DOI:
10.1039/D2FO02225G
(Paper)
Food Funct., 2023,
14, 413-426
Mechanistic study of salidroside on ovalbumin-induced asthmatic model mice based on untargeted metabolomics analysis†
Received
29th July 2022
, Accepted 17th November 2022
First published on 18th November 2022
Abstract
Salidroside (SAL) is a natural component derived from Rhodiola rosea and is well known for its wide range of biological activities such as its anti-inflammatory and anti-oxidative properties. However, its effects and mechanisms of action related to asthma have not been well explored yet. Recent studies have found that changes in host metabolism are closely related to the progression of asthma. Many natural components can ameliorate asthma by affecting host metabolism. The use of untargeted metabolomics can allow for a better understanding of the metabolic regulatory mechanisms of herbs on asthma. This study aimed to demonstrate the anti-asthmatic effects and metabolic regulatory mechanisms of SAL. In this study, the therapeutic effects of SAL on asthmatic mice were tested at first. Secondly, the effects of SAL on the airway inflammatory reaction, oxidative stress, and airway remodeling were investigated. Finally, untargeted metabolomics analysis was used to explore the influence of SAL on lung metabolites. The results showed that SAL had a significant therapeutic effect on asthmatic model mice. Moreover, SAL treatment lowered interleukin (IL)-4, IL-5, and IL-13 levels but elevated interferon gamma (IFN-γ) and IL-10 levels in bronchoalveolar lavage fluid (BALF). Additionally, it also increased superoxide dismutase (SOD) and glutathione peroxidase (GSH-Px) activities and decreased methane dicarboxylic aldehyde (MDA) levels in the lungs. Besides, SAL-treated mice showed decreased expression of smooth muscle actin (α-SMA), matrix metallopeptidase 2 (MMP2), matrix metallopeptidase 9 (MMP9), and transforming growth factor-beta 1 (TGF-β1) in the lung. Untargeted metabolomics analysis showed 31 metabolites in the lungs that were influenced by SAL. These metabolites were related to pyrimidine metabolism, steroid hormone biosynthesis, and tricarboxylic acid (TCA) cycle. In conclusion, SAL treatment can reduce the inflammatory response, oxidative stress, and airway remodeling in asthmatic model mice. The mechanism of SAL in the treatment of asthma may be related to the regulation of pyrimidine metabolism, steroid hormone biosynthesis, and the TCA cycle. Further studies can be carried out using targeted metabolomics and in vitro models to deeply elucidate the anti-inflammatory and anti-oxidative mechanisms of SAL on asthma based on regulating metabolism.
Introduction
Asthma is a chronic non-specific airway disease involving several types of inflammatory cells. It is characterized by chronic airway inflammation, recurrent airway obstruction, and bronchial hyperresponsiveness. According to the Global Initiative for Asthma, the number of asthmatic patients will reach 400 million worldwide by 2025.1 In the past decade, asthma prevalence has increased significantly in children.2 There are many types of medications used to treat asthma including bronchodilators (e.g., β2 agonists, etc.), anti-inflammatory drugs (e.g., glucocorticoids, sodium cromoglycate, etc.), and immunomodulators. However, most drugs used to treat asthma (e.g., glucocorticoids and β2 agonists) have serious side effects, and an overdose may cause tachycardia, muscle tremors, and inhibition of bone metabolism and adrenal gland functions.3 Therefore, the development of a safe and effective medication to relieve asthma has become a prolific field of study.
Several fundamental studies have found that natural ingredients in plants and nutrients have significant advantages in improving the inflammatory response and airway remodeling in asthma.4 Elucidating the mechanisms of these active components for asthma treatment could contribute to the development of new asthma medications. Some lipid mediators such as n-3 polyunsaturated fatty acids (n-3 PUFAs) and eicosapentaenoic acid (EPA) have been reported to have significant ameliorative effects on asthma by reducing airway inflammation.5 Embelin could alleviate airway inflammation in asthmatic model rats by suppressing the T helper cell (Th2)-mediated response.6 Safranal alleviated the ovalbumin (OVA)-induced airway inflammatory response in asthmatic mice by inhibiting mast cell activation.7 Cycloastragenol inhibited the inflammatory response and airway remodeling in asthmatic mice by inhibiting airway epithelial cell autophagy.8 Ginsenoside Rg3 exerted a therapeutic effect on asthma by inhibiting oxidative stress in airway epithelial cells.9 Schisandrin B inhibited the inflammatory response in asthmatic mice models by modulating the nuclear factor-kappa B (NF-κB) signaling pathway.10
With the widespread applications of untargeted metabolomics, many researchers have confirmed that the changes in the human body's metabolic levels are closely correlated with the onset and development of asthma. Compared with healthy individuals, serum levels of monosaccharides, retinyl ester, lysophosphatidylcholine (lysoPC) (18
:
1), phosphatidylcholine (PC) (18
:
1/2
:
0), phosphatidylethanolamine (PE) (18
:
3/14
:
0), lysoPC (o-18
:
0), arachidonic acid, and PC (16
:
0/18
:
1) were increased in patients with asthma, whereas, the serum levels of glycerophosphocholine, phytosphingosine, phosphatidylserine (PS) (18
:
0/22
:
5), cholesterol glucuronide, sphinganine, lysoPC (p-18
:
1), retinol, and PC (20
:
4/16
:
1) were decreased. Alterations in these metabolites are closely correlated with asthmatic pathological responses including airway inflammation, airway injury, and abnormal airway mucus secretion.11 The regulation of metabolite levels provides new ideas for elucidating the mechanisms of the medical treatment of asthma. Opuntia humifusa increases serum levels of urea and glycerol in asthmatic mice.12 Paeoniflorin may exert therapeutic effects on asthma by regulating fatty acid metabolism in the lung tissue.13
Salidroside (SAL) is a natural component of Rhodiola rosea with anti-inflammatory and anti-oxidative properties. SAL has significant therapeutic effects on OVA-induced asthmatic mice.14 SAL can reduce the inflammatory response in asthmatic model mice by inhibiting the activation of NF-κB and p38 mitogen-activated protein kinase (MAPK).15 However, its effects on asthma and mechanism of action have not been well explored yet. Owing to the significance of host metabolism regulation in asthma treatment, the use of untargeted metabolomics can enhance our understanding of the metabolic regulatory mechanisms of herbs on asthma. This study aimed to demonstrate the metabolic regulatory mechanisms of SAL on asthma using untargeted metabolomics.
Materials and methods
Reagents
OVA (cat: A800) was obtained from Solarbio Biotechnology Co., Ltd (Beijing, China). SAL (C14H20O7, molecular weight 300.3, purity ≥98%, cat: B20504) and dexamethasone (DXM; cat: S17003) were obtained from Shanghai Yuanye Biotechnology Co., Ltd (Shanghai, China). Total protein (cat: A045-4), SOD (cat: A001-3-2), MDA (cat: A003-1-2), and GSH-Px (cat: A006-2-1) assay kits were obtained from Nanjing Jiancheng Biological Engineering Institute (Nanjing, China). Enzyme-linked immunosorbent assay (ELISA) kits of mouse IFN-γ (cat: EK280HS), IL-4 (cat: EK204), IL-5 (cat: EK205), IL-10 (cat: EK210), IL-13 (cat: EK213), and immunoglobulin E (IgE; cat: EK275) were purchased from Multi-Science Biotechnology Co., Ltd (Hangzhou, China). α-SMA polyclonal antibody (cat: 14395-1-AP), MMP2 polyclonal antibody (cat: 10373-2-AP), MMP9 (N-terminal) polyclonal antibody (cat: 10375-2-AP), and TGF-β1 polyclonal antibody (cat: 21898-1-AP) were purchased from Proteintech Group, Inc. (Wuhan, China). RNA extraction (cat: DP419), cDNA reverse transcription (cat: R6906), and SYBR Green polymerase chain reaction (PCR) amplification (cat: S7516) kits were purchased from Tiangen (Beijing, China).
Experimental animals
Fifty male BALB/c mice (6–8 weeks old, 20 ± 2 g) were provided by Beijing Huafukang, Certificate of Approval No. SCXK (Beijing) 2019-0008. The mice were maintained in a specific pathogen-free (SPF) grade clean environment with free access to food and water. All procedures were approved by the Animal Medicine and Animal Protection Ethics Committee of Qingdao University.
Development of asthmatic mouse model
After one week of acclimation, the mice were intraperitoneally injected with 0.2 mL saline mixture containing 20 μg OVA with 1 mg aluminum hydroxide (20 μg OVA + 1 mg aluminum hydroxide + 200 μL saline) on days 0, 7, and 14. From day 21 onwards, sensitized mice were housed in an airtight room and nebulized with 1% OVA for 30 min once every two days for 4 consecutive weeks.16
Grouping and dosing regimens
The 50 mice were equally grouped into control, model, DXM, SAL low-dose, and SAL high-dose groups. The mice in the control group were intraperitoneally injected with 0.2 mL saline on days 0, 7, and 14 of feeding. From day 21 onwards, the mice in the control group were placed in an airtight chamber and nebulized with saline for 30 min once every two days for 4 consecutive weeks. The model, DXM, SAL low-dose, and SAL high-dose groups were used to establish the asthma model via OVA injection and nebulization. From day 21, mice in the control and model groups were intragastrically administered 0.2 mL saline daily. Meanwhile, mice in the DXM, SAL low-dose, and SAL high-dose groups were intragastrically administered 2 mg kg−1 DXM, and 24 mg kg−1 and 48 mg kg−1 SAL daily for 4 weeks, respectively (Fig. 1).
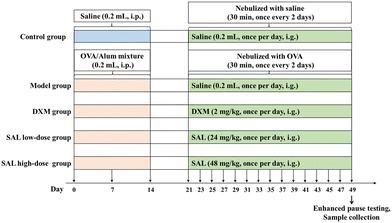 |
| Fig. 1 Experimental design and dosing regimen of this study. | |
Enhanced pause testing
After 4 weeks of SAL intervention, mice were anesthetized with 1% sodium pentobarbital. The trachea was fully exposed and a catheter was inserted after the mice were stabilized. One end of the catheter was connected to the trachea and the other end to the EMKA Small Animal Lung Function Test System Ventilator. The mice in the control group were first exposed to a phosphate buffered saline (PBS) nebulizer to obtain Penh baseline values through direct administration of acetylcholine aerosol using a ventilator. Then, each mouse was exposed to different concentrations of acetylcholine (Ach; 6.25, 12.5, 25, and 50 g L−1) and their Penh values were recorded for 3 minutes per dose.
Bronchoalveolar lavage fluid (BALF) collection
After 4 weeks of SAL intervention, the mice were anesthetized. Then, a syringe was used to collect blood from the abdominal aorta. All the samples were centrifuged at 4 °C (3000 rpm, 15 min) for serum collection.
Then, the mice were sacrificed and their thoracic cavities were carefully opened. The cervical trachea was exposed and a Lanz incision was made on the trachea. A sterilized mouse gavage needle was inserted into the lower end of the right bronchus and then the trachea was ligated using surgical sutures. Subsequently, the mouse's hilus of the left lung was firmly ligated using surgical sutures, ensuring that the left lung was in an airtight position. Using a syringe filled with 0.5 mL saline that was connected to the ligated gavage needle, saline was flushed into the right lungs of mice gently. Then, saline was gently withdrawn back to obtain BALF after retaining it in the alveoli for 15–30 s. The injection and withdrawal procedures were repeated thrice, and approximately 1 mL BALF was recovered from each mouse.
Lung wet-to-dry (W/D) weight ratio
The unirrigated left lungs of mice were retained. The left lung's wet mass (W) was measured, followed by drying in a constant temperature oven at 80 °C for 48 hours until the weight no longer changed. At this point, the lung was weighed as the dry weight (D), and the lung tissue W/D was calculated.
BALF total cell count, eosinophil count, and total protein concentration assay
The BALF was centrifuged at 4 °C for ten minutes at 3000 rpm, and the cell precipitate and supernatant were separately collected. The total number of cells in the BALF precipitate was calculated using the cell counting plate. The number of eosinophils in BALF was detected using Wright's–Giemsa staining. The BCA assay kit was used to test the total protein concentration in the BALF supernatant.
Pathological staining of lung tissue
Lung tissue samples of mice were collected, followed by fixation in a formalin solution. The samples were cut into paraffin sections that were routinely stained with hematoxylin and eosin (HE) and Masson and then sealed with neutral balsam. The histopathological changes in each group's lung tissue samples were observed under a microscope, and the inflammation levels of the lung tissue samples were scored based on the inflammation scale17 (Table S1†). Collagen deposition in the lungs was observed by Masson staining and was scored as described previously (Zhou et al., 2016) (Table S2†).49
ELISA
IL-4, IL-5, IL-13, IFN-γ, and IL-10 in BALF supernatants and the IgE levels in serum were measured based on the ELISA kit's instructions.
Lung tissue biochemical test
Saline (450 μL) was added to a sample of lung tissue weighing 50 mg. Ultrasonic homogenization was performed to prepare lung tissue homogenates, and then centrifugation was performed at 4 °C for 10 min at 3000 rpm. Finally, the supernatants of the homogenized tissues were collected. Based on the BCA kit's instructions, protein levels in the tissue homogenates were measured, which were then used to detect the activities of SOD, GSH-Px, and MDA.
Immunohistochemistry staining
Lung tissue samples of mice were collected, followed by fixation in formalin solution and made into paraffin sections. Antigen repair was performed after dewaxing and hydration. The sections were permeabilized with 0.5% Triton X-100 for 5 minutes, blocked with 5% bovine serum albumin (BSA), and incubated at 37 °C for 1 hour. The sections were incubated with primary antibody α-SMA (1
:
500), MMP2 (1
:
500), MMP9 (1
:
500), and TGF-β1 (1
:
500) overnight at 4 °C and washed thrice with PBS. Then, a secondary antibody was added and incubated for 1 hour at room temperature, and sections were subsequently washed three times with PBS. After that, conventional DAB was used for color development, re-staining, dehydration, and transparency. Finally, the slides were mounted with neutral balsam and the histopathological changes in each group of mice were observed under a light microscope. Furthermore, the area of positive expression was quantified using Image-Pro Plus v.6.0, and the average optical density (AOD) was calculated with the formula: AOD = integrated optical density (IOD)/total area.
Real-time quantitative PCR (RT-qPCR)
The mRNA expression of smooth muscle actin (Acta2), matrix metallopeptidase 2 (Mmp2), matrix metallopeptidase 9 (Mmp9) and transforming growth factor beta 1 (Tgfb1) in lung tissue was detected by RT-qPCR. Briefly, total RNA was extracted from lung tissue and reversely transcribed into cDNA. PCR amplification was performed using cDNA as a template. Primers and SuperReal PreMix Plus were added according to the kit instructions. β-Actin (Actb) was used as a loading control. The primer sequences are shown in Table S3.† RT-qPCR was conducted on a BioRad iQ5 Detection System. The relative expression of each gene was calculated using the 2−ΔΔCT protocol as described previously.18
Metabolomics analysis
Lung tissue (100 mg) was added to 500 μL 80% methanol solution and then ultrasonic homogenization followed by centrifugation at 4 °C (15
000g, 20 min) was performed. The supernatant was then collected and diluted with water to reach a 53% methanol level. Next, the collected supernatant was centrifuged at 4 °C (15
000g, 20 min) to obtain a tissue homogenate for untargeted metabolomic analysis and liquid chromatography-mass spectrometry (LC-MS). The chromatographic and mass spectrometric conditions were established and data processing and analysis were conducted as previously described.19
Statistical processing
Data are expressed as mean ± standard deviation. Statistical analysis was conducted using a one-way analysis of variance (ANOVA) followed by Tukey's post hoc test, where differences were considered statistically significant at a P value less than 0.05.
Results
Therapeutic effects of SAL on asthmatic model mice
The Penh value in the model group was significantly higher than that in the control group after treatment with different concentrations of Ach (P < 0.01, respectively). Penh values in the DXM and SAL high-dose groups were significantly decreased after 6.25 mg mL−1 Ach treatment compared with those of the model group (P < 0.01, respectively); Penh values in the DXM (P < 0.01), SAL low-dose (P < 0.05), and SAL high-dose (P < 0.01) groups were significantly decreased after 12.5 mg mL−1 Ach treatment; Penh values in the DXM and SAL high-dose groups were greatly decreased after 25 mg mL−1 Ach treatment (P < 0.05, respectively); Penh values in the DXM (P < 0.01), SAL low-dose (P < 0.05), and SAL high-dose (P < 0.01) groups were significantly decreased after 50 mg mL−1 of ACh treatment (Table 1).
Table 1 Effects of SAL on Penh in mice with asthma
Group |
Acetylcholine (mg mL−1) |
0 |
6.25 |
12.5 |
25 |
50 |
Control, model, DXM, SAL low-dose and SAL high-dose groups (n = 10 per group). ##P < 0.01 compared with the control group; *P < 0.05 compared with the model group; **P < 0.01 compared with the model group. |
Control |
43.41 ± 5.64 |
80.97 ± 15.96 |
110.47 ± 13.25 |
117.94 ± 12.85 |
122.13 ± 13.09 |
Model |
44.4 ± 7.22 |
117.3 ± 13.74## |
240.65 ± 38.35## |
283.88 ± 31.49## |
369.02 ± 29.27## |
DXM |
44.64 ± 4.75 |
94.54 ± 21.33* |
179.94 ± 45.91** |
238.4 ± 42.76* |
301.42 ± 36.66** |
SAL low-dose |
46.58 ± 5.17 |
105.38 ± 13.21 |
202.32 ± 35.43* |
264.28 ± 14.88 |
333.18 ± 27.23* |
SAL high-dose |
46.54 ± 8.34 |
96.33 ± 24.14* |
189.7 ± 32.46** |
244.66 ± 42.33* |
321.67 ± 36.16** |
The lung tissue W/D ratio of asthmatic mice was significantly elevated (P < 0.01) as compared with that of the control group. The ratios in the DXM and SAL high-dose treatment groups significantly decreased (P < 0.01, respectively, Fig. 2A) as compared with those of the model group. Compared to the control group, the total protein content of the BALF supernatant was significantly increased in the model group (P < 0.01); however, it was significantly decreased in DXM (P < 0.01), SAL low-dose (P < 0.05), and SAL high-dose (P < 0.01) groups (Fig. 2B). BALF total cell count was significantly increased in the model group (P < 0.01) compared with that of the control and model groups, however, it was significantly decreased in DXM, low-dose, and high-dose SAL treatment groups (P < 0.01, respectively, Fig. 2C). More eosinophils were found in BALF in the model group (P < 0.01) compared with those in the control group. However, compared with the model group, eosinophil levels in BALF were lower in DXM, SAL low-dose, and SAL high-dose groups (P < 0.01, respectively, Fig. 2D). Additionally, the serum IgE level was increased in the model group (P < 0.01) as compared with that in the control group but significantly decreased in the DXM, SAL low-dose, and SAL high-dose groups (P < 0.01, respectively, Fig. 2E) compared with that in the model group.
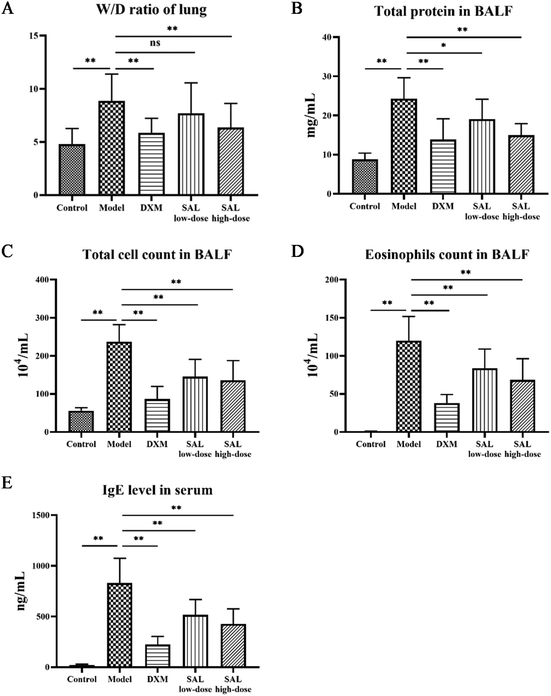 |
| Fig. 2 SAL treatment ameliorated asthma in mice. (A) SAL treatment lowered the W/D ratio. (B) SAL treatment reduced the total protein content in BALF. (C) SAL treatment decreased the total cell count in BALF. (D) SAL decreased the eosinophils count in BALF. (E) SAL treatment decreased the level of IgE in serum. *P < 0.05; **P < 0.01; ns: not statistically significant. | |
HE staining showed that no pathological changes were observed in the lungs of the control group. The irregular arrangement of bronchial epithelial cells and a considerable number of inflammatory cells were observed in the model group. DXM and SAL treatments improved these pathological changes in the lungs (Fig. 3A). Likewise, the inflammation score of the model group was remarkably higher than that of the control group (P < 0.01). However, the inflammation scores were lower in the DXM (P < 0.01), SAL low-dose (P < 0.05), and SAL high-dose (P < 0.01) groups compared with those in the model group (Fig. 3C). Masson staining showed obvious collagen deposition in the lungs in the model group, and fewer collagen contents could be observed in the lungs of DXM and SAL-treated mice compared with those of the model group (Fig. 3B). The Masson score was higher in the model group than that in the control group (P < 0.01), and lower in DXM and SAL high-dose (P < 0.01, respectively) groups compared with that in the model group (Fig. 3D).
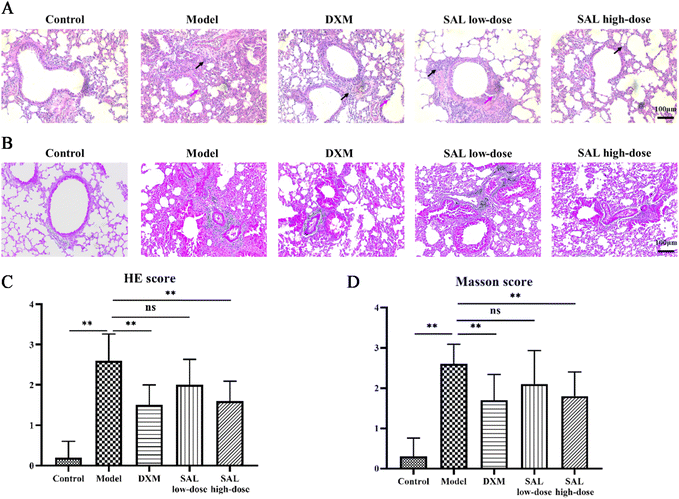 |
| Fig. 3 SAL reduced the pathological changes in asthmatic model mice. (A and C) HE staining showed that SAL reduced the infiltration of inflammatory cells and airway epithelial cell injury (red arrows indicated the airway epithelial cell injury and black arrows indicated the infiltration of inflammation cells) in the lungs (A) and decreased the HE scores (C) (magnification: 100×). (B and D) Masson staining showed that SAL reduced the deposition of collagen contents in the lungs (B) and decreased the Masson scores (D) (magnification: 100×). | |
Effects of SAL on inflammation and oxidative stress in asthmatic mice
The levels of cytokines in BALF were measured to see how SAL affected inflammation in model mice. MDA levels in addition to SOD and GSH-Px activities in the lung tissue homogenate were also tested to evaluate SAL's impact on oxidative stress. ELISA results showed that IL-4, IL-5, and IL-13 levels in the model group increased as expected compared with those of the control group (P < 0.01), while these cytokine levels were significantly reduced in the DXM group compared with those of the model group (P < 0.01, respectively). SAL treatment lowered IL-4, IL-5, and IL-13 levels in a dose-dependent manner (Fig. 4A–C). IFN-γ and IL-10 levels in the model group were lower than that in the control group (P < 0.01, respectively). DXM and SAL high-dose treatment both had elevated IFN-γ and IL-10 levels compared with the model group (P < 0.01, respectively, Fig. 4D and E).
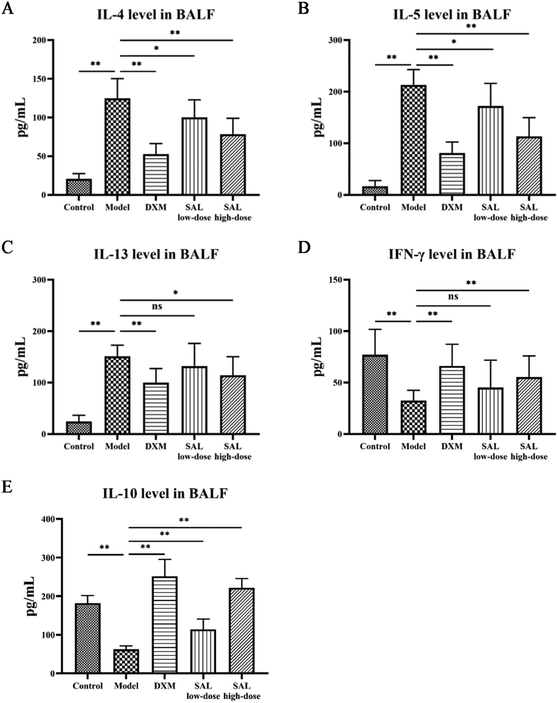 |
| Fig. 4 SAL treatment inhibited the inflammatory response in mice with asthma. The cytokine levels of IL-4, IL-5, IL-13, IFN-γ and IL-10 in BALF in each group were measured using ELISA. Results showed that SAL treatment decreased the levels of IL-4 (A), IL-5 (B) and IL-13 (C) and increased the level of IFN-γ (D) and IL-10 (E) in BALF. | |
SOD and GSH-Px activities dropped while MDA levels increased in the model group compared with those in the control group (P < 0.01, respectively), whereas SOD and GSH-Px activities increased and MDA levels decreased in the SAL high-dose group compared with those in the model group (P < 0.05, P < 0.01 P < 0.05, respectively, Table 2).
Table 2 Effects of SAL on SOD and GSH-Px activities and MDA level in lung tissue homogenate in asthmatic model mice
Group |
SOD (U mgprot−1) |
MDA (nmol mgprot−1) |
GSH-Px (U mgprot−1) |
##
P < 0.01 compared with the control group; *P < 0.05 compared with the model group; **P < 0.01 compared with the model group. |
Control |
58.82 ± 13.16 |
2.12 ± 0.61 |
24.14 ± 6.3 |
Model |
28.58 ± 14.28## |
4.2 ± 1.23## |
8.96 ± 3.91## |
DXM |
41.48 ± 11.41* |
2.64 ± 1.04** |
15.21 ± 5.34** |
SAL low-dose |
36.32 ± 7.01 |
3.18 ± 0.93* |
11.53 ± 4.43 |
SAL high-dose |
46.09 ± 14.1* |
3.07 ± 1.04* |
15.82 ± 5.55** |
Effects of SAL on airway remodeling in mice with asthma
The effect of SAL on airway remodeling was then studied through the expression of α-SMA, MMP2, MMP9, and TGF-β1 in the lungs via immunohistochemistry staining. The results showed that the expression of α-SMA, MMP2, MMP9, and TGF-β1 was significantly increased in the lungs of mice in the model group compared with that in the control group (P < 0.01, P < 0.01, P < 0.05, P < 0.01, respectively), while DXM and high-dose of SAL treatment reduced the levels of α-SMA (P < 0.01, respectively, Fig. 5A and B), MMP2 (P < 0.01, respectively, Fig. 5A and C), MMP9 (P < 0.05, respectively, Fig. 5A and D), and TGF-β1 (P < 0.01, Fig. 5A and E), respectively.
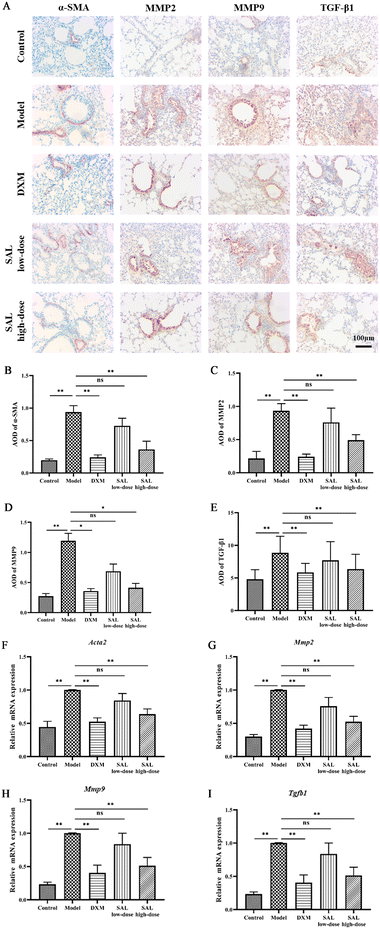 |
| Fig. 5 SAL treatment improved airway remodeling in mice with asthma. The protein levels of α-SMA, MMP2, MMP9, and TGF-β1 in lung tissue were detected using immunohistochemical staining (A) (magnification: 100×) and the positive expression area was quantified using Image Pro Plus v.6.0 (B–E). The result showed that SAL treatment decreased the protein levels of α-SMA (A and B), MMP2 (A and C), MMP9 (A and D), and TGF-β1 (A and E) in the lung tissue. Besides, the gene expression of Acta2, Mmp2, Mmp9 and Tgfb1 was investigated using PCR (F–I). SAL down-regulated the gene expression of Acta2 (F), Mmp2 (G), Mmp9 (H), and Tgfb1 (I) in the lungs. | |
Moreover, the mRNA expression of Acta2, Mmp2, Mmp9 and Tgfb1 was up-regulated in the model group compared with the control group (P < 0.01, respectively). DXM and high-dose of SAL treatment down-regulated the mRNA expression of Acta2 (Fig. 5F), Mmp2 (Fig. 5G), Mmp9 (Fig. 5H) and Tgfb1 (Fig. 5I) in asthmatic model mice (P < 0.01, respectively).
Effects of SAL on metabolite levels in the lungs in mice with asthma
Untargeted metabolomics analysis was used to explore the effects of SAL on the lung metabolites of asthmatic mice. The principal component analysis (PCA) model showed that the control and model groups were well differentiated and that the model and SAL high-dose groups were well differentiated as well. The distance between the control and SAL high-dose groups was closer than the distance between the control and model groups (Fig. 6A). Adonis 2 test also showed a significant difference between the model and control groups and between the SAL high-dose and model groups (Table 3). Furthermore, the partial least squares discriminant analysis (PLS-DA) model was used to obtain the variable influence on the projection (VIP) of each metabolite. The model group had an explanatory power (R2) Y = 0.97 and a predictive power (Q2) Y = 0.56 compared to the control group (Fig. 6B), whereas SAL high-dose group had an R2Y = 0.98 and a Q2Y = 0.13 compared to the model group (Fig. 6D). The permutation test of the PLS-DA model suggested that the model was stable with good predictive power and its data can be used to identify differentially expressed metabolites (Fig. 6C and E).
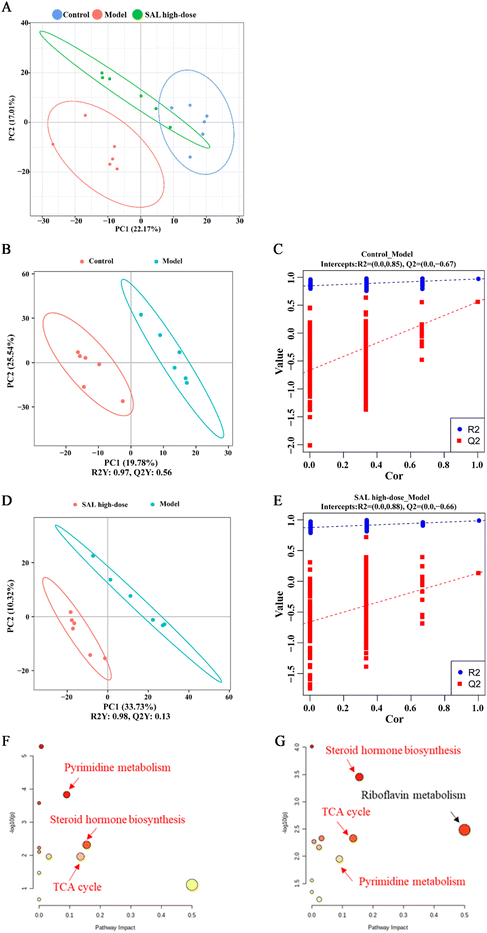 |
| Fig. 6 Untargeted metabolomics showed that high-dose of SAL affected the metabolites in the lungs. (A) Score plot of PCA among control, model and SAL high-dose groups. (B and D) Scores plots of PLS-DA between control and model groups (B) and between model and SAL high-dose groups (D). (C and E) Permutation tests of PLD-DA models between control and model groups (C) and between model and SAL high-dose groups (E). (F and G) Pathway analysis of differential metabolites in the lungs between control and model groups (F) and between model and SAL high-dose groups (G), the common pathways are written in red. | |
Table 3 Adonis2 test of principal component analysis
Pairs |
R
2
|
P value |
Adjusted P value |
C: control group; M: model group; S: SAL high-dose group. |
M vs. C |
0.481 |
0.006 |
0.009 |
S vs. C |
0.201 |
0.087 |
0.087 |
S vs. M |
0.465 |
0.003 |
0.009 |
Differentially expressed metabolites were screened based on the following criteria: (1) P < 0.05 (between control and model groups or between model and SAL high-dose groups); (2) VIP > 1.0 (between control and model groups or between model and SAL high-dose groups); (3) fold change (FC) >1.2 or FC < 0.8 (between control and model groups or between model and SAL high-dose groups).20 Thirty-four metabolites were identified in total (Table 4). Compared with those in the control group, the levels of LysoPC 20
:
2, isoproterenol, 7-ketocholesterol, monobutyl phthalate, L-homocitrulline, citric acid, progesterone, and isocitric acid increased while those of PC (16
:
0/16
:
0), dehydroepiandrosterone, 4-guanidinobutyric acid, vitamin B2, fatty acyl esters of hydroxy fatty acids (FAHFA) (16
:
0/18
:
2), estriol, methyl indole-3-acetate, pantothenic acid, acetyl-L-carnitine, uridine, testosterone, uracil, and DL-glutamine were significantly decreased in the model group. Levels of PC (16
:
0/16
:
0), dehydroepiandrosterone, 4-guanidinobutyric acid, vitamin B2, FAHFA (16
:
0/18
:
2), estriol, methyl indole-3-acetate, 3-indoxyl sulfate, 2′-O-methyluridine, L-asparagine, pantothenic acid, pregnenolone, acetyl-L-carnitine, uridine, testosterone, uracil, and DL-glutamine were significantly increased, whereas those of LysoPC 20
:
2, isoproterenol, 7-ketocholesterol, monobutyl phthalate, L-homocitrulline, citric acid, progesterone, and isocitric acid were significantly decreased in the SAL high-dose group compared with those in the model group (Table 4).
Table 4 Differential metabolites in asthmatic model mice after the treatment of SAL
No. |
Formula |
RT [min] |
m/z |
Metabolites |
VIP |
FC |
Trend |
Pathway |
M vs. C |
S vs. M |
M vs. C |
S vs. M |
M vs. C |
S vs. M |
Control, model, and SAL high-dose groups (n = 6 per group). RT: retention time; VIP: variable importance of projection; FC: fold change; ↑: content increased; ↓: content decreased; vs.: versus; C: control group; M: model group; S: SAL high-dose group. a: Pyrimidine metabolism; b: Steroid hormone biosynthesis; c: TCA cycle; d: Riboflavin metabolism. |
1 |
C40H80NO8P |
14.73 |
774.56 |
PC (16 : 0/16 : 0) |
1.79 |
1.01 |
0.61 |
1.27 |
↓## |
↑** |
|
2 |
C28H54NO7P |
15.16 |
546.36 |
LysoPC 20 : 2 |
1.85 |
1.70 |
3.55 |
0.23 |
↑## |
↓** |
|
3 |
C19H28O2 |
16.52 |
271.20 |
Dehydroepiandrosterone |
1.59 |
1.15 |
0.40 |
3.08 |
↓## |
↑** |
|
4 |
C5H11N3O2 |
1.41 |
146.09 |
4-Guanidinobutyric acid |
2.34 |
1.29 |
0.24 |
2.50 |
↓## |
↑** |
|
5 |
C11H17NO3 |
11.10 |
212.13 |
Isoproterenol |
2.03 |
1.55 |
2.95 |
0.41 |
↑# |
↓* |
|
6 |
C17H20N4O6 |
8.51 |
375.13 |
Vitamin B2 |
1.67 |
1.27 |
0.72 |
3.79 |
↓## |
↑** |
d |
7 |
C34H62O4 |
14.86 |
533.45 |
FAHFA (16 : 0/18 : 2) |
1.43 |
1.31 |
0.69 |
1.43 |
↓## |
↑** |
|
8 |
C18H24O3 |
6.72 |
311.16 |
Estriol |
1.70 |
1.23 |
0.53 |
2.37 |
↓# |
↑* |
b |
9 |
C11H11NO2 |
10.55 |
190.09 |
Methyl indole-3-acetate |
1.43 |
1.38 |
0.52 |
2.63 |
↓# |
↑* |
|
10 |
C27H44O2 |
14.05 |
401.34 |
7-Ketocholesterol |
1.45 |
2.36 |
1.44 |
0.55 |
↑# |
↓** |
|
11 |
C18H34O4 |
14.35 |
315.25 |
9,10-Dihome |
2.00 |
1.39 |
2.06 |
0.50 |
↑# |
↓* |
|
12 |
C8H7 NO4S |
6.56 |
212.00 |
3-Indoxyl sulphate |
1.71 |
1.28 |
0.45 |
3.66 |
↓# |
↑** |
|
13 |
C12H14O4 |
10.04 |
221.08 |
Monobutyl phthalate |
1.31 |
1.21 |
1.98 |
0.34 |
↑# |
↓** |
|
14 |
C10H14N2O6 |
1.40 |
259.09 |
2′-O-Methyluridine |
1.95 |
1.62 |
0.42 |
2.30 |
↓# |
↑* |
|
15 |
C7H15N3O3 |
1.41 |
190.12 |
L-Homocitrulline |
1.68 |
1.07 |
2.64 |
0.50 |
↑# |
↓* |
|
16 |
C4H8N2O3 |
1.51 |
115.05 |
L-Asparagine |
1.29 |
1.02 |
0.58 |
1.65 |
↓# |
↑* |
|
17 |
C9H17NO5 |
6.42 |
220.12 |
Pantothenic acid |
1.40 |
1.43 |
0.46 |
1.78 |
↓## |
↑** |
|
18 |
C21H32O2 |
13.54 |
317.25 |
Pregnenolone |
1.28 |
1.09 |
0.47 |
1.62 |
↓# |
↑* |
b |
19 |
C9H17NO4 |
1.42 |
204.12 |
Acetyl-L-carnitine |
1.33 |
1.41 |
0.58 |
3.59 |
↓# |
↑* |
|
20 |
C6H8O7 |
2.08 |
191.02 |
Citric acid |
1.62 |
1.70 |
1.94 |
0.53 |
↑# |
↓** |
c |
21 |
C9H12N2O6 |
1.62 |
243.06 |
Uridine |
1.85 |
1.22 |
0.56 |
1.83 |
↓# |
↑* |
a |
22 |
C19H28O2 |
12.65 |
289.22 |
Testosterone |
1.84 |
1.05 |
0.73 |
1.71 |
↓## |
↑** |
b |
23 |
C21H30O2 |
13.32 |
315.23 |
Progesterone |
1.73 |
1.12 |
2.18 |
0.34 |
↑# |
↓** |
b |
24 |
C4H4N2O2 |
1.54 |
113.03 |
Uracil |
2.41 |
1.36 |
0.31 |
1.84 |
↓## |
↑* |
a |
25 |
C5H10N2O3 |
1.28 |
147.08 |
DL-Glutamine |
2.75 |
1.37 |
0.45 |
5.68 |
↓## |
↑** |
|
26 |
C6H8O7 |
1.21 |
191.02 |
Isocitric acid |
1.99 |
1.32 |
5.09 |
0.20 |
↑# |
↓* |
c |
27 |
C10H20O3 |
11.94 |
187.13 |
10-Hydroxydecanoic acid |
1.47 |
1.02 |
0.60 |
1.20 |
↓# |
↑ |
|
28 |
C22H46NO7 P |
14.35 |
526.31 |
LPC 14 : 0 |
1.32 |
1.25 |
0.67 |
1.32 |
↓# |
↓ |
|
29 |
C24H40O5 |
12.82 |
407.28 |
Cholic acid |
1.63 |
1.11 |
3.46 |
0.87 |
↑## |
↓ |
|
30 |
C23H46NO7P |
15.00 |
478.29 |
LPE 18 : 1 |
1.25 |
1.10 |
0.65 |
1.69 |
↓ |
↑* |
|
31 |
C9H7N |
10.55 |
130.06 |
Isoquinoline |
1.32 |
1.23 |
0.88 |
2.33 |
↓ |
↑* |
|
32 |
C10H19NO4 |
1.56 |
218.14 |
Propionylcarnitine |
1.69 |
1.29 |
1.34 |
0.59 |
↑ |
↓* |
|
33 |
C3H7N3O2 |
1.31 |
118.06 |
Guanidineacetic acid |
1.92 |
1.40 |
0.76 |
1.39 |
↓ |
↑* |
|
34 |
C7H13NO2 |
1.40 |
144.10 |
DL-Stachydrine |
1.23 |
1.39 |
0.52 |
1.68 |
↓ |
↑** |
|
Pathway analysis of differential metabolites
The Kyoto Encyclopedia of Genes and Genomes (KEGG) database and the MetaboAnalyst platform were utilized for the metabolic pathway enrichment analysis of the differentially expressed metabolites in the model group. Different pathways were picked based on pathway impact >0.05 and P < 0.05. Differential metabolic pathways between the control and model groups included pyrimidine metabolism, steroid hormone biosynthesis, and the tricarboxylic acid (TCA) cycle (Fig. 6F). Differential metabolic pathways between the model and SAL high-dose groups included pyrimidine metabolism, steroid hormone biosynthesis, TCA cycle, and riboflavin metabolism (Fig. 6G). Among these pathways, pyrimidine metabolism, steroid hormone biosynthesis, and the TCA cycle were pathways common to all three groups.
Discussion
In this study, an asthma model was developed using OVA. The results showed that the lung function of the model mice was reduced. Lung histopathology revealed many infiltrating inflammatory cells and significant accumulation of collagen in the lung tissue. Additionally, the lung tissue W/D ratio, total BALF cell counts, eosinophil counts, and total protein were increased in the model group, indicating increased lung tissue permeability. Mice in the model group also showed higher IgE levels in serum. These results were consistent with the pathological manifestations of asthma, indicating that the model was successfully developed. Additionally, the results show that SAL intervention significantly improved lung function by reducing lung tissue permeability and downregulating serum IgE levels in asthmatic mice. Additionally, DXM, a hormonal drug commonly used to treat asthma, was selected as a positive control reagent.21 The results showed that high-dose SAL differed insignificantly from DXM in improving lung function. The results confirmed that SAL had therapeutic effects on asthma, which is consistent with previous reports.22
Airway inflammation and oxidative stress are major pathological manifestations of asthma. Therefore, the effects of SAL on airway inflammation and oxidative stress in asthmatic mice were further studied. The results showed that the SAL intervention reduced IL-4, IL-5, and IL-13 levels and increased IFN-γ and IL-10 levels in BALF of asthmatic mice. The balance of Th1 and Th2 is important for maintaining the immune homeostasis of the body, and their imbalance is an important mechanism for airway inflammation development in asthma. A study reported reduced Th1 levels in asthma, which reduced the levels of certain Th1-secreted cytokines, including IFN-γ, and elevated Th2 levels, which in turn could produce large amounts of IL-4, IL-5, and IL-13. These secreted cytokines can promote the activation of mast cells, eosinophils, etc., causing airway constriction and aggravating the onset of allergic reactions (Lee et al., 2001).50 Decreasing the Th2-correlated cytokines and increasing the Th1 cytokine, IFN-γ, may help alleviate the inflammatory responses in asthma. Additionally, IL-10 is an important immunomodulatory factor, mainly produced by T regulatory cells (Treg), which inhibits inflammatory responses.23 Oxidative stress is also an important pathological response in asthma, where prolonged airway inflammation can directly cause excessive ROS production in lung tissue.24 Excessive ROS can cause lipid peroxidation and oxidative DNA damage of cells in the lungs, further contributing to the injury to airway smooth muscle cells. The results also showed that SAL increased SOD and GSH-Px activities and decreased MDA levels in the lung tissue of asthmatic mice. MDA is a cytotoxic lipid peroxidation end product, and its levels are positively correlated with the degree of oxidative stress.25 The MDA level was increased in asthmatic patients and positively correlated with the severity of asthma.26 SOD and GSH-Px are both antioxidant enzymes involved in the ROS scavenging process.27 SOD promotes ROS conversion to H2O2, where H2O2 is further converted to H2O and O2, a reaction that is catalyzed by GSH-Px. The elevated SOD and GSH-Px activities protect the lung tissue from oxidative stress damage.28
Structural changes in the airway wall can cause airflow obstruction, bronchial hyperresponsiveness, and chronicity in asthma.29 The effect of SAL on airway remodeling was also tested in this study. The results showed that SAL reduced the gene expression of α-SMA, MMP2, MMP9, and TGF-β1 in the lung tissue of asthmatic mice. Asthma may manifest as fibrosis of the airway epithelium and hypertrophy of the airway smooth muscle. The α-SMA is an important marker of airway smooth muscle cells, and its abnormally elevated levels may indicate the excessive proliferation of airway smooth muscles.30 Additionally, elevated levels of MMP2 and MMP9, members of the metalloproteinase family, degrade airway matrix proteins (e.g., collagen and elastin), causing an imbalance between extracellular matrix (ECM) degradation and synthesis in airway smooth muscles. This leads to ECM deposition in the airway, promoting fibrosis and exacerbating airway inflammation and bronchial hyperresponsiveness.31 TGF-β1 belongs to the transforming growth factor family, and its elevated levels promote the proliferation of bronchial epithelial and smooth muscle cells. Asthma airway remodeling can be improved by decreasing α-SMA, MMP2, MMP9, and TGF-β1 expression levels.32
Next, untargeted metabolomics analysis was used to explore the influence of SAL on metabolites in the lungs of asthmatic mice. PCA results showed significant changes in lung tissue metabolism in asthmatic and control mice. After SAL treatment, the rate of metabolism in the lung tissue of asthmatic mice was significantly affected. Differential metabolite analysis results showed that SAL influenced the levels of 31 metabolites, including FAHFA (16
:
0/18
:
2), 2′-O-methyluridine, L-asparagine, pantothenic acid, acetyl-L-carnitine, PC (16
:
0/16
:
0), DL-glutamine, monobutyl phthalate, and L-homocitrulline. Among them, SAL increased the levels of FAHFA (16
:
0/18
:
2), 2′-O-methyluridine, L-asparagine, pantothenic acid, acetyl-L-carnitine, PC (16
:
0/16
:
0), and DL-glutamine in the lung tissue. FAHFA (16
:
0/18
:
2) inhibits inflammatory responses33 and oxidative stress damage by activating the nuclear factor erythroid 2-related factor 2 (NRF2) signaling pathway.34In vitro and in vivo studies have found that 2′-O-methyluridine inhibits the activity of inducible nitric oxide synthase (iNOS), which then inhibits macrophage activation and exerts anti-inflammatory effects.35L-Asparagine has also been shown to have anti-inflammatory effects by inhibiting the inflammatory response induced by lipopolysaccharide (LPS).36 Pantothenic acid is commonly known as vitamin B5 and has anti-inflammatory effects.37 Acetyl-L-carnitine has an anti-oxidative effect, and it was found that feeding it to aged rats significantly inhibited their oxidative stress responses.38 Further studies revealed that acetyl-L-carnitine could exert anti-oxidative and cytoprotective effects by regulating phospholipid levels in cells, thereby improving their mitochondrial function.39 PC (16
:
0/16
:
0) is a major component of the surface-active component of the lung and plays a vital role in maintaining lung tone. Reduced PC levels can induce pathological changes, including pulmonary edema.40DL-Glutamine relieves asthmatic airway inflammation by inhibiting Th2 cell activation.41 Additionally, SAL reduced the levels of monobutyl phthalate and L-homocitrulline in the lung tissue of asthmatic mice. Monobutyl phthalate levels were significantly elevated in patients with asthma and correlated negatively with the peak expiratory flow rate.42 Additionally, a metabolomic study compared the smoking population with the nonsmoking population and found that L-homocitrulline levels were significantly higher in the smoking population;43 however, the relationship between L-homocitrulline and asthma remains to be further investigated.
Further analysis of the metabolic pathways of the differential metabolites showed that pyrimidine metabolism, steroid hormone biosynthesis, and TCA cycle pathways were the common pathways that changed among the control, model, and SAL high-dose groups. This suggests that the effects of SAL in treating asthma may be mediated through these pathways.
Pyrimidine metabolism
SAL elevated uridine and uracil levels in the lung tissue of asthmatic mice. Both uridine and uracil are important metabolites in pyrimidine metabolism. Uridine has anti-inflammatory activity. One study reported that uridine administration to OVA-induced asthmatic mice significantly reduced IL-4, IL-6, and IL-13 levels in BALF, down-regulated chemokine (C–X–C motif) ligand (CXCL)1, CXCL3, IL-17 levels, and mucin-5AC (Muc5ac) gene expression in the lung tissue, and inhibited MAPK and NF-κB pathway activation.44 Thus, the mechanism by which SAL inhibits the inflammatory responses in asthmatic mice may be associated with the upregulation of pyrimidine metabolism-related metabolites, such as uridine and uracil, in the lung tissue.
Steroid hormone biosynthesis
The results showed that SAL up-regulated the levels of steroid hormone biosynthesis-related metabolites, such as estriol, testosterone, and pregnenolone, whereas it down-regulated pregnenolone levels in the lung tissue of asthmatic mice. Steroid hormone biosynthesis abnormalities are closely correlated with asthma development. Estriol, testosterone, and pregnenolone have anti-inflammatory effects, where estriol inhibits immune cell activation and migration in the lung tissue of mice with acute pneumonia.45 Clinical trials have shown that testosterone inhibits the inflammatory response, whereas pregnenolone exerts anti-inflammatory effects by modulating the innate immune system.46 In contrast, pregnenolone promotes asthma development. One study reported that pregnenolone exacerbates asthma by downregulating let-7f microRNA expression, which then activates IL-23/IL-23 receptor signaling and promotes IL-17A production.47Future studies can further explore the mechanism of action of SAL in asthma treatment by regulating steroid hormone biosynthesis.
TCA cycle
The TCA cycle is the most efficient way for the body to oxidize carbohydrates to yield energy. It is the hub for the metabolic liaison and conversion of carbohydrates, lipids, and amino acids. The results indicated that SAL reduced the levels of TCA cycle-related metabolites, such as citric and isocitric acids, in the lung tissue of asthmatic mice. A study found that citric acid is a distinct asthma inducer, and its application induced cough in mice. Also, asthma can be improved by lowering citric acid levels.48 Citric acid can be converted to isocitric acid by the action of aconitate hydratase. Future studies can be combined with in vitro experiments to further elucidate the mechanism of action of SAL through TCA cycle regulation, thereby improving asthma.
Conclusion and future prospective
In summary, this study suggests that SAL treatment can ameliorate asthma by inhibiting the inflammatory response and oxidative stress as well as improving airway remodeling. The mechanism of SAL in the treatment of asthma may be associated with regulating pyrimidine metabolism, steroid hormone biosynthesis, and the TCA cycle. This study used untargeted metabolomics to explore the mechanisms of SAL on asthma and provided scientific evidence for the anti-asthmatic potentials of natural herbal compounds based on modulating host metabolism. Future studies can use targeted metabolomics to validate the metabolic regulatory mechanisms of SAL on asthma. Additionally, owing to the close relationship between the changes in metabolism and the pathological processes in asthma such as inflammatory response and oxidative stress, further studies can be carried out to explain in detail how SAL can inhibit the inflammatory response and oxidative stress by affecting metabolism using in vitro models.
Author contributions
Kun Wang, Li Wang, and Guojing Zhao carried out the experiments and manuscript writing. Yong Liu and Fengchan Wang provided experimental help. Huan Song and Yin Sun performed data analysis and result interpretation. Zhaoshan Zhou provided resources. Xuechao Lu and Haibo Hu provided ideas and technical guidance for the whole work, Huantian Cui supervised the experiments. All authors contributed to the article and approved the submitted version.
Ethical statement
All animal procedures were performed in accordance with the Guidelines for Care and Use of Laboratory Animals of Qingdao University and approved by the Animal Ethics Committee of Qingdao University.
Conflicts of interest
The authors declared no conflict of interest.
Acknowledgements
This work was supported by the Qingdao Health Science and Technology Project (2020-WJZD053) and the Shandong Provincial TCM Science and Technology Development Plan Project (2019-0596) and TCM Science and Technology Project of Shandong Province (A-1244).
References
- E. D. Bateman, S. S. Hurd and P. J. Barnes,
et al., Global Strategy for Asthma Management and Prevention: GINA Executive Summary, Eur. Respir. J., 2008, 31(1), 143–178 CrossRef CAS PubMed.
- H. E. Hoch, P. R. Houin and P. C. Stillwell, Asthma in Children: A Brief Review for Primary Care Providers, Pediatr. Ann., 2019, 48(3), e103–e109 Search PubMed.
- J. R. Castillo, S. P. Peters and W. W. Busse, Asthma Exacerbations: Pathogenesis, Prevention, and Treatment, J. Allergy Clin. Immunol.: Pract., 2017, 5(4), 918–927 Search PubMed.
- S. Azman, M. Sekar and S. R. Bonam,
et al., Traditional Medicinal Plants Conferring Protection Against Ovalbumin-Induced Asthma in Experimental Animals: A Review, J. Asthma Allergy, 2021, 14, 641–662 CrossRef CAS PubMed.
- J. Zúñiga-Hernández, V. Sambra and F. Echeverría,
et al., N-3 PUFAs and Their Specialized pro-Resolving Lipid Mediators on Airway Inflammatory Response: Beneficial Effects in the Prevention and Treatment of Respiratory Diseases, Food Funct., 2022, 13(8), 4260–4272 RSC.
- S. Azman, M. Sekar and S. Wahidin,
et al., Embelin Alleviates Severe Airway Inflammation in OVA-LPS-Induced Rat Model of Allergic Asthma, J. Asthma Allergy, 2021, 14, 1511–1525 CrossRef CAS PubMed.
- P. Lertnimitphun, W. Zhang, W. Fu, B. Yang, C. Zheng, M. Yuan, H. Zhou, X. Zhang, W. Pei, Y. Lu and H. Xu, Safranal Alleviated OVA-Induced Asthma Model and Inhibits Mast Cell Activation, Front. Immunol., 2021, 12, 585595 CrossRef CAS PubMed.
- X. Zhu, Y. Cao, M. Su, M. Chen, C. Li, L. Yi, J. Qin, W. Tulake, F. Teng, Y. Zhong, W. Tang, S. Wang and J. Dong, Cycloastragenol alleviates airway inflammation in asthmatic mice by inhibiting autophagy, Mol. Med. Rep., 2021, 24(5), 805 CrossRef CAS PubMed.
- W.-C. Huang, T.-H. Huang and K.-W. Yeh,
et al., Ginsenoside Rg3 Ameliorates Allergic Airway Inflammation and Oxidative Stress in Mice, J. Ginseng Res., 2021, 45(6), 654–664 CrossRef PubMed.
- Y. Chen, Y. Kong and Q. Wang,
et al., Schisandrin B Attenuates Airway Inflammation by Regulating the NF-KB/Nrf2 Signaling Pathway in Mouse Models of Asthma, J. Immunol. Res., 2021, 2021, 1–13 Search PubMed.
- C. Wang, S. Jiang and S. Zhang,
et al., Research Progress of Metabolomics in Asthma, Metabolites, 2021, 11(9), 567 CrossRef CAS PubMed.
- S.-H. Seo, E.-J. Kim and S.-E. Park,
et al., GC/MS-Based Metabolomics Reveals Biomarkers in Asthma Murine Model Modulated by Opuntia Humifusa, Evidence-Based Complementary Altern. Med., 2018, 2018, 1–7 CrossRef PubMed.
- Q. Shou, L. Jin, J. Lang, Q. Shan, Z. Ni, C. Cheng, Q. Li, H. Fu and G. Cao, Integration of Metabolomics and Transcriptomics Reveals the Therapeutic Mechanism Underlying Paeoniflorin for the Treatment of Allergic Asthma, Front. Pharmacol., 2019, 9, 1531 CrossRef PubMed.
- H. Li, T. Yang and R. Wu,
et al., Salidroside Inhibits Platelet–derived Growth Factor–Induced Proliferation and Migration of Airway Smooth Muscle Cells, J. Cell. Biochem., 2018, 120(4), 6642–6650 CrossRef PubMed.
- G. H. Yan and Y. H. Choi, Salidroside Attenuates Allergic Airway Inflammation Through Negative Regulation of Nuclear Factor-Kappa B and P38 Mitogen–Activated Protein Kinase, J. Pharmacol. Sci., 2014, 126(2), 126–135 CrossRef CAS PubMed.
- L. Dong, Y. Wang, T. Zheng, Y. Pu, Y. Ma, X. Qi, W. Zhang, F. Xue, Z. Shan, J. Liu, X. Wang and C. Mao, Hypoxic HUCMSC-Derived Extracellular Vesicles Attenuate Allergic Airway Inflammation and Airway Remodeling in Chronic Asthma Mice, Stem Cell Res. Ther., 2021, 12(1), 4 CrossRef CAS PubMed.
- L. Dong, Y. Wang, T. Zheng, Y. Pu, Y. Ma, X. Qi, W. Zhang, F. Xue, Z. Shan, J. Liu, X. Wang and C. Mao, Hypoxic HUCMSC-Derived Extracellular Vesicles Attenuate Allergic Airway Inflammation and Airway Remodeling in Chronic Asthma Mice, Stem Cell Res. Ther., 2021, 12(1), 4 CrossRef CAS PubMed.
- K. J. Livak and T. D. Schmittgen, Analysis of Relative Gene Expression Data Using Real-Time Quantitative PCR and the 2−ΔΔCT Method, Methods, 2001, 25(4), 402–408 CrossRef CAS PubMed.
- X. Xie, J. Liao, Y. Ai, J. Gao, J. Zhao, F. Qu, C. Xu, Z. Zhang, W. Wen, H. Cui and H. Wang, Pi-Dan-Jian-Qing Decoction Ameliorates Type 2 Diabetes Mellitus Through Regulating the Gut Microbiota and Serum Metabolism, Front. Cell. Infect. Microbiol., 2021, 11, 748872 CrossRef CAS PubMed.
- X. Xie, J. Liao, Y. Ai, J. Gao, J. Zhao, F. Qu, C. Xu, Z. Zhang, W. Wen, H. Cui and H. Wang, Pi-Dan-Jian-Qing Decoction Ameliorates Type 2 Diabetes Mellitus Through Regulating the Gut Microbiota and Serum Metabolism, Front. Cell. Infect. Microbiol., 2021, 11, 748872 CrossRef CAS PubMed.
- X. Jing, W. Yan and W. Cheng,
et al., Qingfei Oral Liquid Downregulates TRPV1 Expression to Reduce Airway Inflammation and Mucus Hypersecretion Injury Caused by Respiratory Syncytial Virus Infection and Asthma in Mice, Tradit. Med. Res., 2020, 5(4), 229–237 CAS.
- H. Li, T. Yang and R. Wu,
et al., Salidroside Inhibits Platelet–derived Growth Factor–Induced Proliferation and Migration of Airway Smooth Muscle Cells, J. Cell. Biochem., 2018, 120(4), 6642–6650 CrossRef PubMed.
- S. R. Jahromi, P. A. Mahesh and B. S. Jayaraj,
et al., Serum Levels of IL-10, IL-17F and IL-33 in Patients with Asthma: A Case–Control Study, J. Asthma, 2014, 51(10), 1004–1013 CrossRef PubMed.
- P. A. J. Henricks and F. P. Nijkamp, Reactive Oxygen Species as Mediators in Asthma, Pulm. Pharmacol. Ther., 2001, 14(6), 409–421 CrossRef CAS PubMed.
- P. Kleniewska and R. Pawliczak, The Participation of Oxidative Stress in the Pathogenesis of Bronchial Asthma, Biomed. Pharmacother., 2017, 94, 100–108 CrossRef CAS PubMed.
- L. G. Wood, D. A. Fitzgerald and P. C. Gibson,
et al., Lipid Peroxidation as Determined by Plasma Isoprostanes Is Related to Disease Severity in Mild Asthma, Lipids, 2000, 35(9), 967–974 CrossRef CAS PubMed.
- D. Tekin, B. A. Sin and D. Mungan,
et al., The Antioxidative Defense in Asthma, J. Asthma, 2000, 37(1), 59–63 CrossRef CAS PubMed.
- S. Gong, X. Ji and J. Su,
et al., Yeast Fermentate Prebiotic Ameliorates Allergic Asthma, Associating with Inhibiting Inflammation and Reducing Oxidative Stress Level through Suppressing Autophagy, Mediators Inflammation, 2021, 2021, 1–13 CrossRef PubMed.
- T. R. Bai and D. A. Knight, Structural Changes in the Airways in Asthma: Observations and Consequences, Clin. Sci., 2005, 108(6), 463–477 CrossRef PubMed.
- L. Dong, S.-J. Wang and B. Camoretti-Mercado,
et al., FIZZ1 Plays a Crucial Role in Early Stage Airway Remodeling of OVA-Induced Asthma, J. Asthma, 2008, 45(8), 648–653 CrossRef CAS PubMed.
- D. Cataldo, C. Munaut and A. Noël,
et al., MMP-2- and MMP-9-Linked Gelatinolytic Activity in the Sputum from Patients with Asthma and Chronic Obstructive Pulmonary Disease, Int. Arch. Allergy Appl. Immunol., 2000, 123(3), 259–267 CrossRef CAS PubMed.
- Z.-H. Qu, Z.-C. Yang and L. Chen,
et al., Inhibition Airway Remodeling and Transforming Growth Factor-B1/Smad Signaling Pathway by Astragalus Extract in Asthmatic Mice, Int. J. Mol. Med., 2011, 29(4), 564–568 CrossRef PubMed.
- O. Kuda, M. Brezinova and M. Rombaldova,
et al., Docosahexaenoic Acid–Derived Fatty Acid Esters of Hydroxy Fatty Acids (FAHFAs) With Anti-Inflammatory Properties, Diabetes, 2016, 65(9), 2580–2590 CrossRef CAS PubMed.
- S. G. B. Gowda, H. Fuda and T. Tsukui,
et al., Discovery of Eicosapentaenoic Acid Esters of Hydroxy Fatty Acids as Potent Nrf2 Activators, Antioxidants, 2020, 9(5), 397 CrossRef CAS PubMed.
- W. S. Faraci, A. A. Nagel and K. A. Verdries,
et al., 2-Amino-4-Methylpyridine as a Potent Inhibitor of Inducible NO Synthase Activity in Vitro and in Vivo, Br. J. Pharmacol., 1996, 119(6), 1101–1108 CrossRef CAS PubMed.
- H. Zhu, D. Pi and W. Leng,
et al., Asparagine Preserves Intestinal Barrier Function from LPS-Induced Injury and Regulates CRF/CRFR Signaling Pathway, Innate Immun., 2017, 23(6), 546–556 CrossRef CAS PubMed.
- C. T. Peterson, D. A. Rodionov and A. L. Osterman,
et al., B Vitamins and Their Role in Immune Regulation and Cancer, Nutrients, 2020, 12(11), 3380 CrossRef CAS PubMed.
- T. M. Hagen, J. Liu and J. Lykkesfeldt,
et al., Feeding Acetyl- l -Carnitine and Lipoic Acid to Old Rats Significantly Improves Metabolic Function While Decreasing Oxidative Stress, Proc. Natl. Acad. Sci. U. S. A., 2002, 99(4), 1870–1875 CrossRef CAS PubMed.
- G. Paradies, F. M. Ruggiero and M. N. Gadaleta,
et al., The Effect of Aging and Acetyl-l-Carnitine on the Activity of the Phosphate Carrier and on the Phospholipid Composition in Rat Heart Mitochondria, Biochim. Biophys. Acta, Biomembr., 1992, 1103(2), 324–326 CrossRef CAS PubMed.
- N. Kurabe, T. Hayasaka and H. Igarashi,
et al., Visualization of Phosphatidylcholine (16:0/16:0) in Type II Alveolar Epithelial Cells in the Human Lung Using Imaging Mass Spectrometry, Pathol. Int., 2013, 63(4), 195–200 CrossRef CAS PubMed.
- H. M. Ko, N. I. Kang and Y. S. Kim,
et al., Glutamine Preferentially Inhibits T-Helper Type 2 Cell-Mediated Airway Inflammation and Late Airway Hyperresponsiveness through the Inhibition of Cytosolic Phospholipase A2 Activity in a Murine Asthma, Clin. Exp. Allergy, 2007, 38(2), 357–364 CrossRef PubMed.
- Y.-M. Kim, J. Kim and H.-K. Cheong,
et al., Exposure to Phthalates Aggravates Pulmonary Function and Airway Inflammation in Asthmatic Children, PLoS One, 2018, 13(12), e0208553 CrossRef PubMed.
- E. B. Lugli, R. Correia and R. Fischer,
et al., Expression of Citrulline and Homocitrulline Residues in the Lungs of Non-Smokers and Smokers: Implications for Autoimmunity in Rheumatoid Arthritis, Arthritis Res. Ther., 2015, 17(1), 9 CrossRef PubMed.
- Y. Luo, H. Chen and R. Huang,
et al., Guanosine and Uridine Alleviate Airway Inflammation via Inhibition of the MAPK and NF-KB Signals in OVA-Induced Asthmatic Mice, Pulm. Pharmacol. Ther., 2021, 69, 102049 CrossRef CAS PubMed.
- M. S. Vermillion, R. L. Ursin and S. E. Attreed,
et al., Estriol Reduces Pulmonary Immune Cell Recruitment and Inflammation to Protect Female Mice From Severe Influenza, Endocrinology, 2018, 159(9), 3306–3320 CrossRef PubMed.
- S. Murugan, P. Jakka and S. Namani,
et al., The Neurosteroid Pregnenolone Promotes Degradation of Key Proteins in the Innate Immune Signaling to Suppress Inflammation, J. Biol. Chem., 2019, 294(12), 4596–4607 CrossRef CAS PubMed.
- D. C. Newcomb, J. Y. Cephus and M. G. Boswell,
et al., Estrogen and Progesterone Decrease Let-7f MicroRNA Expression and Increase IL-23/IL-23 Receptor Signaling and IL-17A Production in Patients with Severe Asthma, J. Allergy Clin. Immunol., 2015, 136(4), 1025–1034 CrossRef CAS PubMed.
- F. Farah and K. Fereshteh, Histophatologic changes of lung in asthmatic male rats treated with hydro-alcoholic extract of Plantago major and theophylline, Avicenna J. Phytomed., 2013, 3(2), 143 Search PubMed.
- W. Zhou, X. Mo, W. Cui, W. Zhang, D. Li and L. Li,
et al., Nrf2 inhibits epithelial-mesenchymal transition by suppressing snail expression during pulmonary fibrosis, Sci. Rep., 2016, 6, 38646 CrossRef CAS PubMed.
- Y. C. Lee, K. H. Lee, H. B. Lee and Y. K. Rhee, Serum Levels of Interleukins (IL)-4, IL-5, IL-13, and Interferon-γ in Acute Asthma, J. Asthma, 2001, 38, 665–671 CrossRef CAS PubMed.
|
This journal is © The Royal Society of Chemistry 2023 |
Click here to see how this site uses Cookies. View our privacy policy here.