DOI:
10.1039/D1FO02932K
(Paper)
Food Funct., 2022,
13, 242-254
Piperine, as a TAS2R14 agonist, stimulates the secretion of glucagon-like peptide-1 in the human enteroendocrine cell line Caco-2
Received
3rd September 2021
, Accepted 23rd November 2021
First published on 24th November 2021
Abstract
Piperine is reported to ameliorate common metabolic diseases, however, its molecular mechanism is still unclear. In the present study, we examined whether piperine could stimulate glucagon-like peptide-1 (GLP-1) secretion in a human enteroendocrine cell line, Caco-2, and explored the potential mechanisms from the activation of human bitter taste receptors (TAS2Rs). It was found that TAS2R14 was highly expressed in Caco-2 cells, far more than TAS2R4 and TAS2R10. Piperine and flufenamic acid (FA, a known TAS2R14 agonist) markedly increased intracellular calcium mobilization and significantly enhanced the GLP-1 secretion, accompanied by elevated levels of proglucagon mRNA in Caco-2 cells compared with the control. Moreover, piperine and FA activated TAS2R14 signaling as evidenced by the increased mRNA and protein levels of TAS2R14, and the protein expression of its downstream key molecules including phospholipase C β2 (PLCβ2) and a transient receptor potential channel melastatin 5 (TRPM5). On the other hand, a G protein βγ subunit inhibitor Gallein or a PLC inhibitor U73122 alleviated piperine-stimulated GLP-1 secretion in Caco-2 cells. In the meantime, a flavanone hesperetin significantly attenuated piperine and FA induced the intracellular calcium mobilization and GLP-1 secretion. Furthermore, TAS2R14 knockdown reversed the piperine-triggered up-regulation of PLCβ2 and TRPM5 as well as increased the GLP-1 secretion in Caco-2 cells by TAS2R14 shRNA transfection. In summary, our findings demonstrated that piperine promoted the GLP-1 secretion from enteroendocrine cells through the activation of TAS2R14 signaling. Moreover, TAS2R14 was likely a target of piperine in the alleviation of metabolic diseases.
Introduction
Gut hormones produced by enteroendocrine cells in the gastrointestinal tract regulate metabolism through the coordination of food ingestion, nutrient absorption, insulin secretion, and appetite control.1 Incretins, a class of gut hormones, can augment insulin secretion in a glucose-dependent manner, and glucagon-like peptide-1 (GLP-1) and glucose-dependent insulinotropic polypeptide are the two most important ones.2 In addition to insulinotropic secretion, GLP-1 exerts other significant activities, including the stimulation of insulin biosynthesis, inhibition of glucagon secretion, inhibition of gastric emptying and acid secretion, reduction of food intake, and trophic effects on the pancreas.3 Thus, by virtue of the pleiotropic activities of GLP-1, GLP-1 and its analogues are greatly beneficial for the treatment of metabolic diseases, such as diabetes and obesity.
GLP-1 is mostly released from the enteroendocrine L-cells predominantly in the distal small and large intestine.4 GLP-1 is produced through a tissue-specific post-translational modification of its precursor proglucagon (GCG as the gene symbol) by prohormone convertase 3. GLP-1 secretion induced by glucose is modulated by many factors including cellular energy metabolism, blockade of the ATP-sensitive K+ channel, electrogenic coupled Na+ and glucose entry via sodium-coupled glucose co-transporters, etc. Recently, bitter taste receptors (TAS2Rs) have been found to play important and complex roles in the digestive system and body metabolism. Interestingly, increasing evidence verifies that taste receptor pathways exert key roles in GLP-1 secretion, including both TAS2Rs and sweet taste receptors (TAS1Rs),5–7 which belong to G protein-coupled receptors. TAS2Rs are unequivocally expressed in all vital human organs, particularly in the nasal/oral respiratory tract, gastrointestinal tract, brain, and urogenital system,8 and it is intestinal TAS2R but not TAS1R signaling that predominates in the regulation of enteroendocrine hormone secretion including GLP-1 secretion.9,10
The majority of studies have shown that TAS2R-expressing cells use the canonical pathway to signal in response to stimulation.11 Heterotrimeric G proteins, composed of α-, β-, and γ-subunits, transduce external signals from heptahelical receptors to intracellular effectors. Binding of ligands to TAS2Rs initiates a signaling cascade, leading to a dissociation of the G-protein gustducin into Gα and Gβγ subunits. An increase in the intracellular Gβγ subunit activates phospholipase C β2 (PLCβ2), and then promotes the production of diacylglycerol and inositol 1,4,5-trisphophate, two important mediators of calcium release.6 On the other hand, the released Gα subunit stimulates phosphodiesterase to degrade cyclic adenosine monophosphate.12 In L-cells, the downstream signaling of taste receptor results in the stimulation of peptide hormones (such as GLP-1) and the regulation of ion channel (such as the transient receptor potential channel melastatin 5 (TRPM5)) opening.13 TAS2Rs not only sense toxins, but also recognize molecules, and each TAS2R responds to more than one bitter ligand.14 Importantly, GLP-1 secretion in L-cells can be provoked by a bitter taste due to the presence of secondary plant metabolites, bacterial toxins, or environmental contaminants.15 Considering the long history of herbal medicine and that many plants are bitter to humans, plenty of bitter compounds with different kinds from natural products have been reported to induce GLP-1 secretion through TAS2R signaling in enteroendocrine cells.16–18 Thus, the identification of more bitter agonists with the corresponding TAS2Rs may afford candidate compounds for modern medicine.
Piperine (1-piperoylpiperidine, C17H19NO3), the most abundant alkaloid isolated from Piper nigrum fruits, has a wide range of pharmacological activities.19 Piperine is verified to decrease blood glucose levels and promote glucose uptake.20,21 Moreover, piperine is found to be able to improve common metabolic diseases, such as insulin resistance and obesity-induced physiological derangements.22,23 However, it is still unclear whether the ameliorative effects of piperine on metabolism are associated with the stimulation of GLP-1 secretion in the gut. Considering that the activation of intestinal TAS2R signaling plays a vital role in GLP-1 secretion, we wonder whether piperine can activate TAS2R signaling pathways and then induce GLP-1 secretion in intestine. Experimental screening of novel bitter compounds is often expensive and laborious. Nonetheless, BitterX (an online tool) provides a platform for identifying potential human TAS2Rs for bitter small molecule compounds,24 and we find that piperine is bitter and can bind to TAS2R4, 14, and 10 subtypes with a higher probability. Thus, the effects of piperine on GLP-1 secretion and the activation of TAS2R signaling were observed in higher glucose cultured Caco-2 cells, not only a commonly used human enteroendocrine cell line of colon,25–27 but also expressing some subtypes of TAS2Rs.28,29
Materials and methods
Chemicals and materials
The Caco-2 cell line was purchased from FuHeng Biology, Shanghai, China; piperine (Pip, purity >98%) and hesperetin (Hst, purity >98%) were from Push Bio-Technology, Chengdu, China; flufenamic acid (FA, purity >99%) and 6-methoxyflavone (6-MF, purity >98%) were from Yuanye Biology, Shanghai, China; Dulbecco's modified Eagle's medium (DMEM) (4.5 g L−1D-glucose, Cat. #11995) was from Beijing Solarbio Science & Technology Co., Ltd, China; fetal bovine serum (FBS) was from GIBCO (Cat. #0270-106, Grand Island, NY); cell counting kit-8 (CCK-8) (Cat. #MA0218-5) was from Meilunbio, Dalian, China; the Fura-2 AM calcium probe (Cat. #S1052) was from Beyotime Biotechnology, Nantong, China; the human GLP-1 ELISA kit (Cat. #BP-E10998) was from Boyun Biology, Shanghai, China; the cDNA synthesis kit (Cat. #RR037A) and SYBR Premix Ex Taq II were from Takara, Dalian, China; the rabbit anti-TAS2R14 antibody (Cat. #DF5159) was from Affinity Biosciences Co., Ltd, USA; the rabbit anti-PLCβ2 antibody (Cat. #A8141) was from ABclonal Biotechnology Co., Ltd, Boston, USA; the rabbit anti-TRPM5 antibody (Cat. #18027-1-AP) was from Proteintech Group, Inc., USA; the rabbit anti-β-actin antibody (Cat. #AP0060) was from Bioworld Technology, St Louis, USA; the goat anti rabbit IgG (H + L) secondary antibody (Cat. #V926-32211) was from Li-Cor, Inc, Lincoln, NE; and three TAS2R14 shRNAs (Cat. # PSC100173, #PSC100174, #PSC100175) and the negative control (Cat. #GV112-NC) were from Shanghai GeneChem Chemical Technology, Co., Ltd, China.
Cell culture and treatments
Human Caco-2 enteroendocrine cells were maintained in DMEM with 10% or 15% FBS, 100 U ml−1 penicillin G, and 100 μg ml−1 streptomycin. The cells were cultured at 37 °C under 5% CO2. After cell cycle synchronization for 12 h, the Caco-2 cells were divided into the following groups: control (Ctrl, 25 mM glucose), different concentrations (2, 10, 50 μM) of piperine (Pip-2, 10, 50), and the positive control flufenamic acid (FA, 50 μM), a typical TAS2R14 agonist. After culturing for 24 h, the cells were harvested for the determination of mRNA and protein levels.
TAS2R14 knockdown
For cell transfection, Caco-2 cells were plated at a density of 1.0 × 105 cells per well onto a 6-well plate. When the cells reached 50% confluence, they were divided into three groups: blank control group (Ctrl), shRNA negative control group (NC), and shRNA-1, shRNA-2, and shRNA-3 groups. Transfection was performed in DMEM with 1% FBS for 12 h and continued up to 72 h with full culture to observe the fluorescence intensity of green fluorescence protein. After 48–72 h of killing of normal cells with puromycin, the transfection was complete and the cells were collected. Then the gene silencing efficiency was detected by quantitative real-time PCR. The most effective shRNA sequence was selected for further study. Briefly, lentivirus carrying the selected shRNA was transfected into Caco-2 cells, establishing a stable cell line with TAS2R14 knockdown. Then, the knockdown efficiency of the selected shRNA was further confirmed by the protein expression of TAS2R14. Finally, the cells were divided into two kinds: shRNA and NC. After different treatments for 24 h, the cells were collected for the detection of the protein expressions of PLCβ2 and TRPM5 as well as GLP-1 levels.
Cell viability by the CCK-8 assay
The cell viability of Caco-2 cells was analyzed using the CCK-8 method. Briefly, the cell suspension (100 μl per well, 1.0 × 106 ml−1) was pre-incubated in a 96-well plate for 24–48 h at 37 °C under a humidified atmosphere of 5% CO2. After the cells were incubated in different groups for 24 h, 10 μl of the CCK-8 solution was added to each well of the plate and incubated for 2 h in an incubator. After the addition of 10 μl of 1% (w/v) SDS to each well in the dark at room temperature, the absorbance was determined at 450 nm using a microplate reader. The net absorbance of the normal glucose group was considered as 100% of the cell proliferation viability.
Intracellular calcium level assay
Caco-2 cells were seeded at a clear bottom 96-well black plate, and the medium was changed with PBS after the cells were cultured to a logarithmic growth stage. The fluorescent probe was incubated to be loaded with 200 μl of fura-2 AM dye (5 μM) for 45 min at 37 °C in a dark place. After washing with PBS, drug treatments were performed for 30 min. Intracellular calcium mobilization was observed using a multipurpose microplate reader (Thermo Scientific) at an excitation wavelength of 340/380 nm and an emission wavelength of 510 nm. Intracellular free calcium concentration ([Ca2+]i) was expressed as a ratio of the relative intensity of fluorescence (λ340 nm/λ380 nm).
Measurement of GLP-1 in culture medium
After the Caco-2 cells were cultured with different treatments for 1 h, the culture medium was collected for the GLP-1 assay. GLP-1 levels were measured using the corresponding human GLP-1(7-36) ELISA kits according to the manufacturer's instructions.
Real-time qPCR assay
Total RNA isolation was performed using a TRIzol reagent, and the mRNA was reverse-transcribed into a single-stranded cDNA. The Roche 480 LightCycler® system with an SYBR Green dye binding to a PCR product was used to quantify target mRNA accumulation via fluorescence PCR using human β-actin as a reference. Human primers of the associated genes used for quantitative PCR in this study are presented in Table 1. Relative transcript abundance of a gene was expressed as 2−ΔΔCp values (ΔCp = Cptarget − Cpreference).
Table 1 Primer sequences of the associated genes used for quantitative PCR
Gene |
Forward (5′ to 3′) |
Reverse (5′ to 3′) |
TAS2R14 |
TGGGTGGTGTCATAAAGAGCAT |
GTGAGGATCCGATCAACCGA |
GCG |
TTCACAGGGCACATTCACCA |
TGGCAATGTTATTCCTGTTCCT |
β-actin |
TGACGTGGACATCCGCAAAG |
CTGGAAGGTGGACAGCGAGG |
Western blot assay
The cells were lysed in RIPA buffer with a 1 mM PMSF and 1 mM phosphatase inhibitor cocktail at 4 °C for 30 min followed by 12
000g centrifugation at 4 °C for 15 min to obtain the supernatant. The BCA protein assay was performed to determine the protein concentration according to the manufacturer's instructions. The protein samples were separated using sodium dodecyl sulfate polyacrylamide gel electrophoresis and transferred to a polyvinylidene fluoride membrane. The membrane was blocked with 2% milk powder solution for 60 min and incubated overnight at 4 °C with primary antibodies including anti-TAS2R14 (1
:
1000) and anti-TRPM5 (1
:
1000). The proteins were detected using an IRDye 800CW goat anti rabbit IgG (H + L) secondary antibody (1
:
1000). An infrared imaging system was used to detect immunoreactive blots. Signal densities on the blots were measured using the ImageJ software and normalized using an anti-β-actin antibody (1
:
1000) as an internal control.
Immunocytochemistry analysis
Caco-2 cells plated on round glass dishes were fixed with 4% paraformaldehyde for 15 min, permeabilized with 0.1% TritonX-100 in PBS for 5 min, and treated with blocking medium (1% bovine serum albumin in PBS) for 30 min. The cells were subsequently incubated with an anti-PLCβ2 antibody (1
:
200) at 4 °C overnight. An immune-reacted primary antibody was detected after 1 h of incubation in a dark place at 37 °C with the secondary antibody Dylight 594 Affinipure donkey anti-rabbit IgG (H + L) (1
:
250). The cells were further stained with DAPI for 2 min in a dark place at room temperature, washed, and then mounted onto microscope slides in mounting medium. The cells were viewed under an Olympus BX43F fluorescence microscope.
Statistical analysis
All statistical analyses were performed using the GraphPad Prism 7.0 software. Statistical significances among more than two groups were analyzed by one-way ANOVA followed by Dunnet's or Tukey's post hoc test. Data were presented as mean ± SD. P < 0.05 was considered statistically significant.
Results
Prediction of TAS2Rs for piperine in BitterX and TAS2R abundance in Caco-2 cells
Using the BitterX information platform, we found that piperine could bind to seven TAS2Rs, and the three subtypes TAS2R4, TAS2R14, and TAS2R10 had a higher probability. The details of the prediction result are presented in Table 2. In the meantime, we found that five TAS2R subtypes for piperine were overlapped with those of FA (Table 2). Therefore, we determined the abundance of TAS2R4, TAS2R14, and TAS2R10 in Caco-2 cells. It was found that these three TAS2Rs were all expressed in Caco-2 cells, and the abundance of TAS2R14 was the highest among them, with 9.46 and 28.84 fold that of TAS2R4 and TAS2R10, respectively (Fig. 1). Thus, we selected TAS2R14 as a potential target for piperine on GLP-1 secretion in Caco-2 cells using FA as a positive control drug.
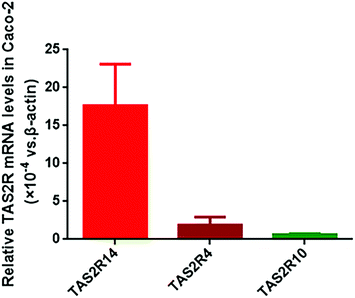 |
| Fig. 1 Abundance of TAS2Rs in Caco-2 cells. Mean ± SD, n = 5 independent experiments. | |
Table 2 Bitter compounds used in this study and receptor probability using BitterX
Compounds |
Receptor probabilitya (%) |
hTAS2R4 |
hTAS2R14
|
hTAS2R10 |
hTAS2R41 |
hTAS2R1 |
hTAS2R39 |
hTAS2R43 |
The activated rate of bitterness receptors was above 60%.
|
Piperine |
79.62 |
77.90 |
75.83 |
71.14 |
67.15 |
65.36 |
60.24 |
Flufenamic acid |
89.09 |
75.32 |
84.92 |
|
68.26 |
|
77.00 |
Hesperetin |
|
75.39 |
|
62.87 |
62.91 |
76.27 |
60.26 |
Concentration screening of piperine and FA
The effects of piperine on cell viability were observed to primarily screen the concentration of piperine in Caco-2 cells using a CCK-8 experiment. It was found that piperine at 0.1, 1, 10, and 50 μM did not affect the cell viability, but 100 μM piperine significantly decreased the cell viability (p < 0.01, Fig. 2A). Furthermore, three concentrations of piperine (2, 10, and 50 μM) were designed to examine the effects of piperine on intracellular calcium mobilization, finding that 10 and 50 μM piperine significantly increased [Ca2+]i in Caco-2 cells (both p < 0.01, Fig. 2B). Moreover, a series of FA (2, 5, 10, 20, 50 μM), a well-known TAS2R14 agonist, were used to investigate the effects of FA on intracellular calcium mobilization, finding that 20 and 50 μM FA overtly increased [Ca2+]i in Caco-2 cells (p < 0.05 or p < 0.01, Fig. 2C), with 50 μM FA having a higher percentage of increase (Fig. 2C). Together, piperine at 2, 10, and 50 μM and the TAS2R14 agonist FA at 50 μM as the positive control were selected for the subsequent studies.
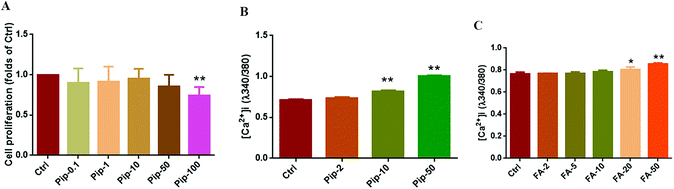 |
| Fig. 2 Effects of piperine on cell viability (A) and intracellular calcium mobilization (B) as well as the concentration screening of the positive control flufenamic acid (FA) (C) in Caco-2 cells. Ctrl, Pip-0.1, Pip-1, Pip-2, Pip-10, Pip-50, and Pip-100: cells treated with 25 mM glucose, plus 0.1, 1, 2, 10, 50, and 100 μM piperine, respectively. Ctrl, FA-2, FA-5, FA-10, FA-20, and FA-50: cells treated with 25 mM glucose, plus 2, 5, 10, 20, and 50 μM flufenamic acid (a typical TAS2R14 agonist), respectively. Mean ± SD, n = 3–6 same microplate wells. * p < 0.05, ** p < 0.01, vs. Ctrl. | |
Piperine upregulated the gene expression of GLP-1 and TAS2R14
We investigated the gene expression of GLP-1 in Caco-2 cells, verifying the existence of the transcript of proglucagon (GCG as the gene symbol), the precursor of GLP-1, in Caco-2 cells. We found that all the three concentrations of piperine increased the GCG mRNA levels and the high concentration had the best effect compared with the Ctrl (p < 0.01, Fig. 3A). Furthermore, the effects of piperine on the gene expression of TAS2R14 were also examined, and all the three concentrations of piperine increased the TAS2R14 mRNA levels in Caco-2 cells, with the high concentration showing a statistically significant difference in comparison with the Ctrl (p < 0.05, Fig. 3B). The positive control FA also significantly increased the GCG and TAS2R14 mRNA levels (both p < 0.01, Fig. 3A and B). These results demonstrated that piperine can promote proglucagon and TAS2R14 gene expressions in Caco-2 cells.
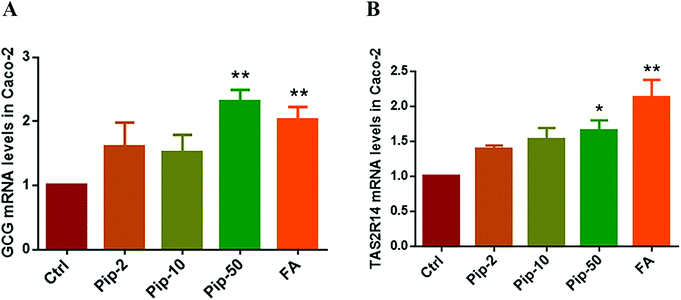 |
| Fig. 3 Effects of piperine on the mRNA levels of proglucagon (GCG as the gene symbol) (A) and TAS2R14 (B) in Caco-2 cells. Ctrl, Pip-2, Pip-10, Pip-50, and FA: cells treated with 25 mM glucose, plus 2, 10, and 50 μM piperine, and 50 μM flufenamic acid (a typical TAS2R14 agonist), respectively. Mean ± SD, n = 5 independent experiments. * p < 0.05, ** p < 0.01 vs. Ctrl. | |
Piperine promoted GLP-1 secretion and inhibition of the Gβγ/PLCβ2 pathway attenuated the effects
On the basis of distinctly increased proglucagon expression by piperine, we found that all the three concentrations of piperine promoted GLP-1 secretion in Caco-2 cells, and piperine at 10 and 50 μM showed a remarkable increase in GLP-1 levels in culture medium (both p < 0.01, Fig. 4A). FA also markedly (p < 0.05) increased GLP-1 levels in medium, but the effect was much weaker than that of 10 μM piperine (Fig. 4A). These demonstrated that piperine showed strong promotion of GLP-1 secretion.
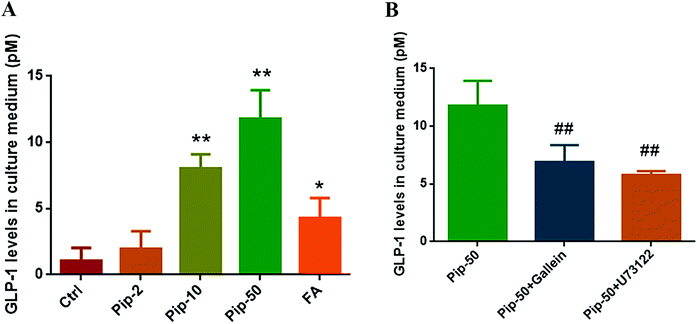 |
| Fig. 4 Effects of piperine on GLP-1 secretion (A) and the inhibitory effects by Gβγ inhibition or PLC inhibition (B) in Caco-2 cells. Ctrl, Pip-2, Pip-10, Pip-50, and FA: cells treated with 25 mM glucose, plus 2, 10, and 50 μM piperine, and 50 μM flufenamic acid (a typical TAS2R14 agonist), respectively. Gallein, a Gβγ inhibitor; U73122, a PLC inhibitor. Mean ± SD, n = 5 independent experiments. * p < 0.05, ** p < 0.01, vs. Ctrl; ## p < 0.01, vs. Pip-50. | |
To examine whether GLP-1 secretion in response to piperine was involved in TAS2R/Gβγ signaling, we evaluated the GLP-1 level after piperine co-treatment with a Gβγ inhibitor Gallein or a PLC inhibitor U73122. GLP-1 secretion in response to piperine was markedly attenuated by Gallein or U73122 in Caco-2 cells (both p < 0.01, Fig. 4B). This indicated that the TAS2R14/Gβγ/PLCβ2 pathway might mediate the effect of piperine on GLP-1 secretion from enteroendocrine cells.
Piperine activated TAS2R14/Gβγ/PLCβ2 pathway
We further investigated the activation of the TAS2R14/Gβγ/PLCβ2 pathway by piperine. Piperine increased the protein expressions of TAS2R14 and its distal downstream molecule TRPM5 in Caco-2 cells compared with the control, with the middle and high concentrations of piperine (10 and 50 μM) showing statistically significant differences (p < 0.05 or p < 0.01, Fig. 5A and B). In the meantime, the middle and high concentrations of piperine greatly increased the protein expression of PLCβ2 in Caco-2 cells (Fig. 5C). Moreover, the high concentration of piperine had similar effects to a typical TAS2R14 agonist FA on the above indices (Fig. 5A–C). These results demonstrated that piperine indeed activated the TAS2R14/Gβγ/PLCβ2 pathway in Caco-2 cells.
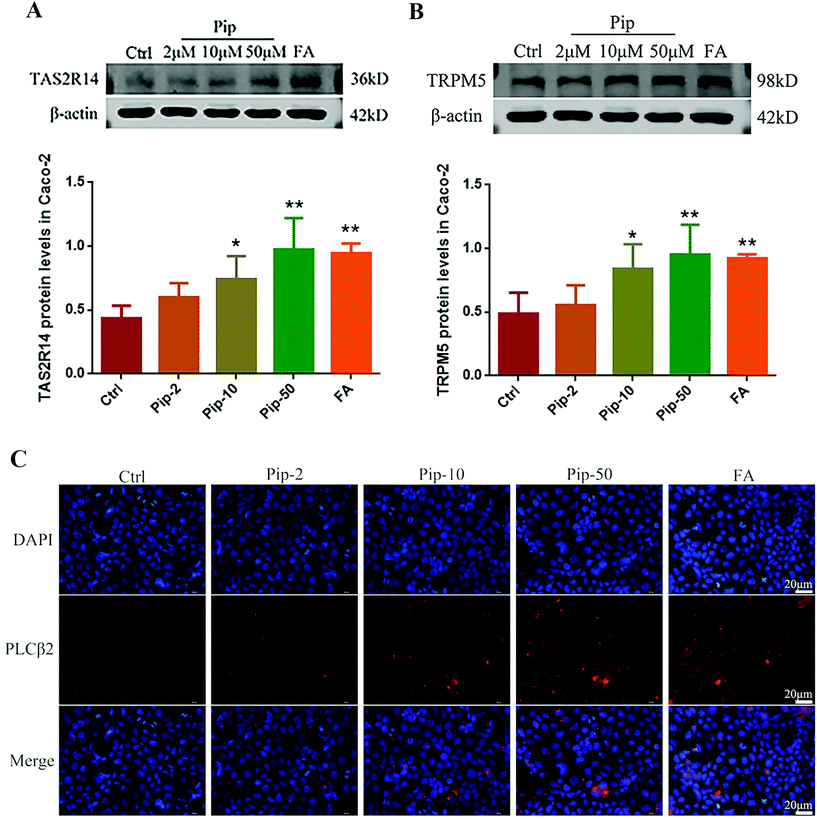 |
| Fig. 5 Effects of piperine on the protein expression of TAS2R14 (A), TRPM5 (B), and PLCβ2 (C) in Caco-2 cells. Ctrl, Pip-2, Pip-10, Pip-50, and FA: cells treated with 25 mM glucose, and plus 2, 10, and 50 μM piperine, 50 μM flufenamic acid (a typical TAS2R14 agonist), respectively. Mean ± SD, n = 3 independent experiments. * p < 0.05, ** p < 0.01, vs. Ctrl. Scale bar 20 μm. | |
Hesperetin was verified to be an antagonist of TAS2R14 signaling
6-Methoxyflavone was used as a TAS2R14 antagonist.30 Therefore the effects of piperine were examined on intracellular calcium mobilization after the high concentration of piperine (50 μM) was co-treated with different concentrations of 6-MF. Much to our surprise, 6-MF at 25, 50, and 100 μM did not mute while increasing the effect of piperine on [Ca2+]i in Caco-2 cells (all p < 0.01, Fig. 6A). Nevertheless, certain flavanones were known to mask bitter taste sensorially, and some flavanones were proved to blunt the effects of the known activators of TAS2Rs (TAS2R39 and TAS2R14).31 Since hesperetin, 4′-methoxy-3′,5,7-trihydroxy flavanone, has overlapping TAS2R subtypes with piperine and FA, including TAS2R14 (Table 2), hesperetin was selected to test TAS2R14 antagonism using calcium mobilization. It was found that hesperetin at 10, 30, and 100 μM all significantly decreased the intracellular calcium concentration in Caco-2 cells (all p < 0.01, Fig. 6B), indicating that hesperetin might be a blocker of TAS2Rs. Furthermore, hesperetin at 25, 50, or 100 μM co-treatment with the high concentration of piperine reduced the [Ca2+]i level (all p < 0.01, Fig. 6C) and GLP-1 secretion (p < 0.05 or p < 0.01, Fig. 6D) compared with the high concentration of piperine alone in Caco-2 cells. Moreover, hesperetin co-treatment with FA also overturned FA-induced calcium mobilization (p < 0.01, Fig. 6C) and GLP-1 secretion (p < 0.05, Fig. 6D). These results demonstrated that the flavanone hesperetin could be used as an antagonist of TAS2R14.
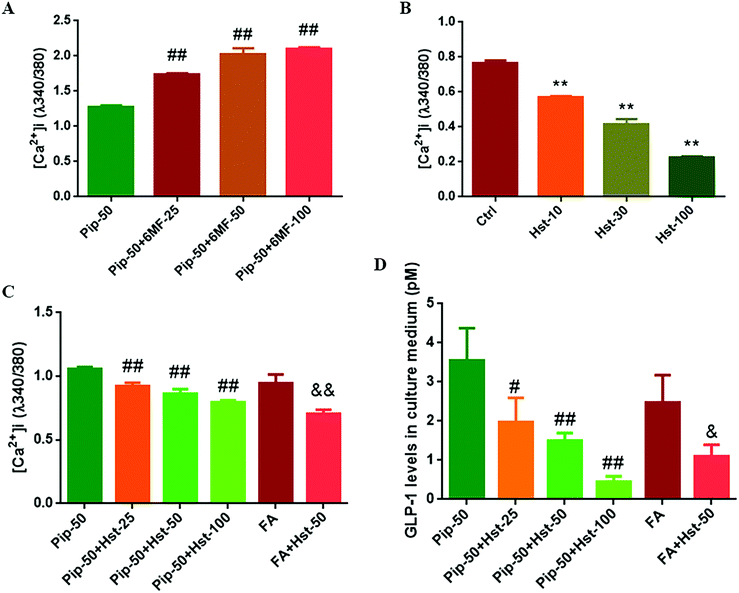 |
| Fig. 6 Effects of TAS2R14 blocking on piperine in calcium mobilization and GLP-1 secretion. (A) 6-Methoxyflavone (6-MF) did not attenuate while enhancing the effect of piperine; (B) a potential TAS2R14 blocker flavanone hesperetin (Hst) reduced the intracellular calcium levels; hesperetin attenuated the effects of piperine and flufenamic acid on calcium mobilization (C) and GLP-1 secretion (D). Ctrl, Pip-50, and FA indicate 25 mM glucose, 50 μM piperine, and 50 μM flufenamic acid (a typical TAS2R14 agonist), respectively. 6-MF-25, 6-MF-50, and 6-MF-100 indicate 25, 50, and 100 μM 6-methoxyflavone, respectively, and Hst-25, Hst-50, and Hst-100 indicate 25, 50, and 100 μM hesperetin, respectively. Mean ± SD, n = 3 same microplate wells (A and C), n = 3 independent experiments (B and D). One-way ANOVA with the Tukey post-test was used for the analysis of statistical significance (C and D). # p < 0.05, ## p < 0.01, vs. Pip-50; ** p < 0.01, vs. Ctrl; &p < 0.05, &&p < 0.01, vs. FA. | |
Hesperetin antagonized the effects of piperine on the TAS2R14/Gβγ/PLCβ2 pathway
Hesperetin as an antagonist of TAS2R14 signaling was further confirmed using two agonists of TAS2R14, namely, piperine and FA. Co-treatment with hesperetin at 50 μM markedly relieved the increase in PLCβ2 protein expression induced by the test compound piperine or the positive control FA (Fig. 7A). Moreover, co-treatment with Gallein, a Gβγ inhibitor, also overtly alleviated PLCβ2 expression induced by our target compound piperine (Fig. 7A). Furthermore, co-treatment with hesperetin at 50 μM abolished increases in the TRPM5 expression caused by piperine or FA (Fig. 7B). These indicated that hesperetin co-treatment attenuated the activation of the TAS2R14/Gβγ/PLCβ2 pathway triggered by piperine and FA.
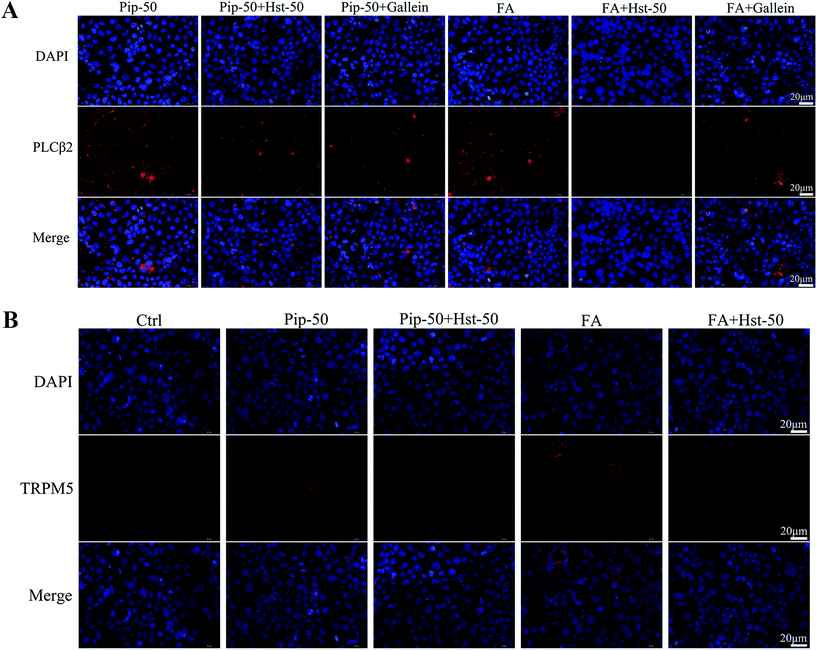 |
| Fig. 7 Antagonizing effects of TAS2R14 blocker hesperetin (Hst) on piperine and flufenamic acid (a typical TAS2R14 agonist) in PLCβ2 (A) and TRPM5 (B) in Caco-2 cells. Ctrl, Pip-50, and FA: cells treated with 25 mM glucose, plus 50 μM piperine or 50 μM flufenamic acid, respectively. Hst-50 and Gallein indicate 50 μM hesperetin and a Gβγ inhibitor at 10 μM, respectively. Scale bar 20 μm. | |
Evaluation and validation of TAS2R14 knockdown
Three TAS2R14 shRNA sequences were used to test the knockdown efficiency of TAS2R14 mRNA. The results of real-time quantitative PCR indicated that the mRNA level of TAS2R14 was higher in Caco-2 cells in the negative control and shRNA-1 groups than that in the blank control group, unchanged in the shRNA-2 group, but the lowest in the shRNA-3 group (Fig. 8A). The shRNA-3 treatment exhibited a marked knockdown effect on the TAS2R14 mRNA level, with an 89.3% reduction relative to the blank control group. Therefore, shRNA-3 was used to establish a stable transfection cell line of Caco-2 cells and continue the follow-up experiments. Furthermore, the protein expression of TAS2R14 was also examined using shRNA-3 treatment in Caco-2 cells. Compared with the negative control, the shRNA-3 treatment remarkably decreased the protein expression of TAS2R14 in Caco-2 cells, with a 47.5% reduction (Fig. 8B), further confirming the good effectiveness of shRNA-3.
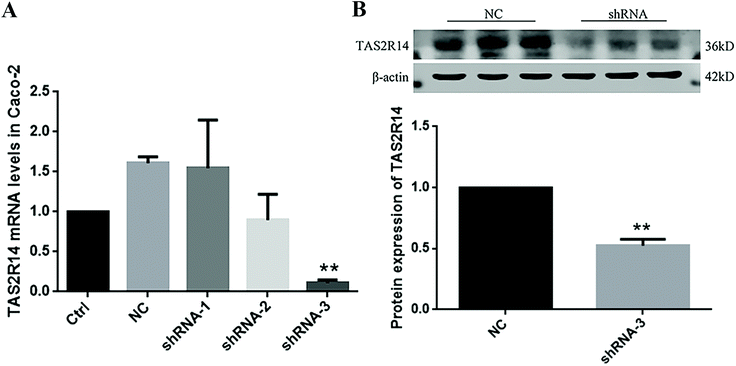 |
| Fig. 8 Effects of three candidate TAS2R14 shRNAs on the TAS2R14 mRNA level (A) and protein expression (B) in Caco-2 cells. Ctrl, NC, shRNA-1, shRNA-2, and shRNA-3 represent 25 mM glucose, the negative control of TAS2R14 shRNA, and candidate TAS2R14 shRNAs 1, 2, and 3, respectively. Mean ± SD, n = 5 independent experiments. ** p < 0.01, vs. NC. | |
TAS2R14 knockdown abolished effects of piperine on the activation of the TAS2R14/Gβγ/TRPM5 pathway and GLP-1 secretion
Although the negative control had an increased tendency towards the TAS2R14 mRNA level compared with the blank control (Fig. 8A), the negative control showed a similar effect to the blank control on the protein expression of PLCβ2, a downstream molecule of TAS2R14 signaling (Fig. 9A). PLCβ2 protein expression was markedly reduced after the TAS2R14 knockdown in Caco-2 cells compared with the negative control, and a piperine-induced PLCβ2 increase in protein expression was abrogated after the TAS2R14 knockdown using shRNA (Fig. 9A). Moreover, the TRPM5 protein expression was significantly decreased after the TAS2R14 knockdown in Caco-2 cells compared with the negative control, and a piperine-induced TRPM5 increase was abolished after the TAS2R14 knockdown (Fig. 9B). Importantly, GLP-1 secretion was also markedly diminished after the TAS2R14 knockdown in Caco-2 cells compared with the negative control, and piperine-induced GLP-1 secretion was reversed after the TAS2R14 knockdown (Fig. 9C). These results further verified that TAS2R14 was an active target of piperine on the activation of the TAS2R/Gβγ/TRPM5 pathway and subsequent GLP-1 secretion.
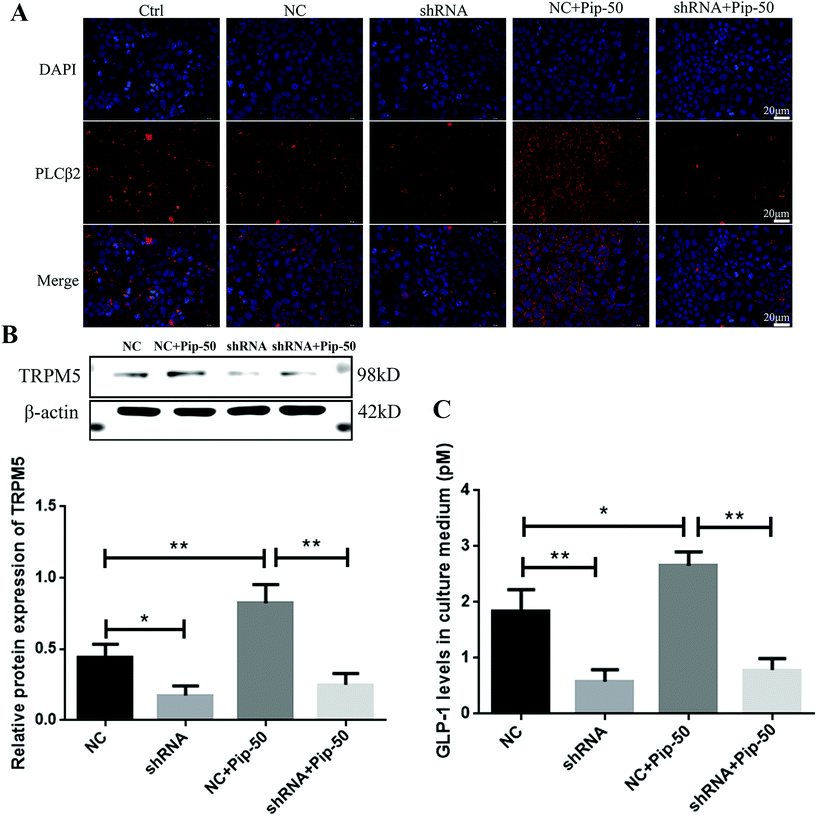 |
| Fig. 9 Effects of TAS2R14 knockdown on piperine in PLCβ2 (A), TRPM5 (B), and GLP-1 secretion (C) in Caco-2 cells. Ctrl, NC, shRNA, and Pip-50 represent 25 mM glucose, the negative control of TAS2R14 shRNA, TAS2R14 shRNA, and 50 μM piperine, respectively. Mean ± SD, n = 3 independent experiments. One-way ANOVA with the Tukey post-test was used for the analysis of statistical significance. * p < 0.05, ** p < 0.01. Scale bar 20 μm. | |
Discussion
There is growing interest in bitter tastants in the enteroendocrine secretion of gut hormones including GLP-1 through the activation of TAS2Rs. In the present study, we found that Caco-2 cells highly expressed TAS2R14 and GCG (the gene symbol of GLP-1 precursor proglucagon) mRNA. On the basis of these results, we found that piperine markedly induced GLP-1 secretion and increased intracellular calcium mobilization in Caco-2 cells. Further studies demonstrated that the activation of the TAS2R14/Gβγ/PLCβ2/TRPM5 pathway contributed to the piperine promoting GLP-1 secretion using both pharmacological inhibition and TAS2R14 knockdown. This study verified that piperine is a strong TAS2R14 agonist with high potency.
Caco-2 cells possess L-cell like functions to secrete GLP-1. Both Caco-2 cells and NCI-H716 cells belong to the human colorectal adenocarcinoma cell line. NCI-H716 cells are frequently used for the in vitro experiment of GLP-1 secretion,6,7 but this cell line is a kind of suspension cultured cell and needs treatment of cell differentiation. In the current study, we found that Caco-2 cells markedly expressed the gene transcripts of the GLP-1 precursor (GCG as the gene symbol), and secreted enough GLP-1 to be determined after higher glucose stimulation. Moreover, piperine significantly increased the GCG mRNA level and GLP-1 secretion in culture medium in Caco-2 cells, and the effects of piperine were stronger than those of flufenamic acid, a representative agonist of TAS2R14. Early in 2015, Song et al. have found that Caco-2 cells express the gene transcripts of gut hormones, cholecystokinin, prohormone convertase 1, proglucagon, peptide YY, cholecystokinin, GLP-1 and peptide YY are secreted from Caco-2 cells after adding sugars, amino acids or fatty acids.26 Recently, intermittent hypoxia has up-regulated the gene expressions of peptide YY and GLP-1 in Caco-2 cells.25 Therefore, these results demonstrate that Caco-2 cells can be used as a cell line for the in vitro study of GLP-1 secretion. Three cell lines human NCI-H716 cells and mouse GLUTag and STC-1 cells can produce and process proglucagon into GLP-1 and increase GLP-1 secretion after stimulations with KCl or glucose plus forskolin/3-isobutyl-1-methylxanthine.32 Thus, these results indicate that Caco-2 is a supplement for an enterodocrine cell line in GLP-1 secretion. Additionally, hormone production in cell line models of the L-cell has limited similarity to the natural L-cells,26 so these results of piperine need to be further examined in mice and STC-1 cells.
Caco-2 cells express high abundance of TAS2R14. Based on the detection range, TAS2Rs are of two types, namely, promiscuous and selective ones, and diverse bitter stimuli activate distinct receptors. We predicted potential TAS2Rs for piperine, indicating that piperine showed a high probability to bind with TAS2R4, TAS2R14, and TAS2R10. In our study, Caco-2 cells expressed the mRNA of all these three receptors, and the abundance of TAS2R14 was far more than those of TAS2R4 and TAS2R10. The ranks of TAS2R14, TAS2R4, and TAS2R10 in Caco-2 cells were the same as that in NCI-H716 cells.6 Additionally, low abundance of TAS2R10 in Caco-2 cells in our study was consistent with that in the recent report.29 In our study, piperine and flufenamic acid increased the mRNA levels of TAS2R14 in Caco-2 cells. Thus, Caco-2 cells can be used to investigate the biological functions of TAS2R14 in the gut, such as stimulating L-cell secretion.
Flavanones rather than flavones are potential antagonists for TAS2R14. Flavonoids can bind with some TAS2Rs, especially TAS2R14 and TAS2R39.8,33 Roland et al. reported that 6-methoxyflavanones are selective TAS2R39 antagonists, and 6-methoxyflavanones also antagonize TAS2R14 to a lesser extent;31 whereas 6-methoxyflavone is used as a TAS2R14 antagonist and abolishes competence stimulating peptides 1-primed gingival epithelial cells attracting differentiated HL-60 immune cells.30 In our study, however, 6-methoxyflavone did not antagonize but synergized the effects of piperine on intracellular calcium mobilization in Caco-2 cells. Fortunately, we found that a flavanone hesperetin dose-dependently reduced intracellular calcium levels in Caco-2 cells, and hesperetin co-treatment with piperine alleviated piperine-stimulated calcium mobilization and GLP-1 secretion, suggesting that hesperetin antagonized the effects of piperine. Some studies have reported that hesperetin increases intracellular calcium levels in HEK-293T cells overexpressed with TAS2R14.33,34 These inconsistent results can be explained by different cell models and baseline calcium levels. Hesperetin as an antagonist of TAS2R14 was further confirmed in our latter experiments. The common flavanones also include naringenin, eriodictyol, homoeriodictyol, etc. Together, a flavanone hesperetin can act as an antagonist rather than an agonist of TAS2R14 in the functional studies of TAS2R14.
Piperine increases GLP-1 secretion through the activation of TAS2R14 signaling. Bitter alkaloid compounds are reported to strengthen TAS2R signaling and subsequently induce GLP-1 secretion in enteroendocrine cells.7,16 In our study, like the TAS2R14 agonist flufenamic acid, piperine, a natural bitter alkaloid compound, activated the TAS2R14/Gβγ/PLCβ2 pathway in Caco-2 cells, reflected by the increased protein expression of TAS2R14 and PLCβ2. It has been found that the TAS2R agonist induced increase in the intracellular free calcium concentration is correlated with the expression level of the TAS2R subtype.35 In our study, piperine increased the intracellular calcium levels along with up-regulated TAS2R14 mRNA and protein expression in Caco-2 cells. TRPM5 is a key component of the downstream signaling pathway shared by sweet, bitter, and umami tastes,36 and Trpm5-knockout mice are not able to perceive sweet, amino acid, and bitter tastes.11 In the present study, both piperine and flufenamic acid increased the TRPM5 protein expression in Caco-2 cells, subsequently causing GLP-1 secretion. A recent report has verified that the TAS2R14 agonist flufenamic acid can increase the total GLP-1 secretion in the rat ex vivo ileum.37 On the other hand, a Gβγ inhibitor Gallein or a PLC inhibitor U73122 reversed a piperine-stimulated increase in GLP-1 secretion in Caco-2 cells. Moreover, a TAS2R14 antagonist hesperetin attenuated piperine and flufenamic acid induced activation of TAS2R14 signaling in Caco-2 cells, evidenced by the decreased protein expression of PLCβ2 and TRPM5, the key downstream molecules of TAS2R14/Gβγ signaling. Furthermore, TAS2R14 knockdown abrogated the enhanced effects of piperine on PLCβ2 and TRPM5 as well as GLP-1 secretion in Caco-2 cells. Together, these results clarify that TAS2R14 is an active target for piperine in promoting GLP-1 secretion.
In conclusion, our study demonstrates that piperine can enhance the intestinal L-cell like functions and markedly promote GLP-1 secretion in the enteroendocrine cell line Caco-2, and the human bitter taste receptor subtype 14 is an active target for piperine. Moreover, our findings partially elucidate the molecular mechanism of piperine, improving common metabolic diseases, including diabetes and obesity.
Author contributions
Yao-Wu Liu: conceptualization, project administration, funding acquisition, and writing – review and editing. Ting-Ting Huang: investigation, methodology, visualization, and writing – original draft preparation. Pan-Pan Gu: methodology and data curation. Ting Zheng: supervision and resources. Ling-Shan Gou: funding acquisition and writing – review and editing. All data were generated in-house, and no paper mill was used. All authors read and approved the final manuscript.
Conflicts of interest
The authors declare no competing interests.
Acknowledgements
This study was supported by the Qinglan Project of Jiangsu Province (2014) and the Medical Science Foundation of Jiangsu Province (H2019007), China.
References
- F. M. Gribble and F. Reimann, Function and mechanisms of enteroendocrine cells and gut hormones in metabolism, Nat. Rev. Endocrinol., 2019, 15, 226–237 CrossRef CAS PubMed.
- L. L. Baggio and D. J. Drucker, Biology of incretins: GLP-1 and GIP, Gastroenterology, 2007, 132, 2131–2157 CrossRef CAS PubMed.
- J. F. Gautier, S. P. Choukem and J. Girard, Physiology of incretins (GIP and GLP-1) and abnormalities in type 2 diabetes, Diabetes Metab., 2008, 34(Suppl 2), S65–S72 CrossRef CAS.
- F. M. Gribble and F. Reimann, Enteroendocrine Cells: Chemosensors in the Intestinal Epithelium, Annu. Rev. Physiol., 2016, 78, 277–299 CrossRef CAS PubMed.
- H. J. Jang, Z. Kokrashvili, M. J. Theodorakis, O. D. Carlson, B. J. Kim, J. Zhou, H. H. Kim, X. Xu, S. L. Chan, M. Juhaszova, M. Bernier, B. Mosinger, R. F. Margolskee and J. M. Egan, Gut-expressed gustducin and taste receptors regulate secretion of glucagon-like peptide-1, Proc. Natl. Acad. Sci. U. S. A., 2007, 104, 15069–15074 CrossRef CAS PubMed.
- K. S. Kim, J. M. Egan and H. J. Jang, Denatonium induces secretion of glucagon-like peptide-1 through activation of bitter taste receptor pathways, Diabetologia, 2014, 57, 2117–2125 CrossRef CAS PubMed.
- Y. Yu, G. Hao, Q. Zhang, W. Hua, M. Wang, W. Zhou, S. Zong, M. Huang and X. Wen, Berberine induces GLP-1 secretion through activation of bitter taste receptor pathways, Biochem. Pharmacol., 2015, 97, 173–177 CrossRef CAS PubMed.
- E. Tarragon and J. J. Moreno, Polyphenols and taste 2 receptors. Physiological, pathophysiological and pharmacological implications, Biochem. Pharmacol., 2020, 178, 114086 CrossRef CAS PubMed.
- C. Xie, X. Wang, R. L. Young, M. Horowitz, C. K. Rayner and T. Wu, Role of Intestinal Bitter Sensing in Enteroendocrine Hormone Secretion and Metabolic Control, Front. Endocrinol., 2018, 9, 576 CrossRef PubMed.
- M. Y. Saltiel, R. E. Kuhre, C. B. Christiansen, R. Eliasen, K. W. Conde-Frieboes, M. M. Rosenkilde and J. J. Holst, Sweet Taste Receptor Activation in the Gut Is of Limited Importance for Glucose-Stimulated GLP-1 and GIP Secretion, Nutrients, 2017, 9, 418 CrossRef PubMed.
- Y. Zhang, M. A. Hoon, J. Chandrashekar, K. L. Mueller, B. Cook, D. Wu, C. S. Zuker and N. J. Ryba, Coding of sweet, bitter, and umami tastes: different receptor cells sharing similar signaling pathways, Cell, 2003, 112, 293–301 CrossRef CAS PubMed.
- R. F. Margolskee, Molecular mechanisms of bitter and sweet taste transduction, J. Biol. Chem., 2002, 277, 1–4 CrossRef CAS PubMed.
- S. C. Kinnamon, Taste receptor signalling - from tongues to lungs, Acta Physiol., 2012, 204, 158–168 CrossRef CAS PubMed.
- W. Meyerhof, C. Batram, C. Kuhn, A. Brockhoff, E. Chudoba, B. Bufe, G. Appendino and M. Behrens, The molecular receptive ranges of human TAS2R bitter taste receptors, Chem. Senses, 2010, 35, 157–170 CrossRef CAS PubMed.
- F. Raka, S. Farr, J. Kelly, A. Stoianov and K. Adeli, Metabolic control via nutrient-sensing mechanisms: role of taste receptors and the gut-brain neuroendocrine axis, Am. J. Physiol. Endocrinol. Metab., 2019, 317, E559–E572 CrossRef CAS PubMed.
- X. Yue, J. Liang, F. Gu, D. Du and F. Chen, Berberine activates bitter taste responses of enteroendocrine STC-1 cells, Mol. Cell. Biochem., 2018, 447, 21–32 CrossRef CAS PubMed.
- M. H. Shin, E. K. Choi, K. S. Kim, K. H. Kim, Y. P. Jang, K. S. Ahn, W. S. Chung, N. H. Cha and H. J. Jang, Hexane Fractions of Bupleurum falcatum L. Stimulates
Glucagon-Like Peptide-1 Secretion through G beta gamma -Mediated Pathway, J. Evidence-Based Complementary Altern. Med., 2014, 2014, 982165 Search PubMed.
- J. Li, J. Xu, R. Hou, X. Jin, J. Wang, N. Yang, L. Yang, L. Liu, F. Tao and H. Lu, Qing-Hua Granule induces GLP-1 secretion via bitter taste receptor in db/db mice, Biomed. Pharmacother., 2017, 89, 10–17 CrossRef PubMed.
- R. M. Gutierrez, A. M. Gonzalez and C. Hoyo-Vadillo, Alkaloids from piper: a review of its phytochemistry and pharmacology, Mini-Rev. Med. Chem., 2013, 13, 163–193 CAS.
- S. Atal, R. P. Agrawal, S. Vyas, P. Phadnis and N. Rai, Evaluation of the effect of piperine per se on blood glucose level in alloxan-induced diabetic mice, Acta Pol. Pharm., 2012, 69, 965–969 CAS.
- A. Maeda, T. Shirao, D. Shirasaya, Y. Yoshioka, Y. Yamashita, M. Akagawa and H. Ashida, Piperine Promotes Glucose Uptake through ROS-Dependent Activation of the CAMKK/AMPK Signaling Pathway in Skeletal Muscle, Mol. Nutr. Food Res., 2018, 62, e1800086 CrossRef PubMed.
- S. Choi, Y. Choi, Y. Choi, S. Kim, J. Jang and T. Park, Piperine reverses high fat diet-induced hepatic steatosis and insulin resistance in mice, Food Chem., 2013, 141, 3627–3635 CrossRef CAS PubMed.
- P. BrahmaNaidu, H. Nemani, B. Meriga, S. K. Mehar, S. Potana and S. Ramgopalrao, Mitigating efficacy of piperine in the physiological derangements of high fat diet induced obesity in Sprague Dawley rats, Chem.-Biol. Interact., 2014, 221, 42–51 CrossRef CAS PubMed.
- W. Huang, Q. Shen, X. Su, M. Ji, X. Liu, Y. Chen, S. Lu, H. Zhuang and J. Zhang, BitterX: a tool for understanding bitter taste in humans, Sci. Rep., 2016, 6, 23450 CrossRef CAS PubMed.
- R. Shobatake, A. Itaya-Hironaka, A. Yamauchi, M. Makino, S. Sakuramoto-Tsuchida, T. Uchiyama, H. Ota, N. Takahashi, S. Ueno, K. Sugie and S. Takasawa, Intermittent Hypoxia Up-Regulates Gene Expressions of Peptide YY (PYY), Glucagon-like Peptide-1 (GLP-1), and Neurotensin (NTS) in Enteroendocrine Cells, Int. J. Mol. Sci., 2019, 20, 1849 CrossRef CAS PubMed.
- W. Y. Song, Y. Aihara, T. Hashimoto, K. Kanazawa and M. Mizuno, (-)-Epigallocatechin-3-gallate induces secretion of anorexigenic gut hormones, J. Clin. Biochem. Nutr., 2015, 57, 164–169 CrossRef CAS PubMed.
- P. K. Huang, S. R. Lin, C. H. Chang, M. J. Tsai, D. N. Lee and C. F. Weng, Natural phenolic compounds potentiate hypoglycemia via inhibition of Dipeptidyl peptidase IV, Sci. Rep., 2019, 9, 15585 CrossRef PubMed.
- T. I. Jeon, Y. K. Seo and T. F. Osborne, Gut bitter taste receptor signalling induces ABCB1 through a mechanism involving CCK, Biochem. J., 2011, 438, 33–37 CrossRef CAS PubMed.
- H. Zhu, L. Liu, L. Ren, J. Ma, S. Hu, Z. Zhu, X. Zhao, C. Shi, X. Wang, C. Zhang, M. Gu and X. Li, Systematic prediction of the biological functions of TAS2R10 using positive co-expression analysis, Exp. Ther. Med., 2020, 19, 1733–1738 CAS.
- M. R. Medapati, N. Singh, A. Y. Bhagirath, K. Duan, B. Triggs-Raine, E. L. Batista Jr. and P. Chelikani, Bitter taste receptor T2R14 detects quorum sensing molecules from cariogenic Streptococcus mutans and mediates innate immune responses in gingival epithelial cells, FASEB J., 2021, 35, e21375 CrossRef CAS PubMed.
- W. S. Roland, R. J. Gouka, H. Gruppen, M. Driesse, L. van Buren, G. Smit and J. P. Vincken, 6-methoxyflavanones as bitter taste receptor blockers for hTAS2R39, PLoS One, 2014, 9, e94451 CrossRef PubMed.
- R. E. Kuhre, N. J. Wewer Albrechtsen, C. F. Deacon, E. Balk-Moller, J. F. Rehfeld, F. Reimann, F. M. Gribble and J. J. Holst, Peptide production and secretion in GLUTag, NCI-H716, and STC-1 cells: a comparison to native L-cells, J. Mol. Endocrinol., 2016, 56, 201–211 CAS.
- W. S. Roland, L. van Buren, H. Gruppen, M. Driesse, R. J. Gouka, G. Smit and J. P. Vincken, Bitter taste receptor activation by flavonoids and isoflavonoids: modeled structural requirements for activation of hTAS2R14 and hTAS2R39, J. Agric. Food Chem., 2013, 61, 10454–10466 CrossRef CAS PubMed.
- A. Levit, S. Nowak, M. Peters, A. Wiener, W. Meyerhof, M. Behrens and M. Y. Niv, The bitter pill: clinical drugs that activate the human bitter taste receptor TAS2R14, FASEB J., 2014, 28, 1181–1197 CrossRef CAS PubMed.
- S. S. An and S. B. Liggett, Taste and smell GPCRs in the lung: Evidence for a previously unrecognized widespread chemosensory system, Cell. Signalling, 2018, 41, 82–88 CrossRef CAS PubMed.
- S. K. Sukumaran, B. C. Lewandowski, Y. Qin, R. Kotha, A. A. Bachmanov and R. F. Margolskee, Whole transcriptome profiling of taste bud cells, Sci. Rep., 2017, 7, 7595 CrossRef PubMed.
- C. Grau-Bove, A. Miguens-Gomez, C. Gonzalez-Quilen, J. A. Fernandez-Lopez, X. Remesar, C. Torres-Fuentes, J. Avila-Roman, E. Rodriguez-Gallego, R. Beltran-Debon, M. T. Blay, X. Terra, A. Ardevol and M. Pinent, Modulation of Food Intake by Differential TAS2R Stimulation in Rat, Nutrients, 2020, 12, 3784 CrossRef CAS PubMed.
|
This journal is © The Royal Society of Chemistry 2022 |
Click here to see how this site uses Cookies. View our privacy policy here.