DOI:
10.1039/D1FO03034E
(Paper)
Food Funct., 2022,
13, 290-303
Dietary gamma-aminobutyric acid ameliorates growth impairment and intestinal dysfunction in turbot (Scophthalmus maximus L.) fed a high soybean meal diet†
Received
10th September 2021
, Accepted 22nd November 2021
First published on 23rd November 2021
Abstract
Over-substitution of fishmeal with soybean meal (SBM) commonly induces inferior growth and intestinal dysfunction in fish. This study aims to evaluate whether dietary gamma-aminobutyric acid (GABA) could ameliorate the adverse effects in turbot fed a high-SBM diet (HSD). Two hundred and seventy turbots were randomly divided into three treatment groups including turbots fed on a control diet (CNT, containing 60% fishmeal), an HSD (with 45% fishmeal protein replaced by SBM), and an HSD supplemented with GABA (160 mg kg−1) for 53 days. The growth and feed utilization parameters were calculated and the intestinal antioxidant status, inflammation, apoptosis, and microbiota were evaluated using assay kits, histological analysis, qRT-PCR, high throughput sequencing, and bioinformatics analysis. The results showed that GABA ameliorated HSD-induced growth impairment and enhanced feed intake of turbot. GABA ameliorated HSD-induced intestinal oxidative stress and apoptosis by restoring the MDA content, CAT and T-AOC activities, and apoptosis-related gene (Bcl-2, Bax, Bid, and Caspase-3) expressions to similar levels to those in the CNT group. GABA also alleviated HSD-induced intestinal inflammation through down-regulating the expressions of TNF-α, IL-1β, and NF-κB p65 and up-regulating the expression of TGF-β1. Furthermore, GABA reversed HSD-induced microbiota dysbiosis through regulating the overall bacterial richness and dominative bacterial population. Spearman's correlation analysis indicated that the altered microbiota was closely associated with growth and intestinal function. Collectively, GABA could ameliorate HSD-induced intestinal dysfunction via relieving oxidative stress, inflammation, apoptosis and microbiota dysbiosis, and these findings would contribute to a better understanding of the function of GABA in the fish intestine.
1 Introduction
Sustainable aquaculture is essential for future food security, which can provide a high-quality protein source for the fast-growing human population. Reducing the dependency of the aquafeed industry on fishmeal is one of the big objectives for sustainable aquaculture development. Soybean meal (SBM) has long been the principal alternative protein source to fishmeal in aquafeed,1,2 however, fish (especially carnivorous fish) are sensitive to the amount of SBM in feed, and excessive addition can damage their growth and health.3–5 Specifically, the intestinal tract of fish has the largest surface area exposed to diet and is the main target organ for various stressors.6 Accumulating studies found that improper addition of SBM in diets can cause intestinal dysfunctions in fish, including oxidative stress injury, uncontrolled inflammation and apoptosis, intestinal mucosal barrier damage, and microbiota disorder.3–5,7,8 Intestinal dysfunction has become an obstacle to the application of the SBM-based diet and the development of healthy aquaculture. Appropriate strategies are required to protect intestinal function and maintain intestinal homeostasis in fish fed with SBM-based or other plant protein-based diets.
Gamma-aminobutyric acid (GABA) is an important functional non-protein amino acid with high bioactivity and an important inhibiting neurotransmitter that widely exists in bacteria, plants, and vertebrates.9,10 GABA plays multiple roles in relieving stress and inflammation, regulating apoptosis, lowering blood pressure, etc.11–14 Also, GABA performs many nutritional and immunoregulatory functions in poultry and livestock animals. For instance, dietary GABA supplementation could alleviate heat stress-induced inhibitory effects on digestive enzymes, absorption and immune functions, and antioxidant function in chicken.15,16 Chen et al. reported that GABA supplementation could increase the amino acid content of the ileal mucosa, enhance the intestinal immunity, and modulate the gut microbiota in weaning piglets.17 Previous studies indicated the positive role of GABA in regulating the intestinal function of cultured animals and provided inspiration for the present study. Recently, some studies also evaluated the effects of dietary GABA on several fish species. Wu et al. indicated that GABA supplementation (87.5 mg kg−1) improved growth performance and antioxidative status, and up-regulated the expression of the feeding-related genes in grass carp (Ctenopharyngodon idellus).18 Temu et al. indicated that the growth performance, superoxide dismutase activity, and serum total protein in juvenile Nile tilapia (Oreochromis niloticus) were remarkably improved via GABA supplementation (75 mg kg−1) with inactivated Lactobacillus paracasei.19 Bae et al. found that dietary GABA (158 mg kg−1) enhanced growth performance, intestinal trypsin activity, non-specific immunity, and disease resistance in olive flounder (Paralichthys olivaceus).20 The abovementioned findings suggested the beneficial influences of GABA on fish and provided some supportive data for the dosage of GABA as a dietary supplement in aquaculture. But taken together, studies regarding the influence of GABA on the intestinal function of fish are still scarce, and the underlying regulatory mechanisms remain elusive.
Turbot (Scophthalmus maximus L.) is a typical marine carnivorous fish with high sensitivity to protein sources.21 In the present study, turbot was chosen as the model species to verify whether dietary GABA supplementation can alleviate the adverse effects induced by a high-SBM diet in carnivorous fish. A comprehensive assessment was performed by measuring the growth performance, antioxidative status, intestinal histology, intestinal inflammation and apoptosis, and intestinal microbiota. This study aims to provide data support for the functions and mechanisms of GABA as a dietary supplement in aquaculture and a solution to intestinal health problems in fish fed plant protein-based diets.
2 Materials and methods
2.1 Experimental diets and feeding trial
The gamma-aminobutyric acid (GABA) used in the present study was provided by Shijitianlong Biotech Co. Ltd (Tianjin, China). Three isonitrogenic and isoenergetic experimental diets were formulated (Table 1): a control diet (CNT, containing 60% fishmeal), a high SBM diet (HSD, with 45% fishmeal protein replaced by SBM), and an HSD supplemented with 160 mg kg−1 GABA (HSDG). GABA was added to the diet with microcrystalline cellulose as a carrier, and the dose for GABA supplementation was selected according to a previous study in olive flounder.20 Crystalline amino acids (lysine and methionine) were supplemented to meet the requirements of juvenile turbot. Raw feed materials were ground and passed through 177 μm sieves, weighed and mixed gradually, and then blended with oil and water. Granular feeds (3 mm × 4 mm) were automatically made using a pelletizer, and then oven-dried for 14 h at 55 °C. All diets were sealed and stored at −20 °C until required. The proximate compositions (moisture, crude protein, crude lipid, ash, and gross energy) of the diets were analyzed using AOAC methods.22 Moisture was analyzed by drying the samples at 105 °C to a constant weight; crude protein was determined using the Kjeldahl method by multiplying the nitrogen content by 6.25; crude lipid was measured by petroleum ether extraction by the Soxhlet method; ash was examined using a muffle furnace at 550 °C for 20 h; gross energy was determined with a Parr1281 automatic bomb calorimeter (Parr, Moline, IL, USA).
Table 1 Formulas and proximate composition of the experimental diets
|
Treatments |
CNT |
HSD |
HSDG |
CNT – the control diet containing 60% fishmeal; HSD – the high-soybean meal diet with 45% fishmeal protein replaced by soybean meal; HSDG – the HSD diet supplemented with 160 mg kg−1 GABA. Mineral premix (g kg−1 diet): MgSO4·7H2O, 1.20; FeSO4·H2O, 0.08; CoCl2·6H2O, 0.05; ZnSO4·H2O, 0.05; MnSO4·H2O, 0.045; Na2SeO3, 0.02; CuSO4·5H2O, 0.01; Ca (IO3)2·6H2O, 0.06; zeolite, 8.485. Vitamin premix (g kg−1 diet): VA, 0.032; VE, 0.24; VK, 0.01; VD3, 0.005; VB1, 0.025; VB2, 0.045; VB6, 0.02; VB12, 0.01; D-calcium pantothenate, 0.06; amine nicotinic acid, 0.20; folic acid, 0.02; VH, 0.06; mesoinositol, 0.80; VC (35%), 2.00. Others (g kg−1 diet): Ca(H2PO4)2, 10.00; soy lectithin, 20.00; attractant, 10.00; choline chloride, 3.00; sodium alginate, 5.00; yttrium oxide, 1.00; calcium propionic acid, 0.50; taurine, 10.00; ethoxyquin, 0.50. |
Ingredients (g kg
−1
diet)
|
Fish meal |
600.00 |
330.00 |
330.00 |
Soybean meal |
— |
378.70 |
378.70 |
Wheat meal |
242.80 |
59.10 |
59.10 |
Wheat gluten meal |
21.20 |
63.40 |
63.40 |
Fish oil |
46.00 |
72.00 |
72.00 |
Amino acid |
— |
6.70 |
6.70 |
Mineral premixa |
10.00 |
10.00 |
10.00 |
Vitamin premixb |
3.53 |
3.53 |
3.53 |
Microcrystalline cellulose |
16.47 |
16.47 |
16.31 |
GABA |
— |
— |
0.16 |
Othersc |
60.00 |
60.00 |
60.00 |
Proximate composition
|
Crude protein (g kg−1 diet) |
501.20 |
498.90 |
499.60 |
Gross energy (KJ g−1 diet) |
19.76 |
20.13 |
20.25 |
Crude lipid (g kg−1 diet) |
129.80 |
131.30 |
132.70 |
Juvenile turbots were purchased from a local farm (Qingdao, China). The fish were acclimated in a flowing water system and fed with commercial feed for 2 weeks prior to the feeding trial. After a 24 h fasting, 270 healthy turbots with even size (13.50 ± 0.13 g) were randomly allocated to 9 tanks (three tanks per dietary treatment, 30 fish per 300 L tank). Fish were hand-fed twice a day (7:00 a.m. and 6:00 p.m.) to apparent satiation for 53 days. The feces were cleaned using a siphoning tube prior to feeding, and about 50% of water was changed every day to maintain the water quality. During the trial period, the aquatic environmental parameters were as follows: water temperature 18–21 °C, salinity 24–27‰, ammonia-nitrogen <0.2 mg L−1, nitrite <0.1 mg L−1, and dissolved oxygen 6–8 mg L−1.
2.2 Sample collection
At the end of the 53 d feeding trial, fish from each tank were counted and group weighed after 24 h of fasting, and anesthetized with eugenol (1
:
10
000, Shanghai Reagent Co., Shanghai, China). Blood of six fish from each tank was taken from the caudal veins using disposable sterile syringes and centrifuged at 4000 g for 10 min at 4 °C, and then the plasma aliquots were stored at −80 °C until use for the detection of cytokine contents. The intestines of nine fish per tank were taken out; the contents were removed, and rinsed at least three times with sterilized normal saline. After that, the entire intestines of three fish were collected for the detection of antioxidant parameters. The distal intestines of three fish were collected for quantitative real-time PCR (qRT-PCR) analysis. Meanwhile, the distal intestines from the remaining three fish were sampled and immersed in 4% paraformaldehyde for histological evaluation. As for microbiome analysis, the entire intestinal contents of another six fish from each tank were aseptically collected and combined into one sample, and thus three samples per group were obtained. All the samples of intestinal tissues and contents for antioxidant parameter detection, qRT-PCR analysis and microbiome analysis were instantly frozen in liquid nitrogen, and then stored at −80 °C.
2.3 Detection of plasma cytokine contents and intestinal antioxidant parameters
The contents of cytokines tumor necrosis factor-alpha (TNF-α), interleukin-1 beta (IL-1β) and transforming growth factor-beta1 (TGF-β1) in the plasma were measured using double antibody sandwich enzyme-linked immunosorbent assay (ELISA) with kits purchased from Cusabio (Wuhan, China) and the results were presented as pg per mL plasma. The collected samples of entire intestines were thawed and homogenized in sterilized normal saline, and then centrifuged at 2500 rpm for 10 min at 4 °C. The supernatants were collected for the determination of protein concentration by the Coomassie Brilliant Blue method using kits from Jiancheng Bioengineering Institute (Nanjing, China). The activities of superoxide dismutase (SOD), total antioxidant capacity (T-AOC), and catalase (CAT) were detected by enzymatic colorimetric methods using kits purchased from Jiancheng Bioengineering Institute (Nanjing, China). The malondialdehyde (MDA) level was determined by the thiobarbituric acid (TBA) method. Briefly, the prepared supernatants from samples were blended with sodium dodecyl sulfate, acetate buffer (pH 3.5), and TBA solution. The mixture was heated (95 °C for 40 min), centrifuged (4000 g for 10 min), and then the absorbance of the supernatant was assayed at 523 nm. The results of enzyme activity and MDA level were presented as U per mg protein and nmol per mg protein, respectively.
2.4 Intestinal histological analysis
The distal intestinal samples were fixed and transferred into 70% alcohol. Then, approximately 1 cm-length fixed tissues were collected, routinely dehydrated with gradient alcohol and embedded in paraffin wax. Approximately 5 micron-thick tissues were cut with a microtome (Leica, RM2016, Germany), installed onto glass slides and then stained using hematoxylin and eosin (H&E). The slides were photographed with a light microscope (Olympus, DP72) equipped with CellSens Standard Software (Olympus) and a camera (Nikon E600). The villus height (VH), muscular layer thickness (MLT), and lamina propria width (LPW) were measured and analyzed to visually evaluate the gut health of fish fed different diets.
2.5 qRT-PCR analysis
The relative mRNA expressions of antioxidant genes (SOD, CAT, and glutathione peroxidase (GSH-Px)), nuclear transcription factor-κB p65 (NF-κB p65), pro-inflammatory cytokines (TNF-α and IL-1β), anti-inflammatory cytokine TGF-β1, anti-apoptosis gene B-cell lymphoma-2 (Bcl-2), and pro-apoptosis genes (BH3-interacting domain death agonist (Bid), Bcl-2-associated X protein (Bax) and Caspase-3) were examined by qRT-PCR to explore the possible molecular mechanism behind the intestinal function changes. Total RNA of each distal intestine (∼50 mg) was extracted using RNAiso Plus purchased from Takara Biotech Co. Ltd (Dalian, China). Electrophoresis on 1.2% agarose gel was performed to measure the RNA integrity, and a Nano Drop®2000 spectrophotometer (Thermo Fisher Scientific, USA) was used to determine the RNA concentration. Then, the high-quality RNA was reverse transcribed into cDNA with the Primer Script™ RT reagent kit (Takara, Japan). All the primers were synthesized by Sangon Biotech. (Shanghai, China) and are presented in Table 2. The qRT-PCR was carried out in a quantitative thermal cycler (Mastercycler ep realplex, Eppendorf, Germany) following the program: 95 °C for 20 s, followed by 39 cycles of 95 °C for 5 s, 57–58 °C for 30 s, and 72 °C for 30 s. The relative quantitative method (2−ΔΔCT)23 was used to detect the gene expression level using β-actin as the housekeeping gene for sample normalization.
Table 2 Sequences of primers used in the present study for qRT-PCR
Target gene |
Forward primer (5′–3′) |
Reverse primer (5′–3′) |
Genbank number |
SOD – superoxide dismutase; CAT – catalase; GSH-Px – glutathione peroxidase; NF-κB p65 – nuclear transcription factor-κB p65; TNF-α – tumor necrosis factor-α; IL-1β – interleukin-1β; TGF-β1 – transforming growth factor-β1; Bcl-2 – B-cell lymphoma-2; Bid – BH3-interacting domain death agonist; Bax – Bcl-2-associated X protein. |
SOD
|
AAACAATCTGCCAAACCTCTG |
CAGGAGAACAGTAAAGCATGG |
MG253620.1 |
CAT
|
TATCTTCGTCCGCACTGT TG |
AGAAACCCAGCCTCACTTTG |
MG253621.1 |
GSH-Px
|
CCCTGATGACTGACCCAAAG |
GCACAAGGCTGAGGAGTT TC |
AWP02885.1 |
NF-κB p65
|
ACACTGCTGAGCTGAAGATC |
CTCTGAGCCCATCAGGGTC |
MF370855 |
TNF-α
|
CCCTTATCATTATGGCCCTT |
TCCGAGTACCGCCATATCCT |
FJ654645.1 |
IL-1β
|
TACCTGTCGTGCCAACAGGAA |
TGATGTACCAGTTGGGGAA |
AJ295836.2 |
TGF-β1
|
CTGCAGGACTGGCTCAAAGG |
CATGGTCAGGATGTATGGTGGT |
KU238187 |
Bcl-2
|
TTCCTCAACTCTCAAAGCACAATTC |
ATTACACTCGCTCGCCATTCC |
MN782168 |
Bid
|
TCTGGTGCTCCTTTCCTT |
CTTTGTCCGTCGGTTTCT |
AWP08813.1 |
Bax
|
AGCATCTTTGCTGACGGGAT |
GCGCTCTCTGATGACCTGAA |
MN782169 |
Caspase-3
|
TTCTGCCATTGTCTCTGTGC |
GCCCTGCAACATAAAGCAAC |
JU391554.1 |
β-Actin
|
GCTGTCTTCCCTTCTATCGTCG |
TCCATGTCATCCCAGTTGGTC |
AY008305.1 |
2.6 Genomic DNA extraction, PCR amplification and high throughput sequencing
For the intestinal microbiota analysis, total genomic DNA was extracted from intestinal content samples by the CTAB method. The high-quality DNA with an appropriate concentration (1 ng μL−1) and superior integrity was used for the amplification of the 16S V4 hypervariable regions using specific primer pair 515F (5′-GTGCCAGCMGCCGCGG-3′)/806R (5′-GGACTACHVGGGTWTCTAAT-3′) with the barcode by Phusion® High-Fidelity PCR Master Mix (New England Biolabs). The amplification program was performed following the description in a previous study.5 The pooled PCR products from all samples were purified using the Qiagen Gel Extraction Kit (Qiagen, Germany). Sequencing libraries were constructed using the TruSeq® DNA PCR-Free Sample Preparation Kit (Illumina, USA) with the addition of index codes. The Qubit@ 2.0 fluorometer (Thermo Scientific) and Agilent 2100 Bioanalyzer system were used to evaluate the library quality. Finally, the library was sequenced on an Illumina NovaSeq platform and 250 bp paired-end reads were generated. The raw sequence data reported in this paper have been deposited in the Genome Sequence Archive (Genomics, Proteomics & Bioinformatics 2021) in the National Genomics Data Center (Nucleic Acids Res 2021), China National Center for Bioinformation/Beijing Institute of Genomics, Chinese Academy of Sciences, under accession number PRJCA005828 that are publicly accessible at https://ngdc.cncb.ac.cn/gsa.
2.7 Data processing and bioinformatics analysis
The barcode and primer sequence of paired-end reads were removed and then merged using FLASH (v1.2.7).24 Clean tags with high quality were obtained through quality filtering performed under specific filtering conditions based on QIIME (v1.9.1).25 The tags were compared with the reference database (Silva database)26 using the UCHIME algorithm27 to detect chimera sequences, and then the chimera sequences were removed.28 The effective tags were ultimately obtained for other OTU clusters (≥97% similarity) using Uparse software (v7.0.1001).29 Representative sequence of each OTU was screened for further annotation of taxonomic information using the Silva Database (Version 132)26 based on the Mothur algorithm. The phylogenetic relationship construction was performed using MUSCLE software (v3.8.31),30 and the abundance information of OTUs was normalized with a standard sequence number corresponding to the sample with the least sequences. Alpha diversity indices (ACE, Chao1, Shannon, Simpson) and beta diversity were calculated using QIIME (v1.7.0) and displayed using R software (v2.15.3). Beta diversity analysis, including non-metric multi-dimensional scaling (NMDS) and principal co-ordinate analysis (PCoA) based on weighted unifrac distances, were included to compare the microbiome composition similarity of different samples. NMDS was displayed using the vegan package, and PCoA using the WGCNA package, stats packages and ggplot2 package in R software (v2.15.3). Statistical differences of alpha diversity and beta diversity between treatments were determined using Tukey's test and wilcoxon's test. For the environmental factor correlation analysis, the corr. test function of the psych package in R software (v2.15.3) was used to calculate the Spearman correlation values of species and environmental factors and test their significance. Then, the pheatmap function in the pheatmap package was used for visualization.
2.8 Calculations and statistical analysis
The following variables were calculated:
Data were analyzed by one-way analysis of variance (ANOVA) followed by Tukey's test using SPSS 22.0. The results were presented as mean ± standard error and p < 0.05 was considered statistically significant.
3 Results
3.1 Growth and feed utilization
As shown in Fig. 1, at the end of the 53 day feeding trial, no significant differences were found in the SR among groups (p > 0.05). Final body weight (FBW), SGR, FER, and PER were significantly reduced in the HSD-fed group compared to the CNT group (p < 0.05), however, no significant reductions in growth and feed utilization performance were found in the HSDG group (p > 0.05). In particular, turbot in the HSDG group had significantly higher FI than those in CNT and HSD-fed groups. These results indicated that dietary GABA effectively ameliorated HSD-induced growth impairment and enhanced feed intake in turbot.
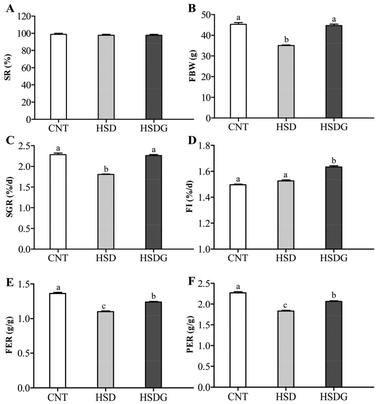 |
| Fig. 1 Growth and feed utilization parameters of turbot. A – Survival rate (SR); B – final body weight (FBW); C – specific growth rate (SGR); D – feed intake (FI); E – feed efficiency ratio (FER); and F – protein efficiency ratio (PER). Values are displayed as mean ± standard error (n = 3). Different letters (a, b, c) above the bars represent statistical differences based on Tukey's test (p < 0.05, one-way ANOVA). | |
3.2 Antioxidant status and related gene expression
The HSDG group had the highest SOD activity, followed by the CNT group, and the lowest in the HSD-fed group (Fig. 2A), although no significant statistical differences were obtained (p > 0.05). HSD remarkably reduced the CAT and T-AOC activities, and increased the MDA level (p < 0.05, Fig. 2B–D), while the HSDG group showed no significant differences in these parameters compared to the CNT group (p > 0.05, Fig. 2B–D). Furthermore, the expression of genes involved in antioxidant mechanisms (SOD, CAT, and GSH-Px) was determined. As shown in Fig. 2E, the HSDG group showed the highest expression of SOD among groups (p < 0.05). HSD significantly reduced the expression of CAT (p < 0.05), while the HSDG group showed a similar level to that in the CNT group (p > 0.05). In addition, no significant differences were observed in the expression of GSH-Px among the three groups. These results indicated that dietary GABA could alleviate oxidative stress induced by HSD in turbot.
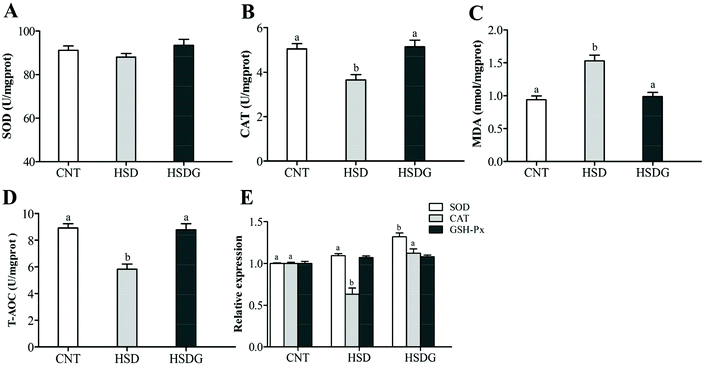 |
| Fig. 2 Antioxidant enzyme activity and related gene expression in the intestine of turbot. A – Superoxide dismutase (SOD); B – catalase (CAT); C – malondialdehyde (MDA); D – the total antioxidant capacity (T-AOC); and E – the mRNA expressions of SOD, CAT and glutathione peroxidase (GSH-Px). Values are displayed as means ± standard error (n = 6). Different letters (a, b) above the bars represent statistical differences based on Tukey's test (p < 0.05, one-way ANOVA). | |
3.3 Apoptosis-related gene expression
The expressions of anti-apoptosis gene Bcl-2 and pro-apoptosis genes (Bax, Bid, and Caspase-3) were determined. It indicated that the expression levels of all the selected apoptosis-related genes were significantly up-regulated in the HSD-fed group compared to the CNT group (p < 0.05, Fig. 3). However, the HSDG group showed significantly lower expression of these apoptosis-related genes than the HSD-fed group (p < 0.05) and had a more similar expression to the CNT group. The finding indicated that dietary GABA could effectively alleviate the SBM-induced excessive apoptosis in the turbot intestine.
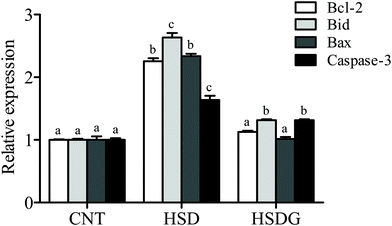 |
| Fig. 3 Apoptosis-related gene expression in the intestine of turbot. Values are displayed as mean ± standard error (n = 6). Different letters (a, b, c) above the bars represent statistical differences based on Tukey's test (p < 0.05, one-way ANOVA). | |
3.4. Gut micromorphology and inflammation-related gene expression
As shown in Fig. 4A, turbot in the CNT group showed an integrated epithelial structure, tight villus, and no significant symptoms of inflammation. However, the HSD-fed group presented remarkable histopathological disruption and typical features of intestinal inflammation (Fig. 4B), including significantly decreased VH and MLT, and increased LPW compared with the CNT group (p < 0.05, Fig. 4D–F). Nevertheless, the HSDG group alleviated HSD-induced intestinal histopathological disruption with significantly fewer inflammatory alterations (Fig. 4C), higher VH and MLT, and lower LPW than the HSD-fed group (p < 0.05, Fig. 4D–F). In parallel with the histomorphological symptoms, the mRNA expressions of pro-inflammatory (TNF-α and IL-1β) and anti-inflammatory (TGF-β1) cytokines were remarkably up-regulated or down-regulated, respectively, in the HSD-fed group (p < 0.05), while the mRNA expressions of the cytokines in the HSDG group remained at a similar level to those in the CNT group (p > 0.05, Fig. 4G). The contents of the abovementioned cytokines in the plasma of turbot fed the three diets showed the same tendency (ESI Table 1†). Furthermore, the mRNA expression of NF-κB p65 was significantly up-regulated in the HSD-fed group (p < 0.05). However, no significant differences were observed in the HSDG group compared to the CNT group (p > 0.05, Fig. 4G). These results indicated that HSD induced intestinal pathological disruption and inflammation, while dietary GABA could effectively alleviate such adverse effects.
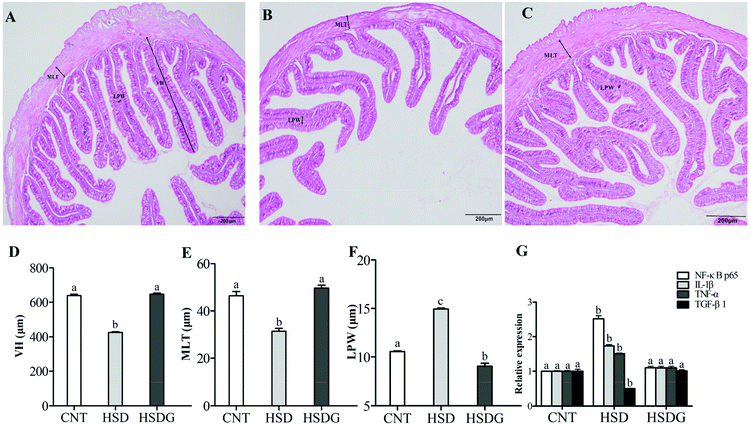 |
| Fig. 4 Intestinal micromorphology analysis and inflammation-related gene expression in turbot fed on experimental diets. A – Typical image of the CNT group; B – typical image of the HSD-fed group; C – typical image of the HSDG group; D – villus height (VH) of the turbot intestine; E – muscular layer thickness (MLT) of the turbot intestine; F – lamina propria width (LPW) of the turbot intestine; and G – inflammation-related gene expression in the turbot intestine. Values are displayed as mean ± standard error (n = 3). Different letters (a, b, c) above the bars represent statistical differences based on Tukey's test (p < 0.05, one-way ANOVA). | |
3.5 Intestinal microbiota
The intestinal microbiota alterations in turbot fed with different diets were analyzed with high throughput sequencing technology using an Illumina NovaSeq platform. Rarefaction analysis suggested that an adequate sequencing depth was attained for all samples (Fig. 5A). Also, Good's coverage for all groups was above 0.95, revealing that adequate sequencing coverage was attained (Table 3). The Venn diagram displayed that 1501 OTUs were shared by CNT, HSD, and HSDG groups, and the number of unique OTUs in CNT, HSD, and HSDG groups was 1094, 266, and 1174, respectively (Fig. 5B).
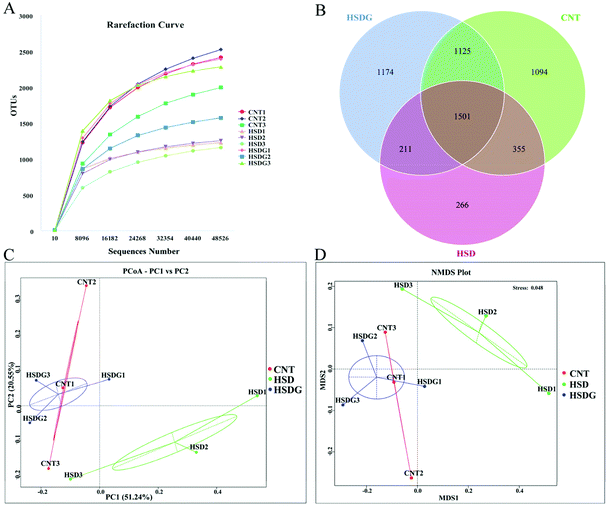 |
| Fig. 5 Comparison of intestinal microbiota profile of turbot from the three dietary groups. A – Rarefaction curves of the observed species number for all the samples. B – Venn diagram of shared and unique OTUs. Every circle in the Venn diagram represents one group. C – Principal coordinate analysis (PCoA) based on weighted UniFrac metrics. D – Non-metric multi-dimensional scaling (NMDS) analysis based on weighted UniFrac metrics. CNT1, CNT2, and CNT3 are three replicates of the CNT group; HSD1, HSD2 and HSD3 are three replicates of the HSD-fed group; HSDG1, HSDG2 and HSDG3 are three replicates of the HSDG group. | |
Table 3 Alpha diversity index of the intestinal microbiota in turbot
Treatments |
ACE |
Chao1 |
Shannon |
Simpson |
Goods_coverage |
Values are displayed as mean ± standard error, n = 3; different superscripted small letters in the same column mean significant difference (p < 0.05). |
CNT |
2688.12 ± 188.82a |
2632.01 ± 209.54a |
6.84 ± 0.55 |
0.94 ± 0.02 |
0.989 ± 0.001 |
HSD |
1351.43 ± 17.40b |
1341.47 ± 19.10b |
6.17 ± 0.87 |
0.89 ± 0.05 |
0.996 ± 0.0004 |
HSDG |
2255.32 ± 102.37a |
2254.17 ± 109.95a |
7.47 ± 0.56 |
0.96 ± 0.01 |
0.993 ± 0.001 |
Bacterial richness and diversity of turbot in the three groups were compared via the analysis of alpha diversity indices. As shown in Table 3, the bacterial richness indices of ACE and Chao1 were significantly decreased in the HSD-fed group compared to the CNT group (p < 0.05), however, no significant decrease was observed in the HSDG group (p > 0.05). As for the bacterial diversity indices of Shannon and Simpson, no significant differences were observed among the three groups (p > 0.05). Moreover, beta diversity analysis of PCoA (Fig. 5C) and NMDS (Fig. 5D) showed clear clusters of microbial samples from the three groups, indicating that the intestinal microbiota profile was remarkably regulated by experimental diets. Interestingly, both PCoA and NMDS showed that the clusters of the HSDG group were more similar to the CNT group, and distinctly separated from the HSD-fed group.
The specific changes of the intestinal microbiota in turbot are displayed in Fig. 6. Proteobacteria, Firmicutes, and Bacteroidetes were identified as the top three most abundant bacterial phyla in the turbot intestines from the three groups (Fig. 6A). HSD remarkably decreased the relative abundance of Firmicutes and increased those of Proteobacteria and Bacteroidetes compared with the CNT group (p < 0.05, Fig. 6B). Nevertheless, no significant differences were found in the relative abundances of the top three most abundant bacterial phyla in the HSDG group compared to the CNT group (p > 0.05, Fig. 6B). At the genus level, Pseudomonas, Leuconostoc, Lactobacillus, Cobetia, Lactococcus, Streptococcus, Pseudoalteromonas, Prevotella, Blautia, and Ruminococcus comprised the top ten dominant genera of the intestinal microbiota in turbot from the three groups (Fig. 6C). As for the top three most abundant bacterial genera, remarkable reductions in the relative abundances of Leuconostoc and Lactobacillus were observed in the HSD-fed group compared with the CNT group (p < 0.05, Fig. 6D). Nevertheless, the HSDG group displayed relatively higher abundances of Leuconostoc and Lactobacillus than the HSD-fed group, and no significant differences were found compared to the CNT group (p > 0.05, Fig. 6D). Spearman's correlation analysis showed that Firmicutes were positively associated with the growth and feed utilization parameters (SGR, FER, and PER) (Fig. 7A) and the CAT activity (Fig. 7B), and negatively associated with the mRNA expressions of Bid and Bax (Fig. 7D). Furthermore, Cyanobacteria were positively associated with the feed utilization parameters (FER and PER) (Fig. 7A) and the T-AOC activity (Fig. 7B), and negatively associated with the MDA level (Fig. 7B), the mRNA expressions of inflammation-related genes (NF-κB, p65 and TNF-α) (Fig. 7C), and the mRNA expressions of apoptosis-related genes (Bcl-2, Bax, Bid, and Caspase-3) (Fig. 7D). These results indicated that dietary GABA supplementation could positively regulate the intestinal microbiota, which was correlated closely with the growth performance, feed utilization and intestinal function of turbot.
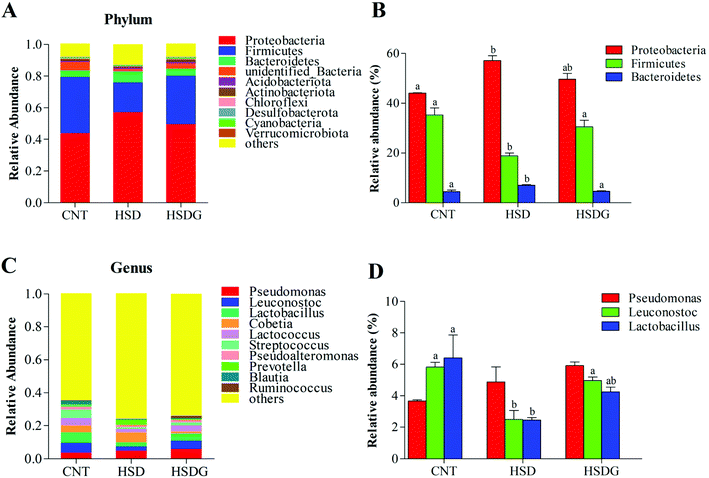 |
| Fig. 6 Specific microbiota composition of the turbot intestine. A – Relative abundance of bacterial phyla in different treatment groups. B – Top three dominant bacterial phyla in different treatment groups. C – Relative abundance of bacterial genera in different treatment groups. D – Top three dominant bacterial genera in different treatment groups. Values are displayed as mean ± standard error (n = 3). Different letters (a, b) above the bars represent statistical differences based on Tukey's test (p < 0.05, one-way ANOVA). | |
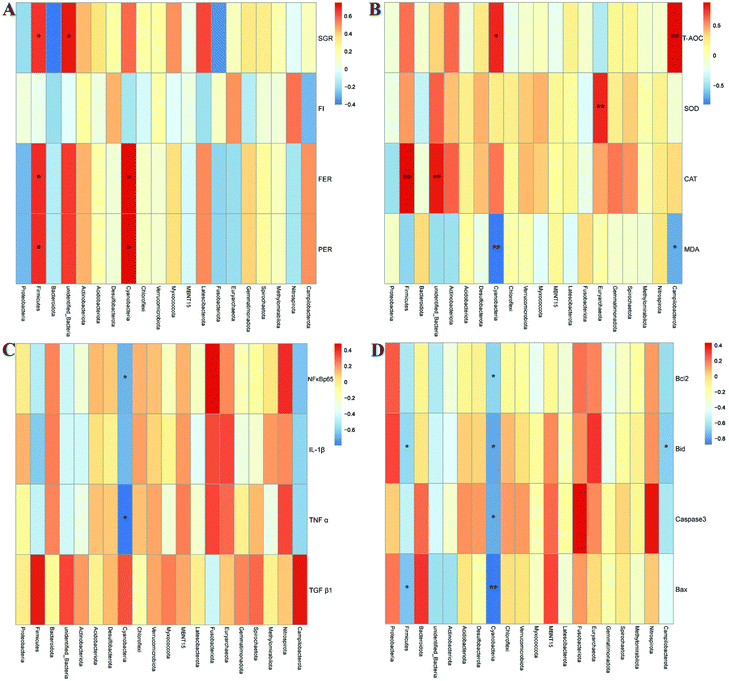 |
| Fig. 7 Environmental factor correlation analysis of turbot in different treatment groups based on Spearman correlations. A – Correlation analysis of the microbiota and the growth and feed utilization performance. B – Correlation analysis of the microbiota and the antioxidant status. C – Correlation analysis of the microbiota and the mRNA expressions of inflammation-related genes. D – Correlation analysis of the microbiota and the mRNA expressions of apoptosis-related genes. *p < 0.05; **p < 0.01. | |
4 Discussion
The effects of GABA on the growth performance of fish varied among species. In earlier studies, dietary GABA showed no effects on the growth of Japanese flounder, Paralichthys olivaceus, and even impaired the growth of the giant freshwater prawn, Macrobrachium rosenbergii.31,32 However, several recent studies reported that dietary GABA could improve growth in fish, including grass carp (Ctenopharyngodon idellus),18 Nile tilapia (Oreochromis niloticus),19 and olive flounder (Paralichthys olivaceus).20 Research into the effects of GABA on fish fed HSD is yet to be undertaken. In the present study, inferior growth and feed utilization performance were observed in turbot fed HSD, which was in line with the previous studies.5,33 However, dietary GABA ameliorated the growth impairment induced by HSD and no significant reductions of FBW, SGR, FER, and PER were observed in the HSDG group compared to the CNT group. Interestingly, turbot in the HSDG group showed significantly higher feed intake than those in the CNT and HSD-fed groups, which could be attributed to the nutritional function of GABA in enhancing appetite.34 This finding was conducive to the further application of GABA as a dietary supplement in carnivorous fish fed plant protein-based diets.
Oxidative homoeostasis in fish is easily destroyed under long-term stress, resulting in oxidative stress in the body, thus affecting the functions of various tissues and organs.35 Numerous studies have indicated that HSD could provoke an oxidative stress response in fish, including Japanese seabass, gilthead sea bream, and turbot.3,36,37 The anti-stress effects of GABA have been widely reported in mammals38–40 and several fish species,18,19 and therefore it was expected that dietary GABA could alleviate HSD-induced oxidative stress in turbot. T-AOC, SOD, and CAT, the important components of the enzymatic antioxidant system,41,42 were measured to evaluate the antioxidant status of turbot. MDA, a biochemical marker for disease progression and lipid peroxidation,43 was also measured to ascertain the severity of endogenous oxidative damage. As the results showed, severe oxidative stress with remarkably reduced T-AOC and CAT activities, decreased the expression of CAT mRNA, and an increased MDA level could be observed in the HSD-fed group. As hypothesized, better growth performance and antioxidant status in the HSDG group were observed, showing similar T-AOC and CAT activities, CAT mRNA expression, and MDA level to the CNT group. Turbot in the HSDG group even demonstrated the highest SOD activity and SOD mRNA expression among the three groups. Better performance of the antioxidant status in the HSDG group could be related to the superior ability of GABA to trap reactive intermediates or directly react with MDA during lipid peroxidation.43,44 Moreover, the findings further confirmed the antioxidant effects of dietary GABA supplementation in aquafeed.
Apoptosis is a cellular response to a multitude of triggers. The regeneration and repair of intestinal epithelial cells require a certain degree of apoptosis;45,46 however, excessive apoptosis increases intestinal permeability and induces intestinal barrier dysfunction.47 In this study, the expressions of anti-apoptosis gene Bcl-2 and pro-apoptosis genes (Bax, Bid, and Caspase-3) were examined to better reveal the possible molecular mechanism of the intestinal function changes. It indicated that the expression levels of all the selected apoptosis-related genes were significantly up-regulated in the HSD-fed group compared to the CNT group, which matched the findings in Atlantic salmon (Salmo salar L.),48 sturgeon (Acipenser schrenckii),8 and turbot.49 However, the HSDG group showed significantly lower expression of these apoptosis-related genes than the HSD-fed group, suggesting that dietary GABA could alleviate HSD-induced apoptosis in the turbot intestine. The anti-apoptosis activity of GABA can also be supported with reference to the findings of Xia et al.,14 who reported that GABA attenuated Escherichia coli-induced apoptosis in the gut epithelial cell via activating GABAAR signaling and the AMPK-autophagy pathways.
The observation of intestinal histology can intuitively reflect the intestinal health status of fish that were fed different diets. Accumulating studies have shown that HSD could destroy the intestinal structure and cause enteritis in carnivorous fish.50–52 In the present study, the HSD-fed group presented remarkable pathological disruption and typical characteristics of intestinal inflammation, including significantly decreased VH and MLT, and increased LPW compared to the CNT group; however, dietary GABA alleviated such HSD-induced intestinal pathological disruption with significantly fewer inflammatory alterations, higher VH and MLT, and lower LPW than the HSD-fed group. A recent study also revealed that dietary GABA could increase the VH in the intestine of olive flounder (Paralichthys olivaceus).20 The production of cytokines by immune cells is a key step in the establishment and maintenance of inflammatory response.53,54 The mRNA expressions of several cytokines (TNF-α, IL-1β, and TGF-β1) and the central mediator involved in inflammation development and progression, NF-κB p65,55 were measured to verify the observed phenotypic symptoms and better understand the underlying mechanisms. In parallel with the morphological symptoms, HSD increased the mRNA expressions of NF-κB p65 and pro-inflammatory cytokines (TNF-α and IL-1β) and down-regulated the mRNA expression of anti-inflammatory cytokine (TGF-β1), however, the expressions of the selected inflammation-related genes in the HSDG group remained at a similar level to those in the CNT group. A similar tendency was also found in the contents of the abovementioned cytokines in the plasma of turbot. Studies on the regulation of cytokine production by GABA in fish are extremely scarce, however, akin to the current findings, GABA supplementation inhibited the gene expressions of proinflammatory cytokines (IL-1 and IL-18) but promoted the expressions of anti-inflammatory cytokines (IL-4 and IL-10) in the intestines of weaning piglets.17 Also, GABA could increase the TGF-β production and inhibit NF-kB activation in both lymphocytes and pancreatic islet beta cells.56 Taken together, GABA supplementation might regulate cytokine production via modulating NF-κB signaling, thus attenuating intestinal inflammation in turbot.
The regulatory effects of dietary components on the intestinal microbiota in aquatic animals have attracted much attention,57,58 however, relevant studies of GABA in fish are yet to be undertaken. To better understand the underlying mechanism regarding the influence of GABA on the intestinal function, the changes in the intestinal microbiota in turbot from different dietary groups were evaluated based on an Illumina NovaSeq platform. The alpha diversity analysis suggested that the bacterial richness was significantly decreased in the HSD-fed group, however, no significant reduction was found in the HSDG group compared to the CNT group. Interestingly, both PCoA and NMDS analysis showed that CNT and HSDG clusters were relatively consistent, while the HSD cluster was evidently separated from the CNT and HSDG clusters. The aforementioned findings related to HSD-induced microbiome changes were expected, as reported in previous studies;5,33 however, GABA seemed to counteract some of the HSD-induced microbiome changes in the turbot intestine.
Although the specific compositions of intestinal microbiota varied among species, most of the research indicated that Proteobacteria, Firmicutes, Bacteroidetes, Actinobacteria, and Fusobacteria are the core groups in the intestine of marine carnivorous fish.59 In the present study, Proteobacteria, Firmicutes and Bacteroidetes were identified as the top three most abundant bacterial phyla in the turbot intestine from the three groups. As shown in the results, HSD significantly decreased the relative abundance of Firmicutes, and increased Proteobacteria and Bacteroidetes compared with the control group. The increase of Bacteroidetes was widely reported in previous studies on turbot fed an SBM-based diet.3,5,49,60 The higher content of carbohydrate in SBM can explain the enrichment of intestinal Bacteroidetes, which possesses a superior ability to degrade dietary carbohydrate.61 However, the improved utilization of carbohydrates might increase the glucose content and destroy glucose homeostasis, thus causing intestinal dysfunction in carnivorous fish62 because most carnivorous fish are intolerant to glucose.63 The HSD-induced increase of Proteobacteria was also observed in the previous study.5 The increased abundance of Proteobacteria is usually related to various human diseases.64 Although Proteobacteria is the predominant phylum in most fish species,65,66 excessive enrichment thereof may be a potential marker of microbiota dysbiosis. Interestingly, dietary GABA reversed the HSD-induced changes such that the abundance of the top three predominant phyla in the HSDG group showed similar levels to specimens in the control group. Leuconostoc and Lactobacillus are the two typical genera of the lactic acid bacteria group that produce organic acids, hydrogen peroxide or bacteriocin thus inhibiting pathogenic bacteria.67 A previous study showed that two strains of Leuconostoc mesenteroides (B7 and Z8) exhibited superior probiotic properties and could survive in an environment with low pH, high bile salts and high pepsin activity, imparting strong inhibitory effects to Listeria spp.68Lactobacillus is usually recognized as a probiotic, multiple beneficial effects of which are widely reported in many fish species including Nile tilapia (Oreochromis niloticus),69 bighead catfish (Clarias macrocephalus),70 Black Sea bream (Acanthopagrus schlegelii),71 mandarin fish (Siniperca chuatsi),72 and so on. In the present study, HSD significantly decreased the abundances of Leuconostoc and Lactobacillus, which might contribute to microbiota dysbiosis in the turbot intestine. However, turbot fed an HSDG diet showed similar abundances of Leuconostoc and Lactobacillus to that of turbot fed a control diet. This study also revealed that the shifted microbiota was closely related to the growth and feed utilization parameters, the antioxidant status, and the mRNA expressions of inflammation- and apoptosis-related genes in turbot. Taken together, the results demonstrated that GABA could partially reverse the HSD-induced intestinal microbiota dysbiosis, which may further benefit the growth performance and intestinal function in turbot.
5 Conclusions
Protecting the intestinal function in fish fed with high plant protein-based diets is the core factor for their normal growth and health. Thus, this study was conducted to evaluate the possible beneficial role and mechanism of dietary GABA in the growth and intestinal function in turbot fed an HSD. In summary, the findings indicated that dietary GABA (160 mg kg−1) could alleviate HSD-induced growth impairment and enhance the feed intake in turbot. Further research showed that dietary GABA could ameliorate HSD-induced intestinal dysfunctions via relieving oxidative stress, suppressing inflammation, and regulating apoptosis. Also, GABA could reverse the HSD-induced microbiota dysbiosis, which may greatly account for the better growth performances and intestinal function of turbot. This study confirmed the beneficial influences of GABA in turbot fed HSD, and we proposed that GABA could play multiple roles in protecting the intestinal function of fish.
Ethical statement
All animal procedures were performed in accordance with the Guidelines for Care and Use of Laboratory Animals of Shandong University of Technology and approved by the Animal Ethics Committee of Shandong University of Technology.
Author contributions
Chao-Qun Li: conceptualization, data curation, formal analysis, investigation, methodology, project administration, writing – original draft. Yuan Tian: data curation, formal analysis, investigation, methodology, project administration. Qin-Yuan Ma: funding acquisition, resources, supervision. Bei-Li Zhang: conceptualization, writing – review & editing, software, supervision, validation. All authors read and approved the final manuscript.
Conflicts of interest
The authors declare no conflict of interest.
Acknowledgements
This work was supported by the Natural Science Foundation of Shandong Province (grant no. ZR2020MC053). We thank professor Xiuzhen Gao (Shandong University of Technology) for the help in laboratory instruments and equipment. We are also grateful to professor Gen He (Ocean University of China) for the support in feeding trial and data analysis.
References
- D. M. Gatlin III, F. T. Barrows, P. Brown, K. Dabrowski, T. G. Gaylord, R. W. Hardy, E. Herman, G. Hu, A. Krogdahl, R. Nelson, K. Overturf, M. Rust, W. Sealey, D. Skonberg, E. J. Souza, D. Stone, R. Wilson and E. Wurtele, Expanding the utilization of sustainable plant products in aquafeeds: a review, Aquacult. Res., 2007, 38, 551–579 CrossRef.
- K. Hua, J. M. Cobcroft, A. Cole, K. Condon, D. R. Jerry, A. Mangott, C. Praeger, M. J. Vucko, C. Zeng, K. Zenger and J. M. Strugnell, The future of aquatic protein: implications for protein sources in aquaculture diets, One Earth, 2019, 1, 316–329 CrossRef.
- Z. Chen, S. Zhao, Y. Liu, P. Yang, Q. Ai, W. Zhang, W. Xu, Y. Zhang, Y. Zhang and K. Mai, Dietary citric acid supplementation alleviates soybean meal-induced intestinal oxidative damage and micro-ecological imbalance in juvenile turbot, Scophthalmus maximus L, Aquacult. Res., 2018, 49, 3804–3816 CrossRef CAS.
- T. J. Green, R. Smullen and A. C. Barnes, Dietary soybean protein concentrate-induced intestinal disorder in marine farmed Atlantic salmon, Salmo salar is associated with alterations in gut microbiota, Vet. Microbiol., 2013, 166, 286–292 CrossRef CAS.
- C. Li, B. Zhang, C. Liu, H. Zhou, X. Wang, K. Mai and G. He, Effects of dietary raw or Enterococcus faecium fermented soybean meal on growth, antioxidant status, intestinal microbiota, morphology, and inflammatory responses in turbot (Scophthalmus maximus L.), Fish Shellfish Immunol., 2020, 100, 261–271 CrossRef CAS.
- S. A. M. Martin, C. E. Dehler and E. Krol, Transcriptomic responses in the fish intestine, Dev. Comp. Immunol., 2016, 64, 103–117 CrossRef CAS.
- M. Coronado, C. J. Solis, P. P. Hernandez and C. G. Feijoo, Soybean meal-induced intestinal inflammation in zebrafish is T cell-dependent and has a Th17 cytokine profile, Front. Immunol., 2019, 10, 610 CrossRef CAS PubMed.
- H. C. Wei, P. Chen, X. F. Liang, H. H. Yu, X. F. Wu, J. Han, L. Luo, X. Gu and M. Xue, Plant protein diet suppressed immune function by inhibiting spiral valve intestinal mucosal barrier integrity, anti-oxidation, apoptosis, autophagy and proliferation responses in amur sturgeon (Acipenser schrenckii), Fish Shellfish Immunol., 2019, 94, 711–722 CrossRef CAS.
- M. Diana, J. Quilez and M. Rafecas, Gamma-aminobutyric acid as a bioactive compound in foods: a review, J. Funct. Foods, 2014, 10, 407–420 CrossRef CAS.
- M. Xie, H.-H. Chen, S.-P. Nie, J.-Y. Yin and M.-Y. Xie, Gamma-aminobutyric acid increases the production of short-chain fatty acids and decreases pH values in mouse colon, Molecules, 2017, 22, 653 CrossRef.
- M. Yoshimura, T. Toyoshi, A. Sano, T. Izumi, T. Fujii, C. Konishi, S. Inai, C. Matsukura, N. Fukuda, H. Ezura and A. Obata, Antihypertensive effect of a gamma-aminobutyric acid rich tomato cultivar ‘DG03–9′ in spontaneously hypertensive rats, J. Agric. Food Chem., 2010, 58, 615–619 CrossRef CAS.
- S. S. Bajic, J. Djokic, M. Dinic, K. Veljovic, N. Golic, S. Mihajlovic and M. Tolinacki, GABA-producing natural dairy isolate from artisanal zlatar cheese attenuates gut inflammation and strengthens gut epithelial barrier in vitro, Front. Microbiol., 2019, 10, 527 CrossRef.
- X. Tang, R. Yu, Q. Zhou, S. Jiang and G. Le, Protective effects of gamma-aminobutyric acid against H2O2-induced oxidative stress in RIN-m5F pancreatic cells, Nutr. Metab., 2018, 15, 60 CrossRef PubMed.
- Y. Xia, S. Chen, Y. Zhao, S. Chen, R. Huang, G. Zhu, Y. Yin, W. Ren and J. Deng, GABA attenuates ETEC-induced intestinal epithelial cell apoptosis involving GABA(A)R signaling and the AMPK-autophagy pathway, Food Funct., 2019, 10, 7509–7522 RSC.
- N. Chand, S. Muhammad, R. U. Khan, I. A. Alhidary and Z. U. Rehman, Ameliorative effect of synthetic gamma-aminobutyric acid (GABA) on performance traits, antioxidant status and immune response in broiler exposed to cyclic heat stress, Environ. Sci. Pollut. Res., 2016, 23, 23930–23935 CrossRef CAS PubMed.
- Z. Chen, J. Xie, B. Wang and J. Tang, Effect of gamma-aminobutyric acid on digestive enzymes, absorption function, and immune function of intestinal mucosa in heat-stressed chicken, Poult. Sci., 2014, 93, 2490–2500 CrossRef CAS.
- S. Chen, B. Tan, Y. Xia, S. Liao, M. Wang, J. Yin, J. Wang, H. Xiao, M. Qi, P. Bin, G. Liu, W. Ren and Y. Yin, Effects of dietary gamma-aminobutyric acid supplementation on the intestinal functions in weaning piglets, Food Funct., 2019, 10, 366–378 RSC.
- F. Wu, M. Liu, C. Chen, J. Chen and Q. Tan, Effects of dietary gamma aminobutyric acid on growth performance, antioxidant status, and feeding-related gene expression of juvenile grass carp, Ctenopharyngodon idellus, J. World Aquac. Soc., 2016, 47, 820–829 CrossRef CAS.
- V. Temu, H. Kim, A. Hamidoghli, M. Park, S. Won, M. Oh, J. Han and S. C. Bai, Effects of dietary gamma-aminobutyric acid in juvenile Nile tilapia, Orechromis niloticus, Aquaculture, 2019, 507, 475–480 CrossRef CAS.
- J. Bae, A. Hamidoghli, S. Won, W. Choi, S.-G. Lim, K.-W. Kim, B.-J. Lee, S.-W. Hur and S. C. Bai, Evaluation of seven different functional feed additives in a low fish meal diet for olive flounder, Paralichthys olivaceus, Aquaculture, 2020, 525, 735333 CrossRef CAS.
- Y. Liu, G. He, Q. Wang, K. Mai, W. Xu and H. Zhou, Hydroxyproline supplementation on the performances of high plant protein source based diets in turbot (Scophthalmus maximus L.), Aquaculture, 2014, 433, 476–480 CrossRef CAS.
-
AOAC, Official Methods of Analysis, Association of Official Analytical Chemists, Arlington, VA, 18th edn, 2006 Search PubMed.
- K. J. Livak and T. D. Schmittgen, Analysis of relative gene expression data using real-time quantitative PCR and the 2−ΔΔCT method, Methods, 2001, 25, 402–408 CrossRef CAS PubMed.
- T. Magoc and S. L. Salzberg, FLASH: fast length adjustment of short reads to improve genome assemblies, Bioinformatics, 2011, 27, 2957–2963 CrossRef CAS PubMed.
- J. G. Caporaso, J. Kuczynski, J. Stombaugh, K. Bittinger, F. D. Bushman, E. K. Costello, N. Fierer, A. G. Pena, J. K. Goodrich, J. I. Gordon, G. A. Huttley, S. T. Kelley, D. Knights, J. E. Koenig, R. E. Ley, C. A. Lozupone, D. McDonald, B. D. Muegge, M. Pirrung, J. Reeder, J. R. Sevinsky, P. J. Tumbaugh, W. A. Walters, J. Widmann, T. Yatsunenko, J. Zaneveld and R. Knight, QIIME allows analysis of high-throughput community sequencing data, Nat. Methods, 2010, 7, 335–336 CrossRef CAS.
- C. Quast, E. Pruesse, P. Yilmaz, J. Gerken, T. Schweer, P. Yarza, J. Peplies and F. O. Gloeckner, The SILVA ribosomal RNA gene database project: improved data processing and web-based tools, Nucleic
Acids Res., 2013, 41, D590–D596 CrossRef CAS PubMed.
- R. C. Edgar, B. J. Haas, J. C. Clemente, C. Quince and R. Knight, UCHIME improves sensitivity and speed of chimera detection, Bioinformatics, 2011, 27, 2194–2200 CrossRef CAS.
- B. J. Haas, D. Gevers, A. M. Earl, M. Feldgarden, D. V. Ward, G. Giannoukos, D. Ciulla, D. Tabbaa, S. K. Highlander, E. Sodergren, B. Methe, T. Z. DeSantis, J. F. Petrosino, R. Knight, B. W. Birren and C. Human Microbiome, Chimeric 16S rRNA sequence formation and detection in Sanger and 454-pyrosequenced PCR amplicons, Genome Res., 2011, 21, 494–504 CrossRef CAS.
- R. C. Edgar, UPARSE: highly accurate OTU sequences from microbial amplicon reads, Nat. Methods, 2013, 10, 996–998 CrossRef CAS PubMed.
- R. C. Edgar, MUSCLE: multiple sequence alignment with high accuracy and high throughput, Nucleic Acids Res., 2004, 32, 1792–1797 CrossRef CAS.
- S. K. Kim, T. Takeuchi, M. Yokoyama and Y. Murata, Effect of dietary supplementation with taurine, beta-alanine and GABA on the growth of juvenile and fingerling Japanese flounder Paralichthys olivaceus, Fish. Sci., 2003, 69, 242–248 CrossRef CAS.
- J. K. Chettri, N. P. Sahu, A. K. Pal, A. K. Reddy, S. Kumar and V. Kumar, Comparative performance of Gamma Amino Butyric Acid (GABA) and 5-Hydroxytryptamine (5-HT) in the diet of larvae and post larvae of giant freshwater prawn, Macrobrachium rosenbergii: Effect of dose and route of administration on growth and survival, Aquaculture, 2007, 270, 240–248 CrossRef CAS.
- C. Li, B. Zhang, X. Wang, X. Pi, X. Wang, H. Zhou, K. Mai and G. He, Improved utilization of soybean meal through fermentation with commensal Shewanella sp. MR-7 in turbot (Scophthalmus maximus L.), Microb. Cell Fact., 2019, 18, 214–214 CrossRef CAS PubMed.
- T. C. Delgado, Glutamate and GABA in appetite regulation, Front. Endocrinol., 2013, 4, 103–103 Search PubMed.
- V. I. Lushchak, Contaminant-induced oxidative stress in fish: a mechanistic approach, Fish Physiol. Biochem., 2016, 42, 711–747 CrossRef CAS PubMed.
- S. Rahimnejad, K. Lu, L. Wang, K. Song, K. Mai, D. A. Davis and C. Zhang, Replacement of fish meal with Bacillus pumillus SE5 and Pseudozyma aphidis ZR1 fermented soybean meal in diets for Japanese seabass (Lateolabrax japonicus), Fish Shellfish Immunol., 2018, 84, 987–997 CrossRef PubMed.
- F. Kokou, E. Sarropoulou, E. Cotou, G. Rigos, M. Henry, M. Alexis and M. Kentouri, Effects of fish meal replacement by a soybean protein on growth, histology, selected immune and oxidative status markers of gilthead sea bream, Sparus aurata, J. World Aquac. Soc., 2015, 46, 115–128 CrossRef CAS.
- Z. X. Xie, S. F. Xia, Y. Qiao, Y. H. Shi and G. W. Le, Effect of GABA on oxidative stress in the skeletal muscles and plasma free amino acids in mice fed high-fat diet, J. Anim. Physiol. Anim., 2015, 99, 492–500 CrossRef CAS.
- S.-B. Jeong, Y. B. Kim, J.-W. Lee, D.-H. Kim, B.-H. Moon, H.-H. Chang, Y.-H. Choi and K.-W. Lee, Role of dietary gamma-aminobutyric acid in broiler chickens raised under high stocking density, Anim. Nutr., 2020, 6, 293–304 CrossRef PubMed.
- C. Bi, J. Yin, W. Yang, B. Shi and A. Shan, Effects of dietary gamma-aminobutyric acid supplementation on antioxidant status, blood hormones and meat quality in growing-finishing pigs undergoing transport stress, J. Anim. Physiol. Anim. Nutr., 2020, 104, 590–596 CrossRef CAS.
- R. M. Martinez-Alvarez, A. E. Morales and A. Sanz, Antioxidant defenses in fish: Biotic and abiotic factors, Rev. Fish Biol. Fish., 2005, 15, 75–88 CrossRef.
- J. Q. Wu, T. R. Kosten and X. Y. Zhang, Free radicals, antioxidant defense systems, and schizophrenia, Prog. Neuro-Psychopharmacol. Biol. Psychiatry, 2013, 46, 200–206 CrossRef CAS.
- Y. Deng, W. Wang, P. Yu, Z. Xi, L. Xu, X. Li and N. He, Comparison of taurine, GABA, Glu, and Asp as scavengers of malondialdehyde in vitro and in vivo, Nanoscale Res. Lett., 2013, 8, 190 CrossRef.
- Y. Deng, L. Xu, X. Zeng, Z. Li, B. Qin and N. He, New perspective of GABA as an inhibitor of formation of advanced lipoxidation end-products: it's interaction with malondiadehyde, J. Biomed. Nanotechnol., 2010, 6, 318–324 CrossRef CAS.
- H. Bernstein, H. Holubec, J. A. Warneke, H. Garewal, D. L. Earnest, C. M. Payne, D. J. Roe, H. Y. Cui, E. L. Jacobson and C. Bernstein, Patchy field defects of apoptosis resistance and dedifferentiation in flat mucosa of colon resections from colon cancer patients, Ann. Surg. Oncol., 2002, 9, 505–517 Search PubMed.
- K. Fujimoto, R. Iwakiri, B. Wu, T. Fujise, S. Tsunada and A. Ootani, Homeostasis in the small intestinal mucosa balanced between cell proliferation and apoptosis is regulated partly by the central nervous system, J. Gastroenterol., 2002, 37, 139–144 CrossRef CAS.
- N. Dong, X. Xu, C. Xue, C. Wang, X. Li, C. Bi and A. Shan, Ethyl pyruvate inhibits LPS induced IPEC-J2 inflammation and apoptosis through p38 and ERK1/2 pathways, Cell Cycle, 2019, 18, 2614–2628 CrossRef CAS PubMed.
- A. M. Bakke-McKellep, M. H. Penn, P. M. Salas, S. Refstie, S. Sperstad, T. Landsverk, E. Ringo and A. Krogdahl, Effects of dietary soyabean meal, inulin and oxytetracycline on intestinal microbiota and epithelial cell stress, apoptosis and proliferation in the teleost Atlantic salmon (Salmo salar L.), Br. J. Nutr., 2007, 97, 699–713 CrossRef CAS.
- G. Yu, Y. Liu, W. Ou, J. Dai, Q. Ai, W. Zhang, K. Mai and Y. Zhang, The protective role of daidzein in intestinal health of turbot (Scophthalmus maximus L.) fed soybean meal-based diets, Sci. Rep., 2021, 11, 3352 CrossRef CAS.
- C. Sahlmann, B. J. G. Sutherland, T. M. Kortner, B. F. Koop, A. Krogdahl and A. M. Bakke, Early response of gene expression in the distal intestine of Atlantic salmon (Salmo salar L.) during the development of soybean meal induced enteritis, Fish Shellfish Immunol., 2013, 34, 599–609 CrossRef CAS.
- N. E. Saleh, Assessment of sesame meal as a soybean meal replacement in European sea bass (Dicentrarchus labrax) diets based on aspects of growth, amino acid profiles, haematology, intestinal and hepatic integrity and macroelement contents, Fish Physiol. Biochem., 2020, 46, 861–879 CrossRef CAS.
- A. M. Watson, F. Casu, D. W. Bearden, J. Yost, M. R. Denson, T. G. Gaylord, P. Anderson, P. A. Sandifer, J. W. Leffler and F. T. Barrows, Investigation of graded levels of soybean meal diets for red drum, Sciaenops ocellatus, using quantitative PCR derived biomarkers, Comp. Biochem. Physiol., Part D: Genomics Proteomics, 2019, 29, 274–285 CAS.
- N. Khan, O. Khymenets, M. Urpi-Sarda, S. Tulipani, M. Garcia-Aloy, M. Monagas, X. Mora-Cubillos, R. Llorach and C. Andres-Lacueva, Cocoa polyphenols and inflammatory markers of cardiovascular disease, Nutrients, 2014, 6, 844–880 CrossRef PubMed.
- C. Seimi, C. A. Cocchi, M. Lanfredini, C. L. Keen and M. E. Gershwin, Chocolate at heart: The anti-inflammatory impact of cocoa flavanols, Mol. Nutr. Food Res., 2008, 52, 1340–1348 CrossRef.
- T. Liu, L. Zhang, D. Joo and S.-C. Sun, NF-kappa B signaling in inflammation, Signal Transduction Targeted Ther., 2017, 2, 17023 CrossRef.
- G. Prud'homme, Y. Glinka and Q. Wang, GABA exerts anti-inflammatory
and immunosuppressive effects, J. Immunol., 2013, 190, 68–15 Search PubMed.
- K. D. Clements, E. R. Angert, W. L. Montgomery and J. H. Choat, Intestinal microbiota in fishes: what's known and what's not, Mol. Ecol., 2014, 23, 1891–1898 CrossRef PubMed.
- E. Ringo, Z. Zhou, J. L. G. Vecino, S. Wadsworth, J. Romero, A. Krogdahl, R. E. Olsen, A. Dimitroglou, A. Foey, S. Davies, M. Owen, H. L. Lauzon, L. L. Martinsen, P. de Schryver, P. Bossier, S. Sperstad and D. L. Merrifield, Effect of dietary components on the gut microbiota of aquatic animals. A never-ending story?, Aquac. Nutr., 2016, 22, 219–282 CrossRef CAS.
- E. Apper, D. Weissman, F. Respondek, A. Guyonvarch, F. Baron, P. Boisot, A. Rodiles and D. L. Merrifield, Hydrolysed wheat gluten as part of a diet based on animal and plant proteins supports good growth performance of Asian seabass (Lates calcarifer), without impairing intestinal morphology or microbiota, Aquaculture, 2016, 453, 40–48 CrossRef CAS.
- Y. Liu, Z. Chen, J. Dai, P. Yang, H. Hu, Q. Ai, W. Zhang, Y. Zhang, Y. Zhang and K. Mai, The protective role of glutamine on enteropathy induced by high dose of soybean meal in turbot, Scophthalmus maximus L, Aquaculture, 2018, 497, 510–519 CrossRef CAS.
- P. Lapebie, V. Lombard, E. Drula, N. Terrapon and B. Henrissat, Bacteroidetes use thousands of enzyme combinations to break down glycans, Nat. Commun., 2019, 10, 2043 CrossRef.
- K. Deng, M. Pan, J. Liu, M. Yang, Z. Gu, Y. Zhang, G. Liu, D. Liu, W. Zhang and K. Mai, Chronic stress of high dietary carbohydrate level causes inflammation and influences glucose transport through SOCS3 in Japanese flounder Paralichthys olivaceus, Sci. Rep., 2018, 8, 7415 CrossRef.
- S. Polakof, S. Panserat, J. L. Soengas and T. W. Moon, Glucose metabolism in fish: a review, J. Comp. Physiol., B, 2012, 182, 1015–1045 CrossRef CAS PubMed.
- G. Rizzatti, L. R. Lopetuso, G. Gibiino, C. Binda and A. Gasbarrini, Proteobacteria: a common factor in human diseases, BioMed Res. Int., 2017, 2017, 9351507 CAS.
- S. Egerton, S. Culloty, J. Whooley, C. Stanton and R. P. Ross, The gut microbiota of marine fish, Front. Microbiol., 2018, 9, 873 CrossRef.
- M. Ghanbari, W. Kneifel and K. J. Domig, A new view of the fish gut microbiome: Advances from next-generation sequencing, Aquaculture, 2015, 448, 464–475 CrossRef CAS.
- S. Fijan, Microorganisms with claimed probiotic properties: an overview of recent literature, Int. J. Environ. Res. Public Health, 2014, 11, 4745–4767 CrossRef.
- Z. Benmechernene, H. F. Chentouf, B. Yahia, G. Fatima, M. Quintela-Baluja, P. Calo-Mata and J. Barros-Velazquez, Technological aptitude and applications of Leuconostoc mesenteroides bioactive strains isolated from Algerian raw camel milk, BioMed Res. Int., 2013, 2013, 418132 Search PubMed.
- M. J. Foysal, M. Alam, A. Q. M. R. Kawser, F. Hasan, M. M. Rahman, C.-Y. Tay, M. S. H. Prodhan and S. K. Gupta, Meta-omits technologies reveals beneficiary effects of Lactobacillus plantarum as dietary supplements on gut microbiota, immune response and disease resistance of Nile tilapia (Oreochromis niloticus), Aquaculture, 2020, 520, 734974 CrossRef CAS.
- H. Tran Thi Thanh, H. Tran Thi Tuyet, L. Pham Thanh, S. Onoda and D. Pham Minh, Effects of dietary supplementation of heat-killed Lactobacillus plantarum L-137 on growth performance and immune response of bighead catfish (Clarias macrocephalus), Aquac. Rep., 2021, 20, 100741 CrossRef.
- G. Sagada, N. Gray, L. Wang, B. Xu, L. Zheng, Z. Zhong, S. Ullah, A. F. Tegomo and Q. Shao, Effect of dietary inactivated Lactobacillus plantarum on growth performance, antioxidative capacity, and intestinal integrity of black sea bream (Acanthopagrus schlegelii) fingerlings, Aquaculture, 2021, 535, 736370 CrossRef CAS.
- C.-Z. Zhu, D. Li, W.-J. Chen, S.-N. Ban, T. Liu, H. Wen and M. Jiang, Effects of dietary host-associated Lactococcus lactis on growth performance, disease resistance, intestinal morphology and intestinal microbiota of mandarin fish (Siniperca chuatsi), Aquaculture, 2021, 540, 736702 CrossRef CAS.
Footnote |
† Electronic supplementary information (ESI) available. See DOI: 10.1039/d1fo03034e |
|
This journal is © The Royal Society of Chemistry 2022 |
Click here to see how this site uses Cookies. View our privacy policy here.