DOI:
10.1039/D1FO02758A
(Paper)
Food Funct., 2022,
13, 227-241
Ergothioneine exhibits longevity-extension effect in Drosophila melanogaster via regulation of cholinergic neurotransmission, tyrosine metabolism, and fatty acid oxidation†
Received
22nd August 2021
, Accepted 3rd November 2021
First published on 20th November 2021
Abstract
Many studies have demonstrated the protective effect of ergothioneine (EGT), the unique sulfur-containing antioxidant found in mushrooms, on several aging-related diseases. Nevertheless, to date, no single study has explored the potential role of EGT in the lifespan of animal models. We show here that EGT consistently extends fly lifespan in diverse genetic backgrounds and both sexes, as well as in a dose and gender-dependent manner. Additionally, EGT is shown to increases the climbing activity of flies, enhance acetylcholinesterase (AchE) activity, and maintain the ratio of reduced glutathione (GSH) to oxidized glutathione (GSSG)of aged flies. The increase in lifespan by EGT is gut microorganism dependent. We proposed potential mechanisms of lifespan extension in Drosophila by EGT through RNA-seq analysis: preservation of the normal status of the central nervous system via the coordination of cholinergic neurotransmission, tyrosine metabolism, and peroxisomal proteins, regulation of autophagic activity by altering the lysosomal protein CTSD, and the preservation of normal mitochondrial function through controlled substrate feeding into the tricarboxylic acid (TCA) cycle, the major energy-yielding metabolic process in cells.
Introduction
Mushrooms are rich in a variety of proteins, B vitamins, fiber, immunity-enhancing sugars (β-glucans), and other biologically active compounds. As early as 2005, Dubost had discovered that mushrooms are rich in ergothioneine (EGT), and that the level of EGT is at least 10 times that of other foods.1 Mushrooms are an important food source of the sulfur-antioxidants EGT and glutathione, which researchers believe can help fight against aging and enhance health.2 The content of EGT and glutathione in mushrooms varies from species to species. Among the 13 species tested, porcini has the highest content of these two compounds.3 At the same time, studies have found that countries that have more EGT in their diets, such as France and Italy, also have a lower incidence of neurodegenerative diseases, while people in countries with low EGT in their diets, such as the United States, suffer from diseases such as Parkinson's disease (PD), and Alzheimer's disease (AD).3 As a natural amino acid, EGT has many physiological functions, such as free radical scavenging, detoxifying, maintaining DNA biosynthesis, normal cell growth, and cellular immunity.4 The ability of EGT to penetrate the blood–brain barrier may indicate the functional role of EGT in the central nervous system (CNS) and neurons.5,6 Recent studies have shown that blood EGT levels in subjects with mild cognitive impairment and PD are significantly lower than those in age-matched healthy individuals.7 Furthermore, EGT exhibits a protective effect against oxidative DNA telomeric damage and maintains telomere length by increasing the telomerase activity transiently (24–48 h).8 Shortened telomeres are associated with aging and age-related diseases.9 Taken together, the antioxidant, neuroprotective effect, and cytoprotective effect of EGT support its potential protective role in aging.10
Aging is a physiological process mediated by a variety of regulatory pathways and transcription factors, which is mainly reflected in continuous functional decline and increased risk of chronic diseases.11,12Drosophila melanogaster has been used in aging research in recent years to unveil the occurrence and development mechanism of aging. The objective of this study was to explore the role of dietary antioxidant-EGT in longevity and to identify its underlying life extension mechanism. Our research indicates a dose-dependent EGT benefit toward the lifespan of flies. The treatment of flies with 100 μM EGT improves the climbing activity of flies and has no adverse effect on the fecundity of flies. We also evaluated the effect of EGT on oxidative stress markers of flies, examined the acetylcholinesterase (AChE) activity of brain tissue, and elaborated on how EGT causes a series of biological changes in flies through RNA-seq analysis.
Results
EGT extends Drosophila lifespan
EGT is known for its potent antioxidant properties. We hypothesized that EGT may counteract oxidative damage and therefore extend the lifespan of flies. To test the generality of the effect of EGT on lifespan, we examined frequently used the wild-type laboratory strains, Canton S and yw. We administrated the flies with different concentrations of EGT (10 mM, 1 mM, 100 μM, 10 μM, 1 μM, 100 nM, 0 nM). Of the seven concentrations of EGT, no significant increase in longevity was observed for both female and male flies exposed to low concentrations of EGT, 1 μM, and 100 nM. Significant lifespan extension occurred at 10 μM, 100 μM, and 1 mM in repeated experiments, with no significant differences in survival between flies exposed to the three effective concentrations (Fig. 1 and Table S1†). In addition, EGT also increased the lifespan of male flies, but less so than that of females (Fig. 1 and Table S1†). And EGT reduced the lifespan of flies at high concentrations of 1 mM and 10 mM. The detailed results are shown in Table S1.† These data suggest that the response of lifespan to EGT in D.melanogaster occurred to a greater degree in female rather than male flies. In subsequent experiments, we used 100 μM EGT, which led to the greatest increase in the median lifespan of both sexes.
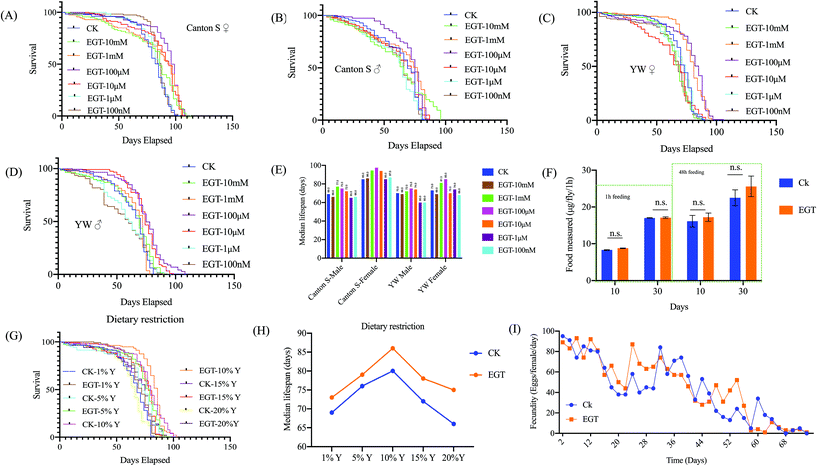 |
| Fig. 1 Effects of EGT on lifespan and fecundity in various standard laboratory strains. (A–E) EGT treatment extends the lifespan of Canton S and yw females and males. Compared to flies on control food (0 mM EGT), flies on 10 μM and 100 μM EGT food exhibit increased median life spans. (F) The figure represents feeding observations during a 1 h period. There was no significant difference in the feeding intake of flies on EGT food compared to flies on food not containing EGT. Feeding intake measurements, using 10- and 30-day old females, were carried out on blue-dye-containing standard food that was supplemented or not containing 100 μM EGT. (G) Survival curves for yw females against yeast concentration (1%Y, 5%Y, 10%Y, 15%Y, and 20%Y) in SYA food and on the same food concentrations but supplemented with 100 μM EGT. (H) The median lifespan of yw on DR food was plotted. Statistical analysis of the median lifespan of the DR experiment is listed in Table S2.† (I) No change was observed in the fecundity of females on 100 μM EGT food. Data are given as the mean number of eggs laid per female per day ± SEM. t-Test p values between egg-laying under different conditions are p > 0.05. For each time point and each condition, eggs from ten vials containing ten flies per vial were counted. The lifespan studies were independently repeated three times with n = 5, 20–30 flies each, with in total over 100 flies per group showing similar results. One representative lifespan is shown. | |
EGT mediated extension in lifespan is independent of dietary restriction
Due to the special smell of certain substances, the supplementation of these substances may affect the palatability of food, thereby reducing the feeding intake of flies, to achieve an effect similar to dietary restriction (DR), thereby prolonging the lifespan of the flies. DR has been shown to extend the lifespan of a wide variety of species.13 To determine if EGT and DR act on the same or different pathways, the feeding intake assay and survival rate of yw female flies fed a DR diet supplemented with or without EGT were measured.
No significant difference in feeding intake within 1 h was observed between female flies fed the SY diet supplemented with or without 100 μM EGT (Fig. 1F). We also examined whether DR affected the lifespan of EGT-treated flies (Fig. 1H and Table S2†). EGT extended the median lifespan at all yeast concentrations tested (Fig. 1H), and the extension was lowest at the 5% yeast concentration. The highest yeast concentration (20%) shortened the lifespan of EGT-supplemented flies by 14% as opposed to 21% in the control group. To sum up, these data suggest that the pro-longevity effect of EGT is regulated at least partly through a DR-independent pathway.
Lifespan extension by EGT is abolished in axenic Drosophila
Flies treated with antibiotics did not respond to EGT consumption. The lifespan-extending effect of EGT observed under conventional conditions was abolished with antibiotic treatment (Fig. S1A†). Additionally, the antibiotic treatment resulted in a compromised climbing ability in both conventional-fed flies and EGT-fed flies (Fig. S1B–D†). In other words, the lifespan-extending effect of EGT is exhibited in a gut bacteria-dependent manner.
The effect of EGT on the fecundity
Lifespan extension in animals in some cases is associated with a decrease in fecundity. An ideal anti-aging compound should prolong the lifespan of flies without sacrificing fecundity ability. To determine if the extension of lifespan by EGT was associated with a decrease in reproduction, we determined the fecundity of female flies on the standard SY diet supplemented with 100 μM of EGT by measuring the entire lifetime egg production of yw female flies. We observed no significant differences in the lifetime egg production by flies fed with an EGT-containing diet compared to the non-supplemented controls (Fig. 1I). This result indicated that supplementation with EGT has no negative effect on the fecundity ability of flies and that the fecundity of female flies is retained.
EGT improves the physiological functions and climbing activity of adult-fed female flies
The measurement of climbing activity is a suitable method by which to examine the health status of flies, and directly reflects their muscle mass.14 We observed no difference in the climbing activity of young flies (day 10) (p > 0.05). EGT significantly improved the climbing ability of aging flies (day 30 and 50), especially for 50-day-old flies (p < 0.001) (Fig. S2A–C†).
We next determined the effect of EGT on the physiological parameters of female flies, including the bodyweight, triglycerides (TAG), adenosine triphosphate (ATP), protein, and glucose levels of female Drosophila at different stages of life (10, 30 and 50 days old), as shown in Fig. S2D–H.† As expected, a time-dependent elevation trend in the total body weight of Drosophila was observed in untreated controls (Fig. S2D†). The supplementation of EGT maintained the bodyweight of Drosophila, and there was no significant weight loss or gain in the body mass of the flies over their lifetime. The TAG levels of the flies directly reflect the level of body fat storage in Drosophila. Similarly, for body mass measurement in aged flies, the control flies also exhibited an age-dependent elevation in the TAG level, which peaked at day 50 (Fig. S2E†). On the contrary, the consumption of EGT invoked a reduction in the TAG levels of aged flies (at days 30 and 50) (Fig. S2E†). The supplementation of EGT thus regulates storage fat accumulation in aged flies.
In terms of the ATP measurements, the results show that EGT can maintain the ATP level in flies at varying stages of life. However, there was a time-dependent reduction in total ATP levels in untreated controls (Fig. S2F†). Regarding total glucose measurement, a similar age-dependent increase was also observed in untreated controls, which was at its highest in aged flies (day 50) (Fig. S2G†). In contrast to the control flies, EGT consumption rescued the glucose level of aged flies to the level of young flies (day 10). In addition, Fig. S2H† shows that the difference in protein levels was not significant between the two groups (p > 0.05).
Increase in the stress tolerance of Drosophila by EGT
Life extension is often accompanied by an increase in stress tolerance, and there is usually a causal link between the two. We, therefore, tested whether EGT-induced longevity could be in part attributable to enhanced stress tolerance. At the age of 10 days, the flies reared on diet with or without EGT were tested for tolerance to heat, desiccation, starvation, and the oxidative stresses paraquat and H2O2. We found that EGT significantly increased tolerance to heat, starvation, paraquat, and H2O2 in females (Fig. 2). Moreover, EGT exhibited a protective effect against heat, starvation, and H2O2 stresses in males, except for paraquat. And EGT treatment had no protective effect for either gender against desiccation. The detailed results are shown in Fig. 2 and Table S3.†
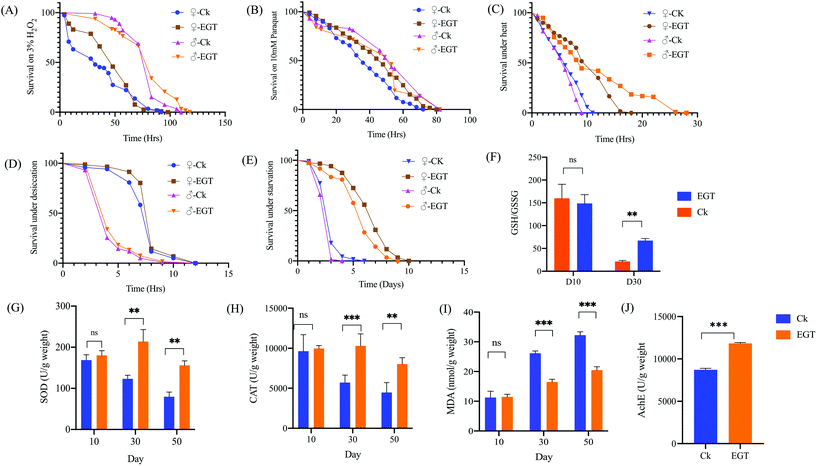 |
| Fig. 2 EGT enhances the stress resistance of the flies, and impacts the oxidative stress makers and AchE activity in agingDrosophila melanogaster yw females pretreated with 100 μM EGT survived longer under (A) H2O2, (B) paraquat, (C) heat, and (D) starvation conditions compared to untreated flies. EGT failed to protect yw males against (B) paraquat stress. And EGT failed to enhance the survival of females and males under (E) desiccation conditions. For all of the stress assays, the flies were pretreated for 2 weeks with 100 μM EGT, and the initial population was 120 flies per condition. Supplementation of EGT rescues the ratio of (F) GSH/GSSG of aging flies; oxidative stress parameters, including (G) SOD, (H) CAT, (I) MDA, were measured in female flies; EGT consumption enhanced the (J) AchE activity of yw flies. Each group contained n = 3 independent groups and the significance is indicated with (*) relative to the control group with ns: p > 0.05, *p < 0.05, **p < 0.01, and ***p < 0.001. | |
Effect of EGT on antioxidant status in the brain of D. melanogaster
As shown in ESI Table S4,† the GSH content in the brain tissue of aging flies treated with EGT (418.98 ± 3.54 μmol g−1 weight) was much higher than that of control flies (262.43 ± 3.29 μmol g−1 weight (p < 0.001). And the control group of aged flies exhibited a higher content of GSSG (12.34 ± 1.21 μmol g−1 weight) than that of the EGT supplement group (6.23 ± 0.432 μmol g−1 weight). Aged flies fed without EGT exhibited a marked reduction in the GSH/GSSG ratio. EGT treatment rescued the decline in the GSH/GSSG ratio in aged flies (Fig. 2F, p < 0.01).
EGT reduces the oxidative stress and restores the AchE activity of flies
Superoxide dismutase (SOD) and catalase (CAT), which are abundant in peroxisomes, are two indispensable antioxidant enzymes that defend against reactive oxygen species (ROS). Malondialdehyde (MDA), one of the main final products of lipid peroxidation, causes the cross-linking and polymerization of proteins, nucleic acids, and other functional macromolecules, resulting in cellular damage and dysfunction. The MDA level has widely been considered as a marker of oxidative stress.15 It can be seen from Fig. 2G–I that the levels of SOD and CAT are significantly elevated in EGT-treated aging flies in comparison with the control flies (30 and 50 days old) (p < 0.001). In addition, the MDA level of the EGT treatment group was significantly lower than that of the control aging flies (Fig. 2I).
Moreover, the brain AChE activity of aged flies fed with an EGT supplemented diet was significantly increased in comparison with the control group (Fig. 2J). Oral administration with EGT rescued the loss in the AchE activity in aging flies (p = 0.000011). AChE is an enzyme of the brain cholinergic system that hydrolyzes the neurotransmitter acetylcholine into choline and acetate in the synaptic cleft.16,17
Quality evaluation of RNA sequencing reads
RNA sequencing analysis was performed on 30-day-old female flies kept on EGT containing medium and control cornmeal food, which revealed that there are a total of 110
112
620 and 114
677
602 clean reads in the untreated flies and EGT treatment group, respectively. The Q30 base percentage of each sample is greater than 94%, and the GC content, the proportion of G and C bases out of an implied four total bases, is greater than 49%, which met the requirement for the following bioinformatics analysis. The detailed results are shown in Table S5.†
Differentially expressed genes (DEGs) in EGT-treated female Drosophila
Global transcriptional changes from normalizing the DEG data were analyzed to identify DEGs. The genes that simultaneously satisfied false discovery rate (FDR) < 0.05 and |log
2(FC)| > 1 were accepted as DEGs. FDR is the adjusted p-value to trim false positive results. DESeq analysis showed that a total of 73 differentially expressed genes were identified, including 46 upregulated and 27 downregulated genes (Fig. 3A). A volcano plot was drawn to show the global fold changes between the upregulated and downregulated genes (Fig. 3B). As shown in the volcano plot, most of the DEGs represented a slight fold change, while some of the DEGs displayed significant differences. Results with this pattern revealed that adding EGT may regulate some unknown genes. Additionally, a heatmap was drawn to present the hierarchical clustering of the DEGs among samples (Fig. 3C). As shown in the heatmap, the expression profiles between the replicates were similar, indicating that the transcriptome data were reliable.
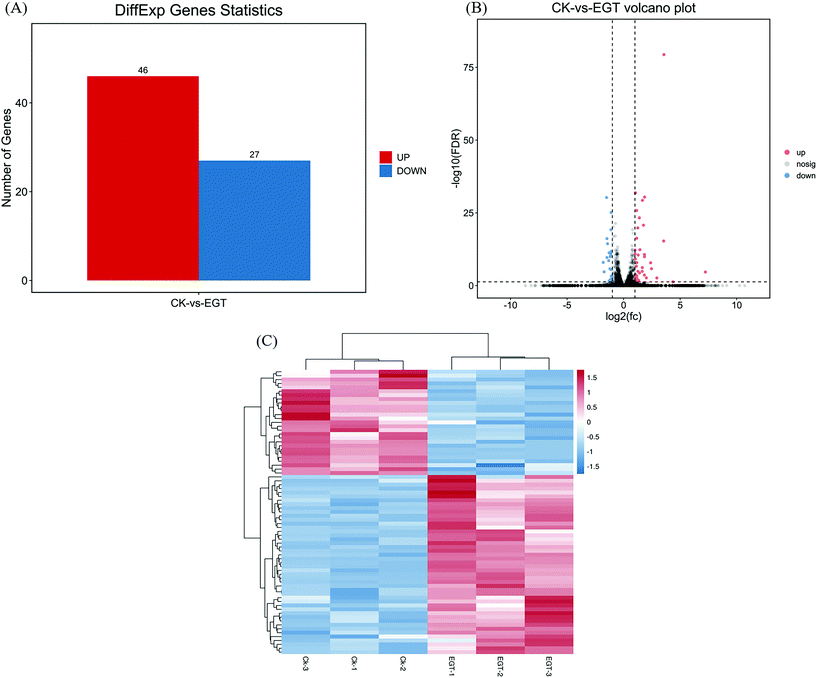 |
| Fig. 3 (A) The numbers of differentially expressed genes (DEGs) were distinguished into up-and down-regulated genes, respectively, based on the category of gene ontology terms: biological process, cellular component, and molecular function. The x-axis represents the number of DEGs, the y-axis represents the distribution of the level 2 GO terms, the green bars indicate downregulated DEGs, and the red bars indicate upregulated DEGs. (B) Volcano plot of the DEGs (CK vs. EGT) (the x-axis represents the log 2-transformed fold change. The y-axis represents the –log 10-transformed significance, the colors indicate the fold change: up-regulated DEGs, red; down-regulated DEGs, blue; non-DEGs, gray). (C) Heat map of the hierarchical clustering of the DEGs (the x-axis represents each compared sample; the y-axis represents the DEGs. The colors indicate the fold change: high, red; low, blue). | |
Functional enrichment analysis of DEGs
Gene ontology (GO) was applied to identify the properties of genes and their products in any organism. GO classifies gene products with three distinct ontologies: molecular function, cellular component, and biological process. According to this classification, the top 20 GO term entries with the smallest Q value were selected and are displayed in Fig. S3A–C.† GO enrichment analysis showed that the supplementation of EGT had the greatest impact on the biological process of Drosophila, and a weaker impact on the cellular component (as shown in Fig. 4A). For GO molecular function, the gene enrichment mainly involved catalytic activity, binding, and transporter activity (Fig. 4A). Cell, cell part, and membrane were the three main cellular components of the identified DEGs (Fig. 4A). In the biological process category, DEGs were mainly enriched in terms related to cellular, metabolic, and multicellular organismal processes (Fig. 4A).
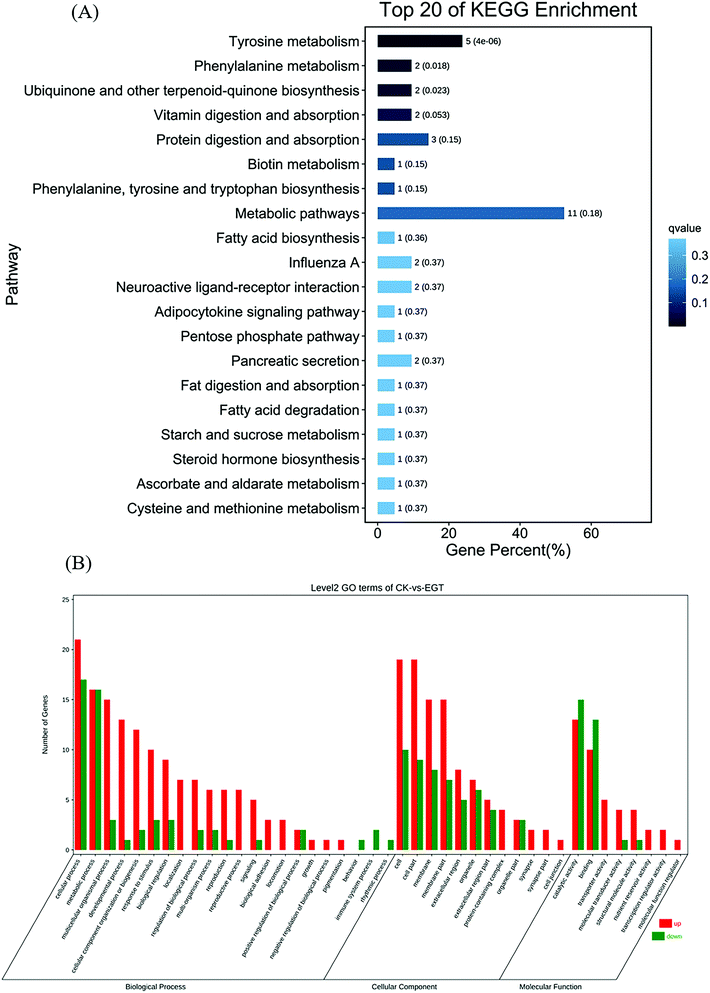 |
| Fig. 4 (A) Bar plot of the KEGG pathway enrichment analysis of DEGs between the Ck group vs. the EGT group, where the top 20 KEGG pathways with the lowest Q values are shown. The X-axis represents the proportion of the number of DEGs described by different functions to the total number of DEGs. The Y-axis represents the different pathways. (B) The numbers of up-or down-regulated DEGs in pairwise comparisons between Ck and EGT, where the green bars indicate downregulated DEGs and the red bars indicate upregulated DEGs. | |
We further explored the functional implications of these DEGs in the life extension of EGT by Kyoto Encyclopedia of Genes and Genomes (KEGG) enrichment pathway analysis. The top 20 KEGG pathways with the smallest Q-value are displayed in Fig. 4B. The results show that in the comparison between EGT-treated Drosophila and untreated controls, three pathways, metabolic pathways, tyrosine metabolism, and protein digestion and absorption, were significantly enriched (Fig. 4B). The detailed results and exact p-values are shown in Table S6.†
Regulation of cholinergic neurotransmission
Of interest is the observation that the transcripts of FBgn0038641/SLC5A7, encoding high-affinity choline transporter (ChT), are significantly upregulated in EGT supplemented flies (Table 1). ChT is a membrane transporter protein that is responsible for the transporting of choline to neurons. Choline is the necessary reagent for the production of acetylcholine (ACh) in neurons, a neurotransmitter of the central and peripheral nervous system that plays an important role in cognitive, autonomic, and motor functions.18 Research has suggested that mutation in SLC5A7 is associated with multiple neuromuscular disorders, such as congenital myasthenic syndrome.19 ChT, therefore, is indirectly associated with the regular functionality of CNS.
Table 1 The expression levels of DEGs obtained from RNA-seq analysis
ID |
CG ID |
Symbol |
Description |
Log 2(FC) |
Significance |
The column of “log 2 FC (fold change)” represents the base-two logarithm value of the fold change of the gene expression; “+” means up-regulated genes; “−” means down-regulated genes. The significance is indicated with (*) relative to the control group with ns: p > 0.05, *p < 0.05, **p < 0.01, and ***p < 0.001. |
FBgn0030558 |
CG1461 |
TAT |
Tyrosine aminotransferase [EC: 2.6.1.5] |
−1.184 |
*** |
FBgn0037697 |
CG9363 |
GstZ2 |
Maleylacetoacetate isomerase (EC: 5.2.1.2) |
−1.086 |
*** |
FBgn0040211 |
CG4779 |
Hgo |
Homogentisate1,2-dioxygenase [EC: 1.13.11.5] |
−1.323 |
*** |
FBgn0036992 |
CG11796 |
Hpd |
4-Hydroxyphenylpyruvate dioxygenase, isoform A [EC: 1.13.11.27] |
−1.505 |
*** |
FBgn0286723 |
CG4500 |
Hll |
ACSBG [EC: 6.2.1.3] |
−1.807 |
*** |
FBgn0032055 |
CG13091 |
Sgp |
Alcohol-forming fatty acyl-CoA reductase [EC: 1.2.1.84] |
−1.108 |
*** |
FBgn0051661 |
CG31661 |
CG31661 |
Cathepsin D [EC: 3.4.23.5] |
1.25 |
*** |
FBgn0038641 |
CG7708 |
ChT |
High-affinity choline transporter |
1.069 |
*** |
Downregulation of the tyrosine degradation pathway
Transcriptomics data analysis showed a surprising discovery, which found that genes involved in tyrosine degradation were significantly downregulated in EGT treated Drosophila (Table 1). The significantly down-regulated DEGs were GstZ2 (FBgn0037697, decreased by 1-fold), CG1461 (FBgn0030558, decreased by 1.2-fold), hgo (FBgn0040211, decreased by 1.3-fold), and Hpd (FBgn0036992, decreased by 1.5-fold).
CG1461 is a functional ortholog of mammalian tyrosine aminotransferase (TAT). TAT encoded by CG1461 is the first rate-limiting enzyme in the tyrosine degradation pathway, and it catalyzes the conversion of tyrosine to 4-hydroxyphenylacetone (Fig. S4†). In addition to common protein functions, tyrosine is an important precursor for the synthesis of nerve mediators (such as dopamine, octopamine, and tyramine), and it can also eventually generate acetoacetate and fumarate through the tyrosine degradation pathway (Fig. S4†). Acetate and fumarate can be added to the TCA cycle and ultimately participate in the energy metabolism pathway of mitochondria.20
Regulation of peroxisome proteins and fatty acid β oxidation
Remarkably, the RNA-seq analysis showed that two DEGs associated with peroxisome and fatty acid β oxidation (FAO), FBgn0032055 (sgp) and FBgn0286723 (hll), were downregulated in EGT treated Drosophila at day 30 (Table 1). Sgp is one of the Drosophila homologs for the human fatty acyl-CoA reductase (FAR) genes, and the membrane proteins encoded by FAR are localized to the peroxisome.21 The FAR family protein of peroxisomes is responsible for the production of long-chain fatty alcohol, which is needed for ether lipid synthesis (ELS).22 However, a possible contribution of sgp to the ELS has been largely unexplored.
In terms of the FAO, the long-chain acyl-CoA synthetase (ACS), encoded by hll, produces fatty acyl CoA and affects the mitochondrial FAO through the enzyme carnitine palmitoyl transferase (CPT), which is in charge of importing fatty acyl CoA into the mitochondria (Fig. 5). FAO is the main energy resource pathway in eukaryotic cells and declines with age.23,24 Previous research suggested that accelerated FAO extended median and maximum lifespan and increased the stress resistance of Drosophila.25 According to RNA-seq data, the downregulated hll in the present study led to reduced fatty acyl CoA levels in cytosol, but how it affected the FAO in the mitochondrial was unknown. Uptake of fatty acyl CoAs across the mitochondrial membrane requires CPTs (CPT 1 and CPT2) and the transporter protein carnitine-acylcarnitine translocase (CACT). To unveil the role played by FAO in lifespan extension by EGT, we further checked the mRNA level of FAO related genes, such as ACC, CACT, and no changes were observed in the present study (ESI 2†).
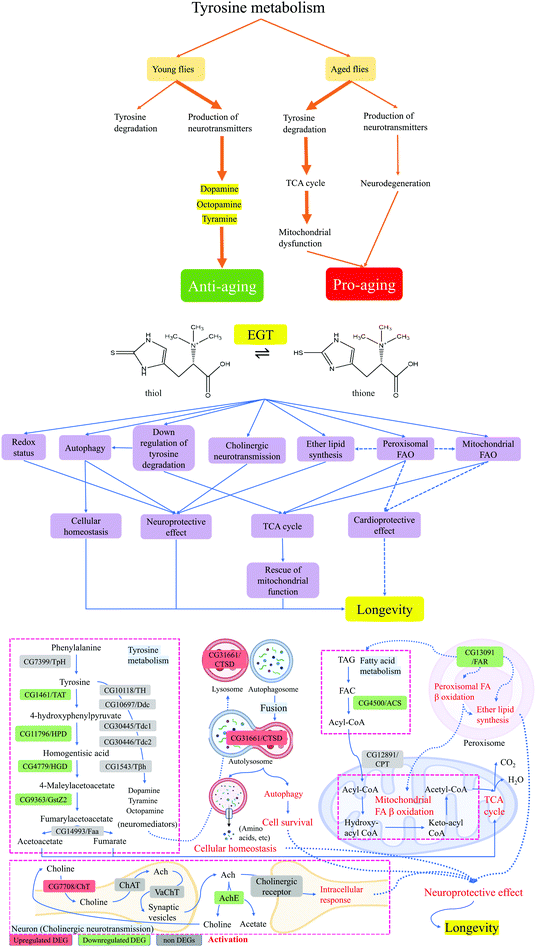 |
| Fig. 5 A schematic presentation of the postulated mechanism of how EGT mediates lifespan extension and improves fitness in Drosophila melanogaster. | |
Regulation of lysosome proteins and autophagy
The transcriptomics data suggested that the consumption of EGT increased autophagic activity by upregulating the expression of CG31661 (FBgn0051661, increased by 1.2-fold). CG31661 is homologous to mammalian cathepsin D. The lysosomal proteolytic enzyme cathepsin D (CTSD) is the main aspartic protease of the lysosome, where it is involved in protein degradation and autophagy. Several lines of evidence have shown that CTSD plays a crucial role in a variety of physiological (cell proliferation, apoptosis, and cell senescence) and pathological (AD, atherosclerosis, and cancer) conditions.26
QRT-PCR verification of RNA-seq analysis
To further verify the RNA-seq data, 8 genes represented in 5 different pathways discussed in the present study were selected to perform qRT-PCR validation (see Table 1). The qRT-PCR results show the differential expression pattern of these DEGs, verifying the reliability of the RNA-Seq data (Fig. 6). Although the fold changes of these genes in qRT-PCR were slightly different from the RNA-Seq data, they shared a similar down-regulated tendency. Overall, these qRT-PCR results show that the DEGs transcription level was consistent with the data obtained by RNA seq analysis.
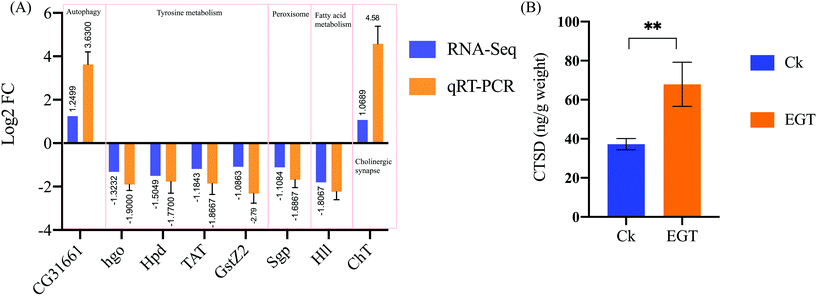 |
| Fig. 6 (A) Quantitative real-time PCR validation of the DEGs. The qRT-PCR was carried out in biological triplicate. The orange bars (RNA-seq) represent the log 2 fold change (Ck/EGT) in the transcriptome data, while the blue bars (qRT-PCR) represent the log 2 regulation of the qRT-PCR data. Each group contained n = 3 independent groups and the significance is indicated with (*) relative to the control group with ns: p > 0.05, *p < 0.05, **p < 0.01, and ***p < 0.001. (B) A commercially available ELISA kit was used to measure CTSD from homogenates prepared from yw female whole flies reared on EGT or control SYA for 30 days. Each point represents the mean ± SD of three assays. | |
EGT feeding increases CTSD levels in adult flies
As shown in Fig. 6B, the enzyme-linked immunosorbent assay (ELISA) showed that 30-day-old female flies fed with EGT (67.89 ± 11.3 ng g−1 weight) exhibit increased levels of CTSD compared with the control flies (37.23 ± 2.88 ng g−1 weight, p < 0.01). The protein level of CTSD was consistent with the data obtained by RNA seq analysis.
Discussion
Given the neuroprotective and anti-oxidative actions of EGT, some researchers have suggested that EGT may have a life-extension effect. However, to our knowledge, no study has examined the effect of EGT in any animal models of aging. Therefore, we investigated the effects of oral EGT administration on D. melanogaster and confirmed its lifespan-extending effect.
The present study showed different and interesting results. The RNA-seq analysis showed that EGT exhibits a positive impact on the health and lifespan of Drosophila via regulation in cholinergic neurotransmission, tyrosine metabolism, FAO, and autophagy.
Unexpectedly, we observed the upregulation of ChT, the choline transporter in choline neurotransmission, in EGT supplemented flies. Given the fact that choline is the direct precursor of acetylcholine (ACh), and AChE is responsible for the hydrolysis of Ach from cholinergic neurons and non-cholinergic neurons in the brain, we therefore further examined the level of AchE in aging flies to confirm our hypothesis. As expected, the increased level of AchE by EGT in the brains of flies (Fig. 2J) further confirmed the idea that the EGT-evoked neuroprotective effects contributed towards an increase in the health span and life expectancy of flies. Escalating evidence has suggested loss of AChE activity in several brain diseases, including neurodegenerative diseases.27,28 Also, positron emission tomography and autopsy studies have shown that patients with AD exhibit obvious loss of AChE in their forebrain.29–31
What is more, from a chemical structure point of view, EGT is also unusual. EGT is a thiol/thione molecule with a unique low molecular weight. GSH, an endogenous thiol, plays a neuroprotective effect in the AD animal model.32 Deficiency in neuronal GSH leads to the progression of neurogenerative diseases.33 However, GSH is difficult to transport into most cells or tissues. Given that GSH and EGT are both low molecular thiol structured antioxidants, and are present in high levels in mushrooms, we hypothesized that EGT supplementation can save the consumption of GSH. This result confirmed our hypothesis and showed that EGT rescues the reduction in the GSH/GSSG ratio (Fig. 2F). Thus, the unique thiol/thione structure of EGT also gives rise to its ability to delay the aging of flies.
Peroxisomal dysfunction is associated with the pathogenesis of various age-related diseases, such as cancer, type-2 diabetes, and neurodegeneration.34 FAO also occurs in peroxisomes when the fatty acid chains are too long to be handled by the mitochondria.21 Previous research highlighted that sgp dysfunction affects the β-oxidation of adult flies and results in increased levels of TAG and body mass.35 Based on this evidence, we believe that the downregulation of sgp induced by supplementation of EGT reflects the alterations in both peroxisomal FAO and mitochondrial FAO. However, the underlying mechanism behind its action is unknown. Taken together, the data suggest that hll and sgp are coordinated in the regulation of FAO (Fig. 5).
FAO dysfunction may be harmful to cardiac function.36 Recent studies have shown that maintaining FAO and mitochondrial integrity is essential for heart function during aging.37 Up to 50–70% of the energy (in the form of ATP production) in the heart with high energy demands comes from FAO.38 A 20-year longitudinal study showed that the level of EGT in tissues is closely related to cardiovascular health.39 A recent study suggested that EGT exhibits a cardioprotective effect in type 2-diabetic rats.40 However, the association between cardio health and consumption of EGT has not been fully explored. And whether the putative cardioprotective effect of EGT is correlated with its regulating effect on FAO remains to the established. In addition, acetyl-CoA generated by FAO enters the mitochondrial TCA cycle, where it is further oxidized to generate NADH and FADH2. The NADH and FADH2 produced by both FAO and the TCA cycle are used by the mitochondrial electron transport chain to further generate ATP. Mitochondrial dysfunction is associated with low NAD/NADH ratios, and contributes towards the aging process.41 This evidence, together with our observations, suggests that the antiaging effects of EGT can be partially mediated through the pleiotropic effect of FAO on the metabolic pathway (Fig. 5).
Additionally, the downregulated tyrosine metabolism by EGT in the present study supports the neuroprotective effect of EGT. Tyrosine metabolism not only produces substrates that participate in the TCA cycle, but also produces neurotransmitters (Fig. S4†). Neurotransmitters are essential to the function of the complex CNS.42 For example, PD is associated with low levels of dopamine.42
Previous study has demonstrated that compared with normal lifespan flies, CG1461/TAT mRNA levels in long-lived flies were significantly reduced.43 In addition, the level of TAT mRNA in aged flies was also significantly higher than that of young flies. This finding indicates that in aging flies, tyrosine is more involved in the degradation pathway and enters the TCA cycle, which eventually leads to the deterioration of mitochondrial function and the reduction of neurotransmitter levels in aging flies (Fig. 5). Therefore, we proposed that the consumption of EGT redirected the tyrosine metabolism of aged flies from the tyrosine degradation pathway into the production of neurotransmitters and resulted in either increased levels of neurotransmitters or the amelioration of mitochondrial dysfunction via decreased tyrosine feeding into the TCA cycle (Fig. 5). This is the first time that the intake of EGT has been shown to exert an effect on the tyrosine metabolism of flies.
Neurotransmitters can trigger CTSD activation that may result in cell survival or cell death, depending on specific cellular context.44 CTSD-mediated proteolysis can remove unfolded or oxidized protein aggregates through the autophagy-lysosome pathway.44 We speculated that the upregulation of CTSD by EGT is associated with the accelerated production of neurotransmitters by downregulating the tyrosine degradation pathway (Fig. 5). The up-regulated neurotransmitters activate CTSD, thereby regulating autophagy in aging flies and maintaining normal proteolytic activity (Fig. 5). The decrease in the proteolytic activity of CTSD during aging causes undigested autophagy substrates and unfolded or oxidized protein aggregates to gradually accumulate in the lysosome, consequently leading to neurodegenerative disorders. CTSD can remove the amyloid β-protein (Aβ) by shearing the β-amyloid precursor protein and inhibiting its accumulation, and inhibit the formation of fibrosis and amyloid deposits, ultimately suppressing the deterioration of AD.45 It has been documented that EGT can inhibit the occurrence of neuronal cell apoptosis induced by β-amyloid peptide and cisplatin and prevent the occurrence of related neurodegenerative diseases.46,47 Recent research has also demonstrated that EGT dose-dependently reduces Aβ-oligomerization of C. elegans, but the specific mechanism of action of EGT is unknown.46 Taking into consideration this research evidence, we speculated that EGT upregulated autophagic activity by regulating the level of CTSD, thereby maintaining cellular homeostasis, reducing and delaying the occurrence of neurogenerative diseases, and extending the lifespan of flies in the present study (Fig. 5).
Concerning CNS pathology, it is interesting to note that ELS and the peroxisomal FAO pathway, two important metabolic pathways in peroxisomes, also play vital roles in the CNS.48 As shown in Fig. 5, sgp was involved in both ELS and peroxisomal FAO. Ether lipids have unique physical properties that impact many aspects of cell biology. Defects in ELS induce CNS pathologies, such as autism, PD, and AD.21,48 Previous study stated that elevations of certain ether lipids were observed in AD.49 We speculated that the downregulation of sgp by EGT was to maintain the normal level of ether lipids in aging flies to play a neuroprotective role, thereby promoting the health and prolonging the lifespan of the flies (Fig. 5).
The beneficial role of EGT in neurodegenerative diseases has been extensively researched, but the detailed mechanism of action remains elusive. Taken together, combining all the data and evidence we discussed above, the mechanisms by which this neuroprotective action of EGT is achieved may be through the integrative action of cholinergic neurotransmission, FAO, autophagy, and peroxisomal metabolic pathways (Fig. 5).
Notably, the present RNA-seq data reveals that EGT exhibits a lifespan extension effect in a way that is different from other canonical longevity pathways, such as IIS, AMPK, etc. However, our current research remains fragmentary. These pathways should be confirmed in terms of protein and enzymatic activity levels in further research. Peroxisomes are still among the more mysterious subject in the aging field21,50 And the primary mode of action by which EGT induce changes in peroxisomes is unknown. Further systematic research is needed to decipher the functional correlation between alterations in peroxisomes and lifespan. Similarly, although the role of lysosomes, autophagy and FAO have been extensively discussed in the aging field, how these alterations are initiated by EGT remains elusive. And whether the regulating of FAO by EGT reflects the putative cardioprotective effect of EGT remains unsolved.
Conclusion
We administered 100 μM of EGT to adult female Drosophila, and evaluated oxidative stress markers, the AchE activity of flies, and employed a transcriptomic study to compare longevity-related gene expression between EGT treated flies and untreated controls, verifying the level of CTSD by ELISA assay. The major findings in this study were as follows. (1) EGT effectively prolongs the lifespan of Drosophila and improves the fitness and stress tolerance of flies. (2) Sexual dimorphism is observed in flies, where EGT-mediated lifespan extension is smaller in males than in females. (3) EGT induces lifespan extension in Drosophila via a mechanism that is distinct from that of dietary restriction. (4) EGT regulates cholinergic activity in aging flies by upregulating the level of ChT and enhancing AchE activity. (5) EGT supplementation prolongs the lifespan of flies by maintaining the generation of neurotransmitters and downregulating the tyrosine degradation pathway to prevent a high level of tyrosine from entering the TCA cycle. (6) Upregulated CTSD reflects that the longevity of Drosophila mediated by EGT includes the upregulation of autophagy to maintain the normal function of the CNS. (7) The regulation of FAO by EGT may contribute towards the maintenance of mitochondrial function in aged flies.
Materials and methods
Fly stocks and husbandry
The D. melanogaster wildtype laboratory strain yw was kindly provided by the Hoffman Institute of Immunology, Guangzhou University of Traditional Chinese Medicine. All stocks were kept under controlled conditions at 25 °C in a 12 h light/dark cycle at ∼60% humidity. For experiments, the standard cornmeal food was prepared by boiling cornmeal (78 g), sucrose (32 g), glucose (63.2 g), and yeast extract (32 g) in 1 L of distilled water for 20 min. The media was cooled to 60–65 °C before adding 15 mL of tegosept per liter. For all of the experiments, except for lifespan, only female D. melanogaster was used, ergothioneine (>95% purity, extracted from multiple types of edible mushrooms, purchased from Tianjin Sinonocy Biological Technology Co., Ltd, China) was dissolved in double distilled H2O at a concentration of 10 mM.
Lifespan assay
The newly emerged flies were mated for 48 h and then sorted according to their sex. Flies were maintained in vials containing either control or an EGT supplemented diet (ranging from 10 nM to 10 mM EGT) at a density of 25–30 flies per vial. The flies were transferred to fresh food vials every 2–3 days without anesthesia and scored for deaths during the whole lifetime. All lifespan experiments were repeated at least twice. The detailed life tables are shown in ESI 2.† Survival analyses were performed using log-rank (Mantel-Cox) testing and the Gehan-breslow-Wilcoxon test. Increases in median lifespan were analyzed using an analysis of variance (ANOVA) multiple comparisons test.
Fecundity assay
The fecundity assay was determined using a modified version of that described in ref. 51. 24 h after the mating of female flies and male flies, the male flies were removed, and the female flies were used in the subsequent experiments. Each vial contained 10 female flies, which were transferred to new vials with fresh food every 2 days. The number of dead flies was counted at each transfer. The vials containing old food and embryos were kept for embryo counting. The number of embryos laid each time until all flies died was recorded. The fecundity assay was repeated 3 times. Lifetime fecundity was calculated by dividing the total number of eggs laid in each vial by the number of female flies at the beginning of the experiment.
Feeding intake assay
The feeding intake method was employed to measure food intake with modifications according to that described in ref. 52. Female flies were first treated with a diet supplemented with 0 or 100 μM EGT for 10 and 30 days. Subsequently, 20 flies were simultaneously transferred to a medium containing 2.5% Brilliant Blue FCF (Sigma, CAS 3844-45-9). After 1 h or 48 h of feeding, 20 flies were frozen at −20 °C and then homogenized in 1 ml of distilled water. The whole fly homogenates were centrifuged at 5000 rpm for 2 min at room temperature, and the supernatant was then filtered through a 0.22 μm filter (Millipore Corporation, Bedford) for later use. The absorbance of the supernatants was measured at 629 nm. 0.1 g of food containing 2.5% Brilliant Blue FCF in 1 ml of distilled water was homogenized, and then centrifuged at 5000 rpm for 2 min. Supernatants were filtered through a 0.22 μM Millipore filter and diluted in a two-fold gradient. The absorbance of each gradient was measured and a standard curve was plotted. The actual feeding intake of each group of flies in 1 h was normalized to the standard curve.
Stress tolerance assay
To analyze their resistance to stress, flies were reared and housed for lifespan experiments. Flies were pretreated with EGT at 100 μM for 15 days and then transferred to a vial containing 20 mM of paraquat in 5% sucrose (Sigma) or 3% H2O2 in 5% sucrose for oxidative stress assays. To determine heat stress, 2-week-old female flies were kept at 30 °C and their deaths recorded every 1.5 h. The desiccation assay was measured by rearing adult females in empty vials at 25 °C at a density of 20–30 flies per vial. A diet containing 1.5% agar and 10% nipagin was prepared for the starvation assay. For the starvation assay, the number of deaths of flies was recorded each day. And for other stresses, the deaths were recorded every 1.5–3 h, and the survival curve was plotted. All of the stress experiments were performed in three biological replicates (100–120 flies in each). The statistical significance of the data was assessed according to log-rank criteria.
Generation and verification of germ-free flies
To eliminate the gut microbiota and consequently generate axenic flies, 500 μg mL−1 ampicillin sodium salt (Solarbio), 50 μg mL−1 tetracycline hydrochloride (Cat No.284592, J&K scientific Ltd), and 200 μg mL−1 rifamycin SV sodium salt were added to the food medium. PCR amplification of the 16S rRNA gene and plate culturing was performed to verify the absence of bacteria in axenic flies. For the plate culturing assay, 100 μL of fly homogenates were cultured in an MRS plate and then incubated at 37 °C for 48 h. A bacterial universal primer was used in PCR verification, and the PCR products were run on 1% agarose gel to confirm the absence of bacteria in axenic individuals.
Climbing activity
The climbing activity was determined in female flies fed a control or an EGT-supplemented diet for 10, 30, and 50 days according to ref. 53. The experiment was independently repeated three times at the same time point to mitigate possible circadian rhythm confound. The experimental flies were transferred to separate empty graduated cylinders without anesthesia and were tapped to the bottom of the glass cylinder. A 2 min trial was conducted for each replicate and the number of flies was counted above the target line (180 ml line of the 250 ml graduated cylinder, 17.5 cm) every 30 s. The percentage of flies above the target line was calculated and each percentage at each time point was plotted. A Student's t-test was performed to determine the significant differences in activity between the control flies and the EGT fed flies.
Measurement of metabolic parameters in Drosophila
Bodyweight was assessed by weighing 20 female flies in replicates of three at the time of anesthetization. Total glucose measurements were taken from three independent groups from whole-body homogenates of female Drosophila. The total protein content was quantified using a BCA protein assay reagent kit (Pierce, Thermo Fisher Scientific). For the measurement of total glucose content, the whole-body homogenate was heat-treated for 10 min at 95 °C to remove any complexes. Glucose levels were measured in 20 μL of whole-body homogenate using a glucose assay kit (Solarbio, Beijing, China) according to the manufacturer's instructions. Whole-body triglycerides were determined in 40 μL of whole-body homogenate using a triglycerides assay kit (Solarbio, Beijing, China) according to the manufacturer's instructions. The total ATP level was determined using the ATP assay kit (Solarbio, Beijing, China). For each treatment group, three independent samples of flies were used. The resultant quantification of metabolic markers was standardized against the weight of the flies.
Determination of oxidative stress markers and AchE activity
To evaluate the oxidation and antioxidant levels of flies, the activities of SOD, CAT and MDA levels were measured using a microplate reader according to the manufacturer's recommendations.
The SOD activity was tested using a xanthine–xanthine oxidase reaction to generate superoxide radicals and nitrotetrazolium blue (NBT) reduction as an indicator of superoxide production.54 Homogenates from 25 female flies were prepared and the procedures were followed according to the SOD assay kit (Solarbio, Beijing, China) to determine the SOD activity. Briefly, the absorbance of reduced NBT was recorded at 560 nm, and the quantification of the SOD activity was normalized to the weight of each sample.
CAT activity was assessed based on the reaction of CAT with methanol in the presence of hydrogen peroxide to generate formaldehyde, as adapted from ref. 55. The absorbance at 240 nm was monitored for 0 and 1 min and quantification was normalized to the weight of the sample.
The MDA level was measured using an MDA assay kit (Solarbio, Beijing, China). MDA reacts with thiobarbituric acid (TBA) to produce a color reaction and its absorbance was determined at 532 nm. An MDA standard curve was plotted using the values obtained from the dilutions of appropriate MDA standards. The amount of MDA present in the flies was calculated from the standard curve and then standardized against the weight of the flies.
The AChE activity in brain tissue was measured using the microplate reader method using an AchE assay kit (Solarbio, Beijing, China). AchE catalyzes the hydrolysis of Ach to produce choline. Choline reacts with dithiop-nitrobenzoic acid (DTNB) to produce 5-mercaptonitrobenzoic acid (TNB). The absorbance of TNB was measurement at 412 nm, and quantification was normalized to the weight of each sample.
Determination of the GSH/GSSG ratio in D. melanogaster brains
The contents of glutathione (GSH) and glutathione disulfide (GSSG) were determined in female flies fed a control or an EGT-supplemented diet for 10 and 30 days using a GSH and GSSG assay kit (Beoytime, Beijing, China). Homogenates from 100 D. melanogaster brains were prepared by adding 5% 5-sulfosalicylic acid and centrifuged at 8000g at 4 °C for 10 min. The supernatants were kept for further steps. A standard curve was generated by making a dilution series of known amounts of oxidized glutathione standard. Quantification of GSH and GSSG contents were normalized to the weight of brain tissue.
CTSD measurement by ELISA assay
ELISA was used to quantitatively measure cathepsin D (CTSD) in adult flies. The whole flies were washed with pre-chilled phosphate-buffered saline (PBS, 0.01 M, pH = 7.4) to remove any residues. The sample was thoroughly homogenized on ice in PBS. The homogenate was sonicated to further lyse the tissue cells, and then centrifuged at 5000g for 10 min. The remaining supernatant was used for further analysis. A standard dose-response curve was developed by making a dilution series. Sample homogenates, standards, biotin-labeled detection antibodies, and HRP enzyme conjugate were added into the wells of a 96-well plate coated with insect CTSD capture antibodies. The plates were incubated for 1 h at 25 °C with gentle shaking, and the wells were then washed with wash buffer and TMB development solution was added. The plates were then incubated at 25 °C for 10 min. Stop solution was added and the absorbance was measured using a microplate reader at a wavelength of 450 nm. The intensity of the color was positively correlated with the insect CTSD in the tissues.
RNA sequencing analysis
The newborn control and EGT treated flies were placed on the respective cornmeal diets for 30 days, with three replica vials for each treatment. The 30-day-old female flies were subject to total RNA extraction. Total RNA was extracted using a Trizol reagent kit (Invitrogen, Carlsbad, CA, USA) according to the manufacturer's protocol. RNA quality was assessed on an Agilent 2100 Bioanalyzer (Agilent Technologies, Palo Alto, CA, USA) and checked using RNase-free agarose gel electrophoresis. The eukaryotic mRNA was enriched using Oligo(dT) magnetic beads after the extraction of total RNA. Then the enriched mRNA was further fragmented using fragmentation buffer and randomly primed during the first strand synthesis by reverse transcription. Second-strand cDNA was synthesized from DNA polymerase I, RNase H, dNTP and buffer to create double-stranded cDNA fragments. Then the cDNA fragments were purified using a QiaQuick PCR extraction kit (Qiagen, Venlo, The Netherlands), end-repaired by Klenow and T4 DNA polymerases, poly(A) was added, and then they were ligated to Illumina paired-end sequencing adapters. The ligation products were followed via size selection by agarose gel electrophoresis, PCR amplification, and were then sequenced using an Illumina HiSeq2500 by Gene Denovo Biotechnology Co. (Guangzhou, China).
Differentially expressed genes
RNA differential expression analysis was performed using the DESeq2 software between two different groups (and by edgeR between the two samples). The transcripts with the parameters of a corrected p value of <0.05 and absolute fold change of ≥ 2 were considered as DEGs.
GO analyses of DEGs
GO enrichment analysis provided all of the GO terms that were significantly enriched in DEGs compared to the genome background and the DEGs that corresponded to biological functions were filtered. GO has three distinct ontologies: molecular function, cellular component, and biological process. The GO enrichment of the screened DEGs was performed using the DAVID online tool (https://david.ncifcrf.gov/). Significantly enriched GO terms in DEGs compared to the genome background were defined by hypergeometric testing. The GO terms with FDR ≤ 0.05 were defined as significantly enriched GO terms in DEGs.
KEGG pathway enrichment analysis
To identify the key metabolic pathways, we performed KEGG analysis for all of the genes. All of the genes with KEGG annotations in the entire genome were used as a background for enrichment analyses. Pathways with an FDR of ≤0.05 were defined as significantly enriched pathways in DEGs.
RNA extraction and cDNA reverse transcription synthesis
Total RNA was isolated from 20 homogenized whole flies using Trizol (Invitrogen, A33250) according to the manufacturer's instructions. cDNA was synthesized from 500 ng of RNA measured using the ND-1000 Nanodrop (ThermoFisher Scientific, Canada) using the One-Step gDNA Removal and cDNA Synthesis kit (Transgene, Beijing, China) according to the manufacturer's instructions.
Quantitative real-time PCR validations of DEGs
Expression of various genes was performed with the PerfectStartTM Green qPCR SuperMix kit (Transgene, Beijing, China) using a QuantStudio™ 3 Real-Time PCR System (Applied Biosystems™, ThermoFisher Scientific). Each sample was an average of three technical replicates and statistical measures were conducted using 6 biological replicates. Primers, their sequences, and annealing temperatures are listed in Table S7.† The target gene expression was calculated using the comparative 2-ddcT method relative to the expression of the housekeeping gene Actin-5C.
Statistics
All data were analyzed using the GraphPad Prism 9.0 software, including the lifespan data, and statistical significance was tested using the t-test method. P < 0.05 was considered as significantly different, and p < 0.01 was considered as extremely significantly different. Log-rank testing was used for statistical significance analysis of the lifespan assay data. Fluorescence quantitative qPCR results were calculated using the 2-ddct method, and significant differences were analyzed using the t-test. The data are expressed as mean ± standard deviation.
Abbreviations
ACS | Acyl-CoA synthetase |
AchE | Acetyl cholinesterase |
CoA | Coenzyme A |
CPT | Carnitine palmitoyl transferase |
CTSD | Cathepsins D |
Ddc | Dopa decarboxylase |
Faa | Fumarylacetoacetase |
FAC/FA | Fatty acids |
FAO | Fatty acid β oxidation |
FAR | Fatty acid reductase |
GstZ2 | Maleylacetoacetate isomerase |
HGD | Homogentisate 1,2-dioxygenase |
Hn | Phenylalanine-4-hydroxylase |
Hpd | 4-Hydroxyphenylpyruvate dioxygenase |
TAG | Triglycerides |
TAT | Tyrosine aminotransferase |
TCA | Tricarboxylic acid cycle |
Tdc | Tyrosine decarboxylase |
TβH | Tyramine-β-hydroxylase |
TH | Tyrosine hydroxylase |
Author contributions
H-YP, L-QG, and J-FL designed the study. H-YP performed data analysis and wrote the manuscript. W-GH and M-ZT performed lifespan assay and RNA extraction and assisted in data analysis. Z-WY and Q-WZ helped revise the manuscript critically for important intellectual content. All authors approved the manuscript before publication.
Conflicts of interest
There are no conflicts to declare.
Acknowledgements
We would like thank Dr You-Lu Lin for providing laboratory wild type D. melanogaster, and thank the staff of Gene Denovo who performed the sequencing of the genomic libraries. This study was supported by the National Natural Science Foundation of China (grant numbers 31772373, 32072646, 32001832, 31901693, 31801918).
References
- N. J. Dubost, R. B. Beelman, D. G. Peterson and D. J. Royse, Identification and quantification of ergothioneine in cultivated mushrooms by Liquid Chromatography Mass Spectroscopy, Int. J. Med. Mushrooms, 2006, 8(3), 215–222 CrossRef CAS.
- R. B. Beelman, M. D. Kalaras and J. P. Richie Jr., Micronutrients and Bioactive Compounds in Mushrooms: A Recipe for Healthy Aging?, Nutr. Today, 2019, 54(1), 16–22 CrossRef.
- M. D. Kalaras, J. P. Richie, A. Calcagnotto and R. B. Beelman, Mushrooms: A rich source of the antioxidants ergothioneine and glutathione, Food Chem., 2017, 233, 429–433 CrossRef CAS.
- B. Halliwell, I. K. Cheah and R. M. Y. Tang, Ergothioneine - a diet-derived antioxidant with therapeutic potential, FEBS Lett., 2018, 592, 3357–3366 CrossRef CAS PubMed.
- K. Ohira, R. Takeuchi, H. Shoji and T. Miyakawa, Fluoxetine-Induced Cortical Adult Neurogenesis, Neuropsychopharmacology, 2013, 38, 909–920 CrossRef CAS PubMed.
- T. Ishimoto, N. Nakamichi, H. Hosotani, Y. Masuo, T. Sugiura and Y. Kato, Organic Cation Transporter-Mediated Ergothioneine Uptake in Mouse Neural Progenitor Cells Suppresses Proliferation and Promotes Differentiation into Neurons, PLoS One, 2014, 9 CrossRef CAS PubMed.
- T. Hatano, S. Saiki, A. Okuzumi, R. P. Mohney and N. Hattori, Identification of novel biomarkers for Parkinson's disease by metabolomic technologies, J. Neurol., Neurosurg. Psychiatry, 2016, 87, 295–301 CrossRef PubMed.
- P. Samuel, M. Tsapekos, N. de Pedro, A. G. Liu, J. Casey Lippmeier and S. Chen, Ergothioneine Mitigates Telomere Shortening under Oxidative Stress Conditions, J. Diet. Suppl., 2020, 1–14, DOI:10.1080/19390211.2020.1854919.
- A. K. Koliada, D. S. Krasnenkov and A. M. Vaiserman, Telomeric aging: mitotic clock or stress indicator?, Front. Genet., 2015, 6, 82 Search PubMed.
- B. N. Ames, Prolonging healthy aging: Longevity vitamins and proteins, Proc. Natl. Acad. Sci., 2018, 115(43), 10836–10844 CrossRef CAS PubMed.
- S. Ahadi, W. Y. Zhou, S. Rose, M. R. Sailani, K. Contrepois, M. Avina, M. Ashland, A. Brunet and M. Snyder, Personal aging markers and ageotypes revealed by deep longitudinal profiling, Nat. Med., 2020, 26(1), 83–90 CrossRef CAS PubMed.
- C. Kenyon, The plasticity of aging: insights from long-lived mutants, Cell, 2005, 120, 449–460 CrossRef CAS PubMed.
- S. D. Katewa and P. Kapahi, Dietary restriction and aging, 2009, Aging Cell, 2010, 9, 105–112 CrossRef CAS PubMed.
- M. A. Jones and M. Grotewiel, Drosophila as a model for age-related impairment in locomotor and other behaviors, Exp. Gerontol., 2011, 46, 320–325 CrossRef PubMed.
- S. Gaweł, M. Wardas, E. Niedworok and P. Wardas, Malondialdehyde (MDA) as a lipid peroxidation marker, Wiad. Lek., 2004, 57, 453–455 Search PubMed.
- F. Carvajal and N. Inestrosa, Interactions of AChE with Aβ Aggregates in Alzheimer’s Brain: Therapeutic Relevance of IDN 5706, Front. Mol. Neurosci., 2011, 4, 19 CAS.
- H. Soreq and S. Seidman, Acetylcholinesterase—new roles for an old actor, Nat. Rev. Neurosci., 2001, 2, 294–302 CrossRef CAS PubMed.
- L. M. Sanders and S. H. Zeisel, Choline: Dietary Requirements and Role in Brain Development, Nutr. Today, 2007, 42, 181–186 CrossRef PubMed.
- J. M. Pardal-Fernández, M. C. Carrascosa-Romero, S. Álvarez, M. C. Medina-Monzón, M. B. Caamaño and C. de Cabo, A new severe mutation in the SLC5A7 gene related to congenital myasthenic syndrome type 20, Neuromuscular Disord., 2018, 28, 881–884 CrossRef PubMed.
- R. Sharma and A. J. P. Ramanathan, The Aging Metabolome Biomarkers to Hub Metabolites, Proteomics, 2020, 20, 5–6 CrossRef PubMed.
- C. Pridie, K. Ueda and A. J. Simmonds, Rosy Beginnings: Studying Peroxisomes in Drosophila, Front. Cell Dev. Biol., 2020, 8, 835 CrossRef CAS PubMed.
- M. Honsho, S. Asaoku, K. Fukumoto and Y. Fujiki, Topogenesis and homeostasis of fatty acyl-CoA reductase 1, J. Biol. Chem., 2013, 288, 34588–34598 CrossRef CAS PubMed.
- S. K. Krisans, R. M. Mortensen and P. B. Lazarow, Acyl-CoA synthetase in rat liver peroxisomes. Computer-assisted analysis of cell fractionation experiments, J. Biol. Chem., 1980, 255, 9599–9607 CrossRef CAS.
- I. Singh, O. Lazo, G. S. Dhaunsi and M. Contreras, Transport of fatty acids into human and rat peroxisomes. Differential transport of palmitic and lignoceric acids and its implication to X-adrenoleukodystrophy, J. Biol. Chem., 1992, 267, 13306–13313 CrossRef CAS.
- S.-H. Lee, S.-K. Lee, D. Paik and K.-J. Min, Overexpression of Fatty-Acid-beta-Oxidation-Related Genes Extends the Lifespan of Drosophila melanogaster, Oxid. Med. Cell. Longevity, 2012, 2012, 854502 Search PubMed.
- O. Mijanovic, A. I. Petushkova, A. Brankovic, B. Turk, A. B. Solovieva, A. I. Nikitkina, S. Bolevich, P. S. Timashev, A. Parodi and A. A. Zamyatnin Jr., Cathepsin D-Managing the Delicate Balance, Pharmaceutics, 2021, 13(6), 837 CrossRef PubMed.
- E. J. Mufson, S. E. Counts, S. E. Perez and S. D. Ginsberg, Cholinergic system during the progression of Alzheimer's disease: therapeutic implications, Expert Rev. Neurother., 2008, 8, 1703–1718 CrossRef CAS PubMed.
- M. Méndez, M. Méndez-López, L. López, M. A. Aller, J. Arias and J. L. Arias, Acetylcholinesterase activity in an experimental rat model of Type C hepatic encephalopathy, Acta Histochem., 2011, 113, 358–362 CrossRef PubMed.
- K. L. Davis, R. C. Mohs, D. Marin, D. P. Purohit, D. P. Perl, M. Lantz, G. Austin and V. Haroutunian, Cholinergic markers in elderly patients with early signs of Alzheimer disease, J. Am. Med. Assoc., 1999, 281, 1401–1406 CrossRef CAS PubMed.
- H. Henke and W. Lang, Cholinergic enzymes in neocortex, hippocampus and basal forebrain of non-neurological and senile dementia of Alzheimer-type patients, Brain Res., 1983, 267, 281–291 CrossRef CAS PubMed.
- D. E. Kuhl, R. A. Koeppe, S. Minoshima, S. E. Snyder, E. P. Ficaro, N. L. Foster, K. A. Frey and M. R. Kilbourn, In vivo mapping of cerebral acetylcholinesterase activity in aging and Alzheimer's disease, Neurology, 1999, 52, 691–699 CrossRef CAS PubMed.
- J. B. Schulz, J. Lindenau, J. Seyfried and J. Dichgans, Glutathione, oxidative stress and neurodegeneration, Eur. J. Biochem., 2000, 267, 4904–4911 CrossRef CAS PubMed.
- S. W. Mercer, S. La Fontaine, C. G. Warr and R. Burke, Reduced glutathione biosynthesis in Drosophila melanogaster causes neuronal defects linked to copper deficiency, J. Neurochem., 2016, 137, 360–370 CrossRef CAS PubMed.
- C. M. Cipolla and I. J. Lodhi, Peroxisomal Dysfunction in Age-Related Diseases, Trends Endocrinol. Metab., 2017, 28, 297–308 CrossRef CAS PubMed.
- S. H. Merkling, H. Riahi, G. J. Overheul, A. Schenck and R. P. van Rij, Peroxisome-associated Sgroppino links fat metabolism with survival after RNA virus infection in Drosophila, Sci. Rep., 2019, 9, 2065 CrossRef PubMed.
- K. W. Chung, Advances in Understanding of the Role of Lipid Metabolism in Aging, Cells, 2021, 10(4), 880 CrossRef CAS PubMed.
- A. A. Johnson and A. Stolzing, The role of lipid metabolism in aging, lifespan regulation, and age-related disease, Aging Cell, 2019, 18, e13048 CrossRef CAS PubMed.
- E. J. Lesnefsky, Q. Chen and C. L. Hoppel, Mitochondrial Metabolism in Aging Heart, Circ. Res., 2016, 118, 1593–1611 CrossRef CAS PubMed.
- E. Smith, F. Ottosson, S. Hellstrand, U. Ericson, M. Orho-Melander, C. Fernandez and O. Melander, Ergothioneine is associated with reduced mortality and decreased risk of cardiovascular disease, Heart, 2020, 106, 691–697 CrossRef CAS PubMed.
- A. Dare, A. A. Elrashedy, M. L. Channa and A. Nadar, Cardioprotective Effects and In-Silico Antioxidant Mechanism of L-Ergothioneine In Experimental Type-2 Diabetic Rats, Cardiovasc. Hematol. Agents Med. Chem., 2021 DOI:10.2174/1871525719666210809122541.
- L. R. Stein and S. Imai, The dynamic regulation of NAD metabolism in mitochondria, Trends Endocrinol. Metab., 2012, 23, 420–428 CrossRef CAS PubMed.
- L. Nguyen, J.-M. Rigo, V. Rocher, S. Belachew, B. Malgrange, B. Rogister, P. Leprince and G. Moonen, Neurotransmitters as early signals for central nervous system development, Cell Tissue Res., 2001, 305, 187–202 CrossRef CAS PubMed.
- A. A. Parkhitko, D. Ramesh, L. Wang, D. Leshchiner, E. Filine, R. Binari, A. L. Olsen, J. M. Asara, V. Cracan, J. D. Rabinowitz, A. Brockmann and N. Perrimon, Downregulation of the tyrosine degradation pathway extends Drosophila lifespan, eLife, 2020, 9, 27 CrossRef PubMed.
- V. Stoka, V. Turk and B. Turk, Lysosomal cathepsins and their regulation in aging and neurodegeneration, Ageing Res. Rev., 2016, 32, 22–37 CrossRef CAS PubMed.
- C. N. Suire, S. O. Abdul-Hay, T. Sahara, D. Kang, M. K. Brizuela, P. Saftig, D. W. Dickson, T. L. Rosenberry and M. A. Leissring, Cathepsin D regulates cerebral Aβ42/40 ratios via differential degradation of Aβ42 and Aβ40, Alzheimer's Res. Ther., 2020, 12, 80 CrossRef CAS PubMed.
- I. K. Cheah, L. T. Ng, L. F. Ng, V. Y. Lam, J. Gruber, C. Y. W. Huang, F. Q. Goh, K. H. C. Lim and B. Halliwell, Inhibition of amyloid-induced toxicity by ergothioneine in a transgenic Caenorhabditis elegans model, FEBS Lett., 2019, 593, 2139–2150 CrossRef CAS PubMed.
- T.-Y. Song, C.-L. Chen, J.-W. Liao, H.-C. Ou and M.-S. Tsai, Ergothioneine protects against neuronal injury induced by cisplatin both in vitro and in vivo, Food Chem. Toxicol., 2010, 48, 3492–3499 CrossRef CAS PubMed.
- F. Dorninger, S. Forss-Petter and J. Berger, From peroxisomal disorders to common neurodegenerative diseases - the role of ether phospholipids in the nervous system, FEBS Lett., 2017, 591, 2761–2788 CrossRef CAS PubMed.
- J. W. Pettegrew, K. Panchalingam, R. L. Hamilton and R. J. McClure, Brain membrane phospholipid alterations in Alzheimer's disease, Neurochem. Res., 2001, 26, 771–782 CrossRef CAS PubMed.
- M. Islinger, A. Voelkl, H. D. Fahimi and M. Schrader, The peroxisome: an update on mysteries 2.0, Histochem. Cell Biol., 2018, 150, 443–471 CrossRef CAS PubMed.
- A. I. Barnes, S. Wigby, J. M. Boone, L. Partridge and T. Chapman, Feeding, fecundity and lifespan in female Drosophila melanogaster, Proc. R. Soc. B, 2008, 275, 1675–1683 CrossRef PubMed.
- S. A. Deshpande, G. B. Carvalho, A. Amador, A. M. Phillips, S. Hoxha, K. J. Lizotte and W. W. Ja, Quantifying Drosophila food intake: comparative analysis of current methodology, Nat. Methods, 2014, 11, 535–540 CrossRef CAS PubMed.
- S. T. Madabattula, J. C. Strautman, A. M. Bysice, J. A. O’Sullivan, A. Androschuk, C. Rosenfelt, K. Doucet, G. Rouleau and F. Bolduc, Quantitative Analysis of Climbing Defects in a Drosophila Model of Neurodegenerative Disorders, J. Visualized Exp., 2015, 100, 52741 Search PubMed.
- D. R. Spitz and L. W. Oberley, An assay for superoxide dismutase activity in mammalian tissue homogenates, Anal. Biochem., 1989, 179, 8–18 CrossRef CAS PubMed.
- L. H. Johansson and L. A. Borg, A spectrophotometric method for determination of catalase activity in small tissue samples, Anal. Biochem., 1988, 174, 331–336 CrossRef CAS PubMed.
Footnote |
† Electronic supplementary information (ESI) available. See DOI: 10.1039/d1fo02758a |
|
This journal is © The Royal Society of Chemistry 2022 |