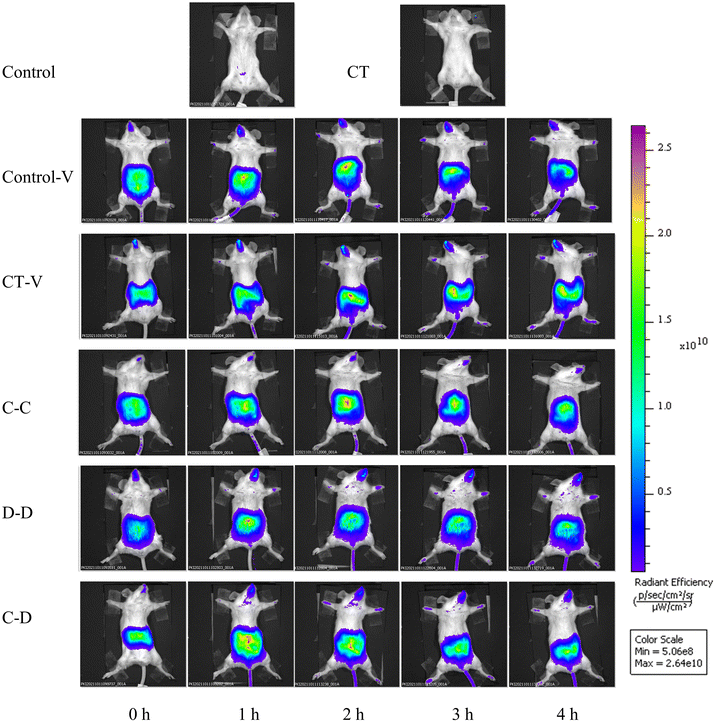 |
| Fig. 3
In vivo images of fluorescence signals accumulated from 0 h to 4 h after intragastric administration of C-OVA and D-OVA. *p < 0.05, **p < 0.01, compared with the control group; # p < 0.01, ## p < 0.05, compared with the C-C group. | |
The gastrointestinal tract, kidneys, liver, spleen and MLNs were completely removed from the mice for in vitro imaging 4 h after i.g. The results are shown in Fig. 4(a), the fluorescent dye in each group had passed through the stomach and jejunum, mainly located in the ileum and reached the cecum. Fig. 4(b) shows that the kidneys and MLNs in the C-C, D-D and C-D groups all showed obvious fluorescent signals. The livers of the C-D group also showed obvious fluorescence signals. Therefore, the relative quantitative analysis of the fluorescence signal intensity of each organ was further carried out.
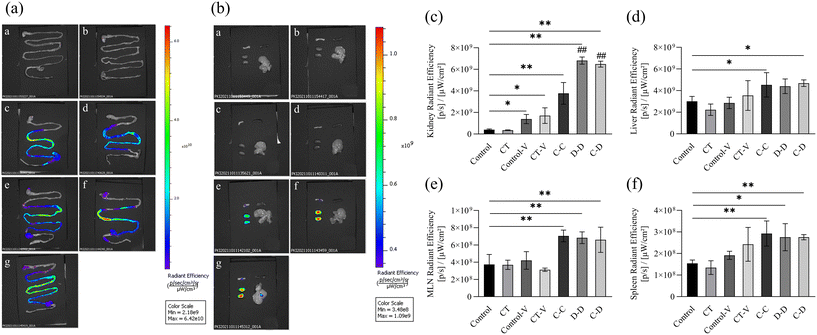 |
| Fig. 4
In vitro images of fluorescence signals of various organs accumulated from 0 h to 4 h after intragastric administration of C-OVA and D-OVA. (a) Fluorescence imaging of the small intestine; (b) fluorescence imaging of mesenteric lymph nodes (upper left), spleen (upper right), kidneys (lower left), and the liver (lower right); (c) relative quantification of the fluorescence intensity of the kidneys; (d) relative quantification of the fluorescence intensity of the liver; (e) relative quantification of the fluorescence intensity of the mesenteric lymph nodes; (f) relative quantification of the fluorescence intensity of the spleen. (a) and (b): a: control group; b: CT group; c: control-V group; d: CT-V group; e: C-C group; f: D-D group; g: C-D group. *p < 0.05, **p < 0.01, compared with the control group; # p < 0.01, ## p < 0.05, compared with the C-C group. | |
Next, we explored the relative quantitative analysis of the fluorescence signal intensity of each organ. The fluorescence signals of the kidneys in the C-C, D-D and C-D groups were significantly increased (p < 0.01) compared to that of the control group (Fig. 4(c)), and among the C-C, D-D and C-D groups, the fluorescence signals of the D-D and C-D groups were significantly higher than that of the C-C group (p < 0.01). The fluorescence signals of the liver in the C-C and C-D groups were significantly higher than those in the control group (p < 0.05) (Fig. 4(d)). However, there was no significant difference in the liver fluorescence signals between the experimental groups C-C, D-D and C-D (p > 0.05). The signals of MLNs and the spleen were all significantly higher in the C-C, D-D and C-D groups than those of the control group (p < 0.01), of which the signals of the spleen in the D-D group had smaller magnitudes (p < 0.05) (Fig. 4(e) and (f)). There was no significant difference in the fluorescence signals of MLNs and the spleen between the experimental groups C-C, D-D and C-D (p > 0.05).
3.3. Uptake of C-OVA and D-OVA by DCs in vivo and in vitro
In order to explore the uptake of allergen proteins by professional antigen-presenting cells DCs, small intestinal DCs and allergens were co-located by immunofluorescence 4 h after i.g. The results showed that a large amount of fluorescent dye-labeled C-OVA in the C-C group was taken up by DCs in the LP, whereas part of the fluorescent dye-labeled D-OVA in the D-D and C-D groups was taken up by DCs in the LP, and the uptake was like that of some pure fluorescent dyes in the control-V and CT-V groups (Fig. 5).
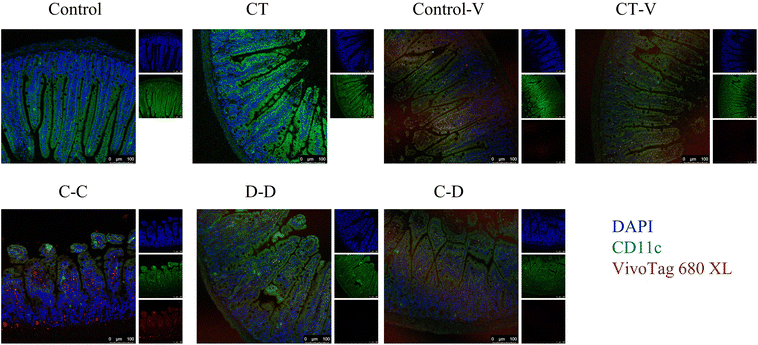 |
| Fig. 5 Fluorescence co-localization of DCs, and absorbed C-OVA and D-OVA in the ileum of mice. Blue fluorescence indicates cell nuclei, green fluorescence indicates the CD11c marker on the surface of DCs, and red fluorescence indicates the fluorescent dye-labeled protein. | |
In this study, myeloid stem cells were extracted from bone marrow and introduced into imDCs by combination of GM-CSF and IL-4 in vitro. In vitro cell experiments were conducted to explore whether mice sensitized by different allergens showed specific phagocytosis to the corresponding allergens. As shown in Fig. 6, 25.90% of DCs in the C-C group phagocytosed C-OVA, which was significantly higher than 17.45% of DCs in the control-V group that phagocytosed the fluorescent dye (p < 0.05), while the C-C group (25.90%), the D-D group (21.70%) and the C-D group (25.35%) had no significant difference (p > 0.05).
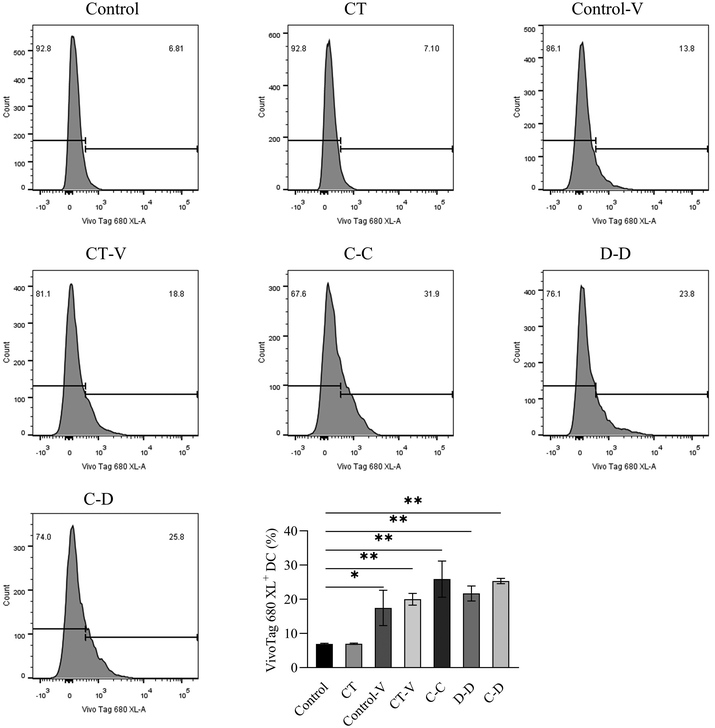 |
| Fig. 6 The differences in DC uptake and phagocytosis between C-OVA and D-OVA. *p < 0.05, **p < 0.01, compared with the control group; # p < 0.01, ## p < 0.05, compared with the C-C group. | |
3.4. Maturation and differentiation of BMDCs by C-OVA and D-OVA
In this study, C-OVA was used to stimulate DCs from C-OVA-sensitized mice, D-OVA to stimulate DCs from D-OVA-sensitized mice, and D-OVA to stimulate DCs from C-OVA-sensitized mice. As shown in Fig. 7, compared with the control group, the D-D group and the C-D group, C-OVA in the C-C group can significantly up-regulate the class II major histocompatibility complex (MHC II) and the expression of costimulatory molecules (CD86 and CD80) in the DCs of C-OVA-sensitized mice (p < 0.01). The proportion of CD11c+ MHC II+ DCs was 21.00%, the proportion of CD11c+ CD86+ DCs was 6.99%, and the proportion of CD11c+ CD80+ DCs was 10.20%. Compared with the control group, the expressions of MHC II, CD86 and CD80 on the surface of the DCs of D-OVA-sensitized mice and C-OVA-sensitized mice were not up-regulated by D-OVA in the D-D and C-D groups (p > 0.05). The proportions of CD11c+ MHC II+ DCs in the D-D and C-D groups were 8.61% and 9.05%, the proportions of CD11c+ CD86+ DCs were 2.48% and 2.57%, and the proportions of CD11c+ CD80+ DCs were 3.14% and 3.67%, respectively.
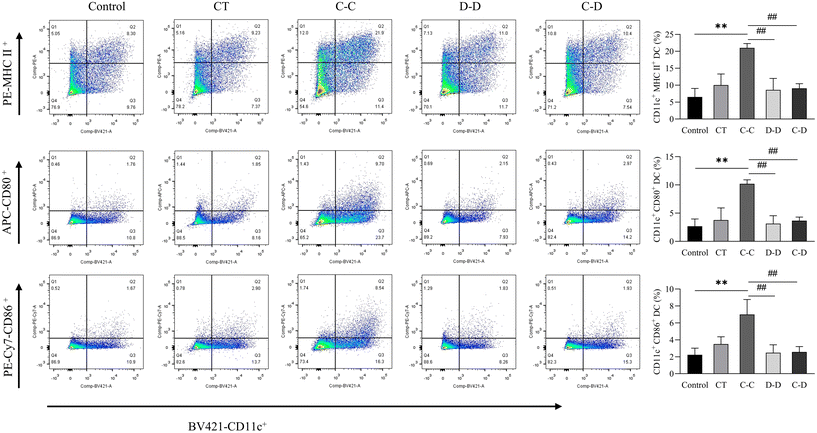 |
| Fig. 7 C-OVA and D-OVA induced differences in DC maturation and differentiation. *p < 0.05, **p < 0.01, compared with the control group; # p < 0.01, ## p < 0.05, compared with the C-C group. | |
3.5. Differentiation of T cells after co-culture
In this study, high-purity naïve CD4+ T cells were isolated from mouse splenocytes by immunomagnetic negative selection and co-cultured with DCs induced by C-OVA and D-OVA. The activation and differentiation of T cells by mDCs induced by C-OVA and immature DCs induced by D-OVA were verified. The results are shown in Fig. 8(a), where naïve CD4+ T cells were highly differentiated into CD4+ IL-4+ Th2 cells in the C-C group. The proportion of CD4+ IL-4+ Th2 cells was 13.13%, which was significantly higher than that in the control group (1.53%) (p < 0.01). The proportions of CD4+ IL-4+ Th2 cells in the D-D and C-D groups were 5.13% and 2.73%, respectively, which were significantly lower than those in the C-C group (p < 0.01). Fig. 8(b) shows that compared with the control group, the naïve CD4+ T cells in the C-C, D-D and C-D groups were not differentiated into CD4+ IFN-γ+ Th1 cells. The proportions of CD4+ IFN-γ+ Th1 cells in the control group, the C-C group, the D-D group and the C-D group were 0.38%, 0.78%, 0.43% and 0.52%, respectively (p > 0.05).
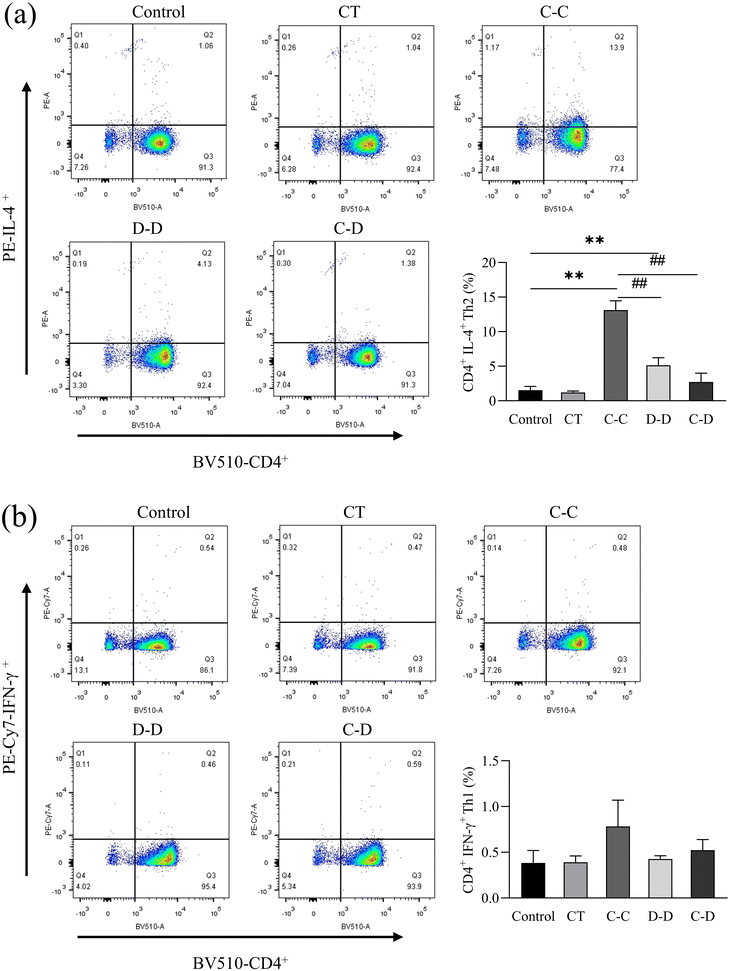 |
| Fig. 8 C-OVA and D-OVA induced differences in the differentiation of naïve CD4+ T cells into Th2 cells (a) and Th1 cells (b) after incubation with DCs. *p < 0.05, **p < 0.01, compared with the control group; # p < 0.01, ## p < 0.05, compared with the C-C group. | |
IL-4 is a cytokine produced by Th2 cells. IFN-γ is a cytokine produced by Th1 cells. The results were consistent with the flow cytometry results. As shown in Fig. 9(a), compared with the control, D-D and C-D groups, the content of IL-4 in the C-C group was significantly increased (p < 0.01). However, there was no significant difference between the D-D and C-D groups and the control group (p > 0.05). As shown in Fig. 9(b), the content of IFN-γ had no significant difference among all groups (p > 0.05).
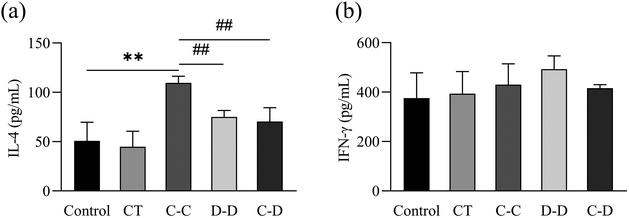 |
| Fig. 9 C-OVA and D-OVA induced differences in the expression of the cytokine levels of IL-4 (a) and IFN-γ (b) from Th2 and Th1 cells, respectively. *p < 0.05, **p < 0.01, compared with the control group; # p < 0.01, ## p < 0.05, compared with the C-C group. | |
4. Discussion
In order to explore the effect of antigen presentation on the differences in the sensitization between C-OVA and D-OVA, visible light volume imaging was used to detect the differences in the uptake of fluorescent dye-labeled C-OVA and D-OVA in sensitized mice. In addition, C-OVA and D-OVA stimulated the maturation and differentiation of DCs, and further activation and differentiation of T cells were investigated using DCs and T cells extracted from sensitized mice.
The difference in the allergic immune response may be due to the difference in the structure and digestibility of C-OVA and D-OVA. Most allergens are believed to be generally resistant to degradation by gastrointestinal proteases due to their structural stability20 and thus contribute to the induction of food allergies.21 When food enters the stomach, pepsin preferentially cleaves protein amino acid (AA) chains at phenylalanine, tyrosine and leucine residues after being activated in acidic pH,22 allowing allergens to cause some degree of denaturation and hydrolysis. In the duodenum, proteins are further broken down under the action of pancreatic enzymes and their allergen levels are altered.23 Astwood et al.24 suggested that allergens are either resistant to gastrointestinal enzymes or produce stable fragments in the intestine, whereas non-allergens are completely digested. Non-allergens produce peptide fragments in the stomach. Intestinal peptide fragments are further degraded into short AA chains or single AA, which serve as nutrients for the human body. However, they are too small to interact with the human immune system.25 According to Moreno et al.,26 OVA is partially resistant to pepsin and gastrointestinal digestion. Intact OVA was still detectable after 120 min exposure to simulated gastric conditions.27 Duodenal digestion had a greater effect on OVA hydrolysis than gastric digestion, and the IgE-binding activity of OVA was significantly reduced after gastrointestinal digestion, but some of its immunoreactive properties were preserved due to the intact epitope.28,29 It is reported that OVA retained 30% of its IgE-binding activity after duodenal digestion.30 Benedé et al.31 used human gastrointestinal fluid to digest OVA, and the resulting peptides were studied for their immunoreactivity levels in human serum, and 8 high-frequency IgE binding epitopes were identified.
Under normal circumstances, a protein is completely degraded into amino acids and dipeptides, which enter the liver through portal circulation for catabolism, and pass through the kidneys, the detoxification organ, to excrete wastes that cannot be digested and absorbed in the metabolic process through urine.32 The fluorescence intensity of the kidneys in the D-D and C-D groups was significantly higher than that in the C-C group (Fig. 4(c)). It is possible that more D-OVA was completely degraded by the mice and then absorbed through the circulation to reach the kidneys. While studies have shown that approximately 15% of incompletely digested proteins, including food allergens,33 are transported by microcystis cells (M cells), transepithelial dendritic cells (TEDs), and goblet cells through paracellular diffusion across the intestinal epithelium and are taken up by APCs in the LP.34–38 APCs migrate from the LP to lymphatic tissues such as MLNs and the spleen through lymphatic vessels, and present the loaded antigens to lymphocytes.39–41 Our results show that C-OVA and D-OVA allergens can enter the relevant immune system through the spleen and mesenteric lymph nodes.
DCs are specialized APCs with the strongest antigen-presenting capacity. Therefore, we explored the uptake of C-OVA and D-OVA by DCs in vitro and in vivo. There is no difference in the uptake of C-OVA and D-OVA by DCs in vitro (Fig. 6), while the ileal DCs of mice in the C-C group take up a large number of C-OVA (Fig. 5). It is speculated that it may be caused by the differences in the immune environment in vivo. On the one hand, DCs can perform luminal antigen sampling by forming transepithelial dendrites (TEDs).42 On the other hand, they can transmit antigens to LP-DCs by inducing goblet cell secretion and increasing the formation of antigen channels, forming an antigen delivery mechanism. At the same time, Esterhazy et al.43 showed that the proximal intestinal draining lymph nodes preferentially develop tolerance and the distal intestine generates a pro-inflammatory T cell response. Our results showed that the massive intake of C-OVA by DCs in the ileum induces a pro-inflammatory T cell response.
DCs process antigens and present them to T cells in lymphoid tissues, and thus initiate the corresponding immune response.41 Furthermore, they also regulate immune responses through signaling molecular pathways and play a key role in the sensitization mechanism. Antigens could induce the maturation of DCs through changing the expression of DCs’ surface molecules, which regulates the downstream immune response. The high expression of MHC II, CD86 and CD80 in the C-C group indicated the maturation of DCs (Fig. 7), and mDCs have strong ability to present antigens and stimulate and activate T cells. This is because the antigen captured by DCs can induce their maturation and up-regulate the expression of MHC II on the plasma membrane. The loading of MHC II with antigenic peptides is the first signal of T cell activation.44–46 At the same time, mDCs migrate to lymphoid organs and upregulate the costimulatory molecules CD80 and CD86, which are the second signals of T cell activation.47 This study verified that C-OVA induced the maturation of DCs in C-OVA-sensitized mice, and mDCs could induce a large number of differentiations of naïve CD4+ T cells into Th2 lymphocytes (Fig. 8). D-OVA failed to stimulate the maturation of DCs in D-OVA and C-OVA-sensitized mice, and the expressions of surface MHC II, CD86 and CD80 were not up-regulated. Therefore, in the absence of antigenic peptides and co-stimulation in the D-D and C-D groups, naïve CD4+ T cells were unresponsive48 and did not differentiate into Th2-type lymphocytes. The overproduction and activation of Th2 lymphocytes play an important control and regulation role in allergic inflammation. Therefore, the activated Th2 cells in the C-C group can secrete a large amount of IL-4, IL-5, IL-6, etc. to induce the transformation of B lymphocytes into plasma cells, produce IgE antibodies, and cause a series of downstream allergic reactions and immune responses.16,17 Therefore, T cells in the C-C group were activated by DCs, differentiated into Th2 cells, secreted a large amount of cytokine IL-4 (Fig. 9), induced B cells to proliferate and differentiate into plasma cells, and produced s-IgE antibodies (Fig. 2), and then produced allergic reactions. However, T cells in the D-D and C-D groups were less activated than those in the C-C group as no significant changes were found in the expressions of IL-4 and s-IgE antibody. Further studies on the intestinal environment and body regulation induced variation in the uptake and antigen presentation by intestinal epithelial-related cells and immune cells can be conducted to better understand the mechanism of the differences in antigen presentation between C-OVA and D-OVA.
5. Conclusion
Our results showed that the intestinal DCs of the mice in the C-C group absorbed a large number of C-OVA, while the intestinal DCs of the mice in the D-D and C-D groups absorbed less D-OVA. C-OVA induced DC maturation, enhanced the antigen presentation ability, and promoted Th2 cell differentiation and immune response in the mice in the C-C group, while D-OVA did not contribute to the maturation of DCs in the D-D group and C-D group mice. Therefore, the differences in presentation between C-OVA and D-OVA were one of the key factors for the differences in their sensitization.
Author contributions
Lina Zhang: conceptualization, validation, writing – original draft, writing – review & editing, and project administration. Ruoya Zhou: methodology and writing – original draft. Kai Zhang: investigation, methodology, and visualization. Peng Zhou: supervision, writing – review & editing, and project administration.
Conflicts of interest
The authors declare that they have no conflict of interest.
Acknowledgements
This work was supported by the State Key Research and Development Plan (No. 2019YFC1605002).
References
- R. Y. Zhou, L. N. Zhang, K. Zhang and P. Zhou, Difference of egg ovalbumin sensitization between egg and duck eggs in BALB/c mice, Eur. Food Res. Technol., 2022, 248, 1035–1048 CrossRef.
- T. Hilmenyuk, I. Bellinghausen, B. Heydenreich, A. Ilchmann, M. Toda, S. Grabbe and J. Saloga, Effects of glycation of the model food allergen ovalbumin on antigen uptake and presentation by human dendritic cells, Immunology, 2010, 129, 437–445 CrossRef CAS PubMed.
- A. Ilchmann, S. Burgdorf, S. Scheurer, Z. Waibler, R. Nagai, A. Wellner, Y. Yamamoto, H. Yamamoto, T. Henle, C. Kurts, U. Kalinke, S. Vieths and M. Toda, Glycation of a food allergen by the Maillard reaction enhances its T-cell immunogenicity: Role of macrophage scavenger receptor class A type I and II, J. Allergy Clin. Immunol., 2010, 125, 175–183 CrossRef CAS PubMed.
- M. Perusko, M. van Roest, D. Stanic-Vucinic, P. J. Simons, R. H. H. Pieters, T. C. Velickovic and J. J. Smit, Glycation of the Major Milk Allergen beta-Lactoglobulin Changes Its Allergenicity by Alterations in Cellular Uptake and Degradation, Mol. Nutr. Food Res., 2018, 62, e1800341 CrossRef PubMed.
- M. Stojadinovic, R. Pieters, J. Smit and T. C. Velickovic, Cross-linking of beta-lactoglobulin enhances allergic sensitization through changes in cellular uptake and processing, Toxicol. Sci., 2014, 140, 224–235 CrossRef CAS PubMed.
- C. Villemin, O. Tranquet, V. Sole-Jamault, J. J. Smit, R. H. H. Pieters, S. Denery-Papini and G. Bouchaud, Deamidation and Enzymatic Hydrolysis of Gliadins Alter Their Processing by Dendritic Cells in Vitro, J. Agric. Food Chem., 2020, 68, 1447–1456 CrossRef CAS PubMed.
- R. Kapila, P. K. Kavadi and S. Kapila, Comparative evaluation of allergic sensitization to milk proteins of cow, buffalo and goat, Small Rumin. Res., 2013, 112, 191–198 CrossRef.
- J. A. Huntington and P. E. Stein, Structure and properties of ovalbumin, J. Chromatogr. B: Biomed. Sci. Appl., 2001, 756, 189–198 CrossRef CAS PubMed.
- F. Geng, J. Wang, D. Liu, Y. Jin and M. Ma, Identification of N-glycosites in chicken egg white proteins using an omics strategy, J. Agric. Food Chem., 2017, 65, 5357–5364 CrossRef CAS PubMed.
- Y. Q. Meng, N. Qiu, F. Geng, Y. Q. Huo, H. H. Sun and R. Keast, Identification of the duck egg white N-glycoproteome and insight into the course of biological evolution, J. Agric. Food Chem., 2019, 67, 9950–9957 CrossRef CAS PubMed.
- A. Rida, M. Yaqi, Q. Ning, S. Haohao, R. Keast and R. Abdur, Phosphoproteomic analysis of duck egg white and insight into the biological functions of identified phosphoproteins, J. Food Biochem., 2020, 44, e13367–e13367 Search PubMed.
- M. Alcántara Villar, L. Palacios Colom, S. Anaya Anaya, L. Bustamante Orvay and L. Jimeno Nogales, Duck Egg Allergy in an Adult Patient Without Allergy to Chicken Egg, J. Invest. Allergol. Clin. Immunol., 2019, 29, 245–246 CrossRef PubMed.
- R. Farré, M. Fiorani, S. A. Rahiman and G. Matteoli, Intestinal Permeability, Inflammation and the Role of Nutrients, Nutrients., 2020, 12, 1185 CrossRef PubMed.
- T. K. Noah, K. A. Knoop, K. G. McDonald, J. K. Gustafsson, L. Waggoner, S. Vanoni, M. Batie, K. Arora, A. P. Naren, Y.-H. Wang, N. W. Lukacs, A. Munitz, M. A. Helmrath, M. M. Mahe, R. D. Newberry and S. P. Hogan, IL-13-induced intestinal secretory epithelial cell antigen passages are required for IgE-mediated food-induced anaphylaxis, J. Allergy Clin. Immunol., 2019, 144, 1058–1073 CrossRef CAS PubMed.
- M. L. Albert, B. Sauter and N. Bhardwaj, Dendritic cells acquire antigen from apoptotic cells and induce class I restricted CTLs, Nature, 1998, 392, 86–89 CrossRef CAS PubMed.
- M. N. Rivas, O. T. Burton, P. Wise, L.-M. Charbonnier, P. Georgiev, H. C. Oettgen, R. Rachid and T. A. Chatila, Regulatory T cell reprogramming toward a Th2-cell-like lineage impairs oral tolerance and promotes food allergy, Immunity, 2015, 42, 512–523 CrossRef PubMed.
- S. Jiang, S. Han, J. Chen, X. Li and H. Che, Inhibition effect of blunting Notch signaling on food allergy through improving Th1/Th2 balance in mice, Ann. Allergy, Asthma, Immunol., 2017, 118, 94–102 CrossRef CAS PubMed.
- Y. Zhou, H. Kawasaki, S.-C. Hsu, R. T. Lee, X. Yao, B. Plunkett, J. Fu, K. Yang, Y. C. Lee and S.-K. Huang, Oral tolerance to food-induced systemic anaphylaxis mediated by the C-type lectin SIGNR1, Nat. Med., 2010, 16, 1128–U1105 CrossRef CAS PubMed.
- J. Zheng, H. Y. Jiang, J. Li, H. C. Tang, X. M. Zhang, X. R. Wang, J. T. Du, H. B. Li and G. Xu, MicroRNA-23b promotes tolerogenic properties of dendritic cells in vitro through inhibiting Notch1/NF-κB signalling pathways, Allergy, 2012, 67, 362–370 CrossRef CAS PubMed.
- E. N. C. Mills, J. A. Jenkins and P. R. Shewry, The Role of Common Properties in Determining Plant Food Protein Allergenicity, Plant Food Allergens, 2003, 1, 158–170 Search PubMed.
- A.-R. Lorenz, S. Scheurer and S. Vieths, Food allergens: molecular and immunological aspects, allergen databases and cross-reactivity, Chem. Immunol. Allergy, 2015, 101, 18–29 CAS.
- E. Untersmayr and E. Jensen-Jarolim, The role of protein digestibility and antacids on food allergy outcomes, J. Allergy Clin. Immunol., 2008, 121, 1301–1308 CrossRef PubMed.
- J. Keller and P. Layer, Human pancreatic exocrine response to nutrients in health and disease, Gut, 2005, 54, 1–28 CrossRef PubMed.
- J. D. Astwood, J. N. Leach and R. L. Fuchs, Stability of food allergens to digestion in vitro, Nat. Biotechnol., 1996, 14, 1269–1273 CrossRef CAS PubMed.
- I. Pali-Scholl and E. Jensen-Jarolim, Anti-acid medication as a risk factor for food allergy, Allergy, 2011, 66, 469–477 CrossRef CAS PubMed.
- F. J. Moreno, Gastrointestinal digestion of food allergens: Effect on their allergenicity, Biomed. Pharmacother., 2007, 61, 50–60 CrossRef CAS PubMed.
- G. Martos, P. Contreras, E. Molina and R. López-FandiÑo, Egg White Ovalbumin Digestion Mimicking Physiological Conditions, J. Agric. Food Chem., 2010, 58, 5640–5648 CrossRef CAS PubMed.
- K. Takagi, R. Teshima, H. Okunuki and J.-I. Sawada, Comparative study of in vitro digestibility of food proteins and effect of preheating on the digestion, Biol. Pharm. Bull., 2003, 26, 969–973 CrossRef CAS PubMed.
- K. Honma, Y. Kohno, K. Saito, N. Shimojo, T. Horiuchi, H. Hayashi, N. Suzuki, T. Hosoya, H. Tsunoo and H. Niimi, Allergenic epitopes of ovalbumin (OVA) in patients with hen's egg allergy: inhibition of basophil histamine release by haptenic ovalbumin peptide, Clin. Exp. Immunol., 1996, 103, 446–453 CrossRef CAS PubMed.
- R. Jimenez-Saiz, J. Belloque, E. Molina and R. Lopez-Fandino, Human immunoglobulin E (IgE) binding to heated and glycated ovalbumin and ovomucoid before and after in vitro digestion, J. Agric. Food Chem., 2011, 59, 10044–10051 CrossRef CAS PubMed.
- S. Benedé, I. Lopez-Exposito, R. Lopez-Fandino and E. Molina, Identification of IgE-Binding Peptides in Hen Egg Ovalbumin Digested in Vitro with Human and Simulated Gastroduodenal Fluids, J. Agric. Food Chem., 2014, 62, 152–158 CrossRef PubMed.
- K. M. Knights, A. Rowland and J. O. Miners, Renal drug metabolism in humans: the potential for drug-endobiotic interactions involving cytochrome P450 (CYP) and UDP-glucuronosyltransferase
(UGT), Br. J. Clin. Pharmacol., 2013, 76, 587–602 CAS.
- R. S. Chinthrajah, J. D. Hernandez, S. D. Boyd, S. J. Galli and K. C. Nadeau, Molecular and cellular mechanisms of food allergy and food tolerance, J. Allergy Clin. Immunol., 2016, 137, 984–997 CrossRef CAS PubMed.
- K. Hase, K. Kawano, T. Nochi, G. S. Pontes, S. Fukuda, M. Ebisawa, K. Kadokura, T. Tobe, Y. Fujimura, S. Kawano, A. Yabashi, S. Waguri, G. Nakato, S. Kimura, T. Murakami, M. Iimura, K. Hamura, S. I. Fukuoka, A. W. Lowe, K. Itoh, H. Kiyono and H. Ohno, Uptake through glycoprotein 2 of FimH(+) bacteria by M cells initiates mucosal immune response, Nature, 2009, 462, 226–U101 CrossRef CAS PubMed.
- P. C. Tyrer, A. R. Foxwell, J. M. Kyd, D. C. Otczyk and A. W. Cripps, Receptor mediated targeting of M-cells, Vaccine, 2007, 25, 3204–3209 CrossRef CAS PubMed.
- J. R. Turner, Intestinal mucosal barrier function in health and disease, Nat. Rev. Immunol., 2009, 9, 799–809 CrossRef CAS PubMed.
- J. R. McDole, L. W. Wheeler, K. G. McDonald, B. Wang, V. Konjufca, K. A. Knoop, R. D. Newberry and M. J. Miller, Goblet cells deliver luminal antigen to CD103(+) dendritic cells in the small intestine, Nature, 2012, 483, 345–U141 CrossRef CAS PubMed.
- M. R. Neutra, A. Frey and J. P. Kraehenbuhl, Epithelial M cells: Gateways for mucosal infection and immunization, Cell, 1996, 86, 345–348 CrossRef CAS PubMed.
- E. Mazzini, L. Massimiliano, G. Penna and M. Rescigno, Oral tolerance can be established via gap junction transfer of fed antigens from CX3CR1(+) macrophages to CD103(+) dendritic cells, Immunity, 2014, 40, 248–261 CrossRef CAS PubMed.
- S. Milling, U. Yrlid, V. Cerovic and G. MacPherson, Subsets of migrating intestinal dendritic cells, Immunol. Rev., 2010, 234, 259–267 CrossRef CAS PubMed.
- T. Worbs, U. Bode, S. Yan, M. W. Hoffmann, G. Hintzen, G. Bernhardt, R. Forster and O. Pabst, Oral tolerance originates in the intestinal immune system and relies on antigen carriage by dendritic cells, J. Exp. Med., 2006, 203, 519–527 CrossRef CAS PubMed.
- K. A. Knoop, K. G. McDonald, S. McCrate, J. R. McDole and R. D. Newberry, Microbial sensing by goblet cells controls immune surveillance of luminal antigens in the colon, Mucosal Immunol., 2015, 8, 198–210 CrossRef CAS PubMed.
- D. Esterhazy, M. C. C. Canesso, L. Mesin, P. A. Muller, T. B. R. de Castro, A. Lockhart, M. ElJalby, A. M. C. Faria and D. Mucida, Compartmentalized gut lymph node drainage dictates adaptive immune responses, Nature, 2019, 569, 126–130 CrossRef CAS PubMed.
- M. Cella, A. Engering, V. Pinet, J. Pieters and A. Lanzavecchia, Inflammatory stimuli induce accumulation of MHC class II complexes on dendritic cells, Nature, 1997, 388, 782–787 CrossRef CAS PubMed.
- K. Inaba, S. Turley, T. Iyoda, F. Yamaide, S. Shimoyama, C. R. E. Sousa, R. N. Germain, I. Mellman and R. M. Steinman, The formation of immunogenic major histocompatibility complex class II-peptide ligands in lysosomal compartments of dendritic cells is regulated by inflammatory stimuli, J. Exp. Med., 2000, 191, 927–936 CrossRef CAS PubMed.
- S. J. Turley, K. Inaba, W. S. Garrett, M. Ebersold, J. Unternaehrer, R. M. Steinman and I. Mellman, Transport of peptide-MHC class II complexes in developing dendritic cells, Science, 2000, 288, 522–527 CrossRef CAS PubMed.
- D. J. Lenschow, T. L. Walunas and J. A. Bluestone, CD28/B7 system of T cell costimulation, Annu. Rev. Immunol., 1996, 14, 233–258 CrossRef CAS PubMed.
- R. H. Schwartz, A cell culture model for T lymphocyte clonal anergy, Science, 1990, 248, 1349–1356 CrossRef CAS PubMed.
|
This journal is © The Royal Society of Chemistry 2023 |
Click here to see how this site uses Cookies. View our privacy policy here.