DOI:
10.1039/D1FO01174J
(Paper)
Food Funct., 2022,
13, 255-269
Camel whey protein (CWP) ameliorates liver injury in type 2 diabetes mellitus rats and insulin resistance (IR) in HepG2 cells via activation of the PI3K/Akt signaling pathway
Received
16th April 2021
, Accepted 5th August 2021
First published on 12th December 2021
Abstract
This research investigated the effects of camel whey protein (CWP) treatment on type 2 diabetes mellitus (T2DM) rats and insulin resistance (IR) HepG2 cell models. Body weight and fasting blood glucose were observed in type 2 diabetes mellitus (T2DM) rats every week, and biochemical parameters in serum samples were evaluated after 6 weeks. Antioxidant activity in the liver was estimated, and histological examination of the liver tissues was conducted. After CWP treatment, the glucose uptake and lipid accumulation were examined in insulin-resistant HepG2 cells. Our results indicated that CWP mitigated the body weight loss, reversed dyslipidemia, and inhibited the inflammatory response, in T2DM rats. Meanwhile, it protected the liver from being injured by reducing the level of oxidative stress. In the CWP group, the pathological changes were significantly reduced, while the liver lobule structure, liver cell arrangement, as well as congestion, edema, and vacuolization were improved. Our results from quantitative real-time PCR and western blot analyses showed that CWP could up-regulate the expression levels of insulin receptor substrate-2 (IRS-2), phosphoinositide3-kinase (PI3K), protein kinase B (AKT), and glycogen synthase (GS). An active protein component CWP8 was isolated and identified, which was shown to be able to stimulate glycogen synthesis and ameliorate lipid accumulation in IR HepG2 cells. These data indicate that CWP and CWP8 might act as potential natural products regulating glucose and lipid metabolism in T2DM.
1. Introduction
Diabetes mellitus (DM) is a metabolic disease characterized by chronically increased blood glucose levels. In recent years, DM has become one of the most severe threats to public health worldwide due to the sharp rise in mortality and the increase in healthcare expenses.1 According to the 2019 Diabetes Epidemic Atlas of the International Diabetes Federation, at present, there are 463 million diabetic patients throughout the world, and the number is predicted to reach 700 million by 2045.2 Diabetes mellitus can be subdivided into type 1 diabetes mellitus (T1DM) and type 2 diabetes mellitus (T2DM). Impaired insulin signal and decreased insulin sensitivity caused by insulin resistance (IR) are highly associated with the occurrence and development of T2DM.3 The liver plays a vital role in maintaining metabolic homeostasis and controlling the regulation of glucose and lipid metabolism. IR in the liver would induce glucose homeostasis imbalance and lipid accumulation, thus causing liver insulin insensitivity. Given the prevalence of liver damage in diabetes complications, improving liver insulin resistance might have a potential in the treatment of diabetes.4
Camel whey protein (CWP), as a nutritional supplement of amino acids and biologically active protein, has been documented to exert therapeutic effects under various pathological conditions. The beneficial effects of CWP includes stimulating innate immunity and adaptive immunity, as well as anti-inflammatory, anti-cancer, anti-diabetic, anti-bacterial, and anti-viral activities. CWP has also been shown to be able to decrease oxidative stress, enhance immune system function, and increase glutathione levels.5 Previous findings have shown that CWP and its specific components can improve diabetes-induced biochemical and histopathological abnormalities,6 including hyperlipidemia,7 non-alcoholic fatty liver disease (NAFLD),8,9 impaired liver function, and IR.3,10 CWP supplementation can ameliorate impaired wound healing in diabetic patients by decreasing blood sugar levels, oxidative stress, and inflammatory response.11 CWP could not only reduce the blood sugar levels, levels of the free radical diphenylpicrylhydrazyl (DPPH), and lipid peroxidation in diabetic mice, but also protect the neurons of the central neural system (CNS) against oxidative stress and improve the neurobehavior in diabetic mice.12,13
Our previous study has shown that CWP can effectively improve glucose and lipid metabolism, as well as liver tissue damage in diabetic mice induced by streptozotocin (STZ).14 By up-regulating the expression levels of PI3K and Akt, CWP can effectively improve the morphology, proliferation, and apoptosis of palmitic acid-damaged MIN6 cells.15 In this study, the effects of CWP on the PI3K/Akt signaling pathway were investigated, in T2DM rats and IR HepG2 cell models. Moreover, the possible underlying molecular mechanism was also analyzed.
2. Materials and methods
2.1 Materials and reagents
Streptozotocin (STZ) was purchased from Sigma (Cambridge, MA, USA). Metformin hydrochloride (Met) was purchased from Bristol-Myers Squibb (Shanghai, China). High-fat feed (HFD) was purchased from Beijing Huafukang Co. Ltd. Low-sugar DMEM, phosphate buffer solution (pH 7.2), and 0.25% trypsin were purchased from Hyclone Co. (Logan, USA). Fetal bovine serum (FBS) was purchased from BI Co. (Israel). Palmitic acid and Oil red O and glycogen PAS staining kits (cell-specific) were purchased from Beijing Solarbio Technology Co., Ltd (Beijing, China). The glucose determination kit was purchased from Shanghai Rongsheng Biopharmaceutical Co., Ltd (Shanghai, China). Primary antibodies against IRS-2, PI3K, p-PI3K, Akt, p-Akt, GSK3β, GS, FoxO1, and G6Pase were purchased from Abcam (Cambridge, MA, USA). Total cholesterol (TC), triacylglycerol (TG), high-density lipoprotein cholesterol (HDL-C), low-density lipoprotein cholesterol (LDL-C), superoxide dismutase (SOD), glutathione peroxidase (GSH-Px), malondialdehyde (MDA), IL-10, IL-6, and TNF-α detection kits were purchased from Nanjing Jiancheng Biological Engineering Institute Co., Ltd (Nanjing, China).
2.2. Preparation of the sample
The raw camel milk (mixed samples of normal camel milk from 10 camels collected from the outskirts of Urumqi, Xinjiang) was centrifuged at 5000 rpm/20 min, and the fat was discarded to obtain the skimmed milk. The skimmed milk was then incubated in a water bath at 4 °C for 20 min. Its pH was adjusted to 4.6 with glacial acetic acid–water solution (v/v = 1
:
9) and it was kept at 4 °C overnight. On the second day, the skimmed milk was subsequently centrifuged at 8000 rpm/20 min and the supernatant was collected. The collected supernatant was freeze-dried to obtain camel whey protein (CWP). According to Table 1, different concentrations of ammonium sulfate were added to the obtained supernatant, and graded and salted out to obtain camel whey protein components named by numbers from 1 to 8, namely CWP1 to CWP8 (CWP1–8).
Table 1 Ammonium sulfate precipitation table
Camel whey protein group |
Ammonium sulfate saturation |
CWP1 |
0–20% |
CWP2 |
20%–30% |
CWP3 |
30%–40% |
CWP4 |
40%–50% |
CWP5 |
50%–60% |
CWP6 |
60%–70% |
CWP7 |
70%–80% |
CWP8 |
80%–100% |
2.3 Animals and experimental design
Male SD rats (SPF, weight 180–220 g) were acquired from the Animal Experiment Center of Xinjiang Medical University and maintained there. All animal experimental protocols used in this study were approved by the Institutional Animal Care and Use Committee of the Xinjiang Medical University (IACUC-20200620-1) and complied with the National Institutes of Health Guidelines for the Care and Use of Laboratory Animals (NIH publications no. 8023, revised 1978). After one week of adaptive feeding in an SPF environment, the rats were divided into four groups:
Group I (control group, Con) received normal feed;
Group II (diabetes model group, DM) received HFD;
Group III (metformin hydrochloride, Met) received HFD;
Group IV (camel whey protein, CWP) received HFD and was administered with 200 mg kg−1 day−1 camel whey by intragastric gavage.
Six weeks later, group II, group III, and group IV were rendered diabetic by intraperitoneal injections of streptozotocin (STZ) in citrate buffer at the dose of 35 mg kg−1. Group I was given the same dose of citrate buffer. Only animals with average fasting blood glucose (FBG) higher than 11.1 mmol L−1 after one week of the induction of diabetes were included in the research. Group II was treated with normal saline and group III was treated with metformin hydrochloride (200 mg per kg body wt, i.g.). Group IV rats were treated with CWP (200 mg per kg body wt, i.g.). There were no less than 10 biological replicates per group. All the animals were fed with HFD. Simultaneously, group I rats received normal feed, which was used for baseline measurements. The rats in all groups were weighed every week, and FBG was detected by caudal vein blood sampling.
2.4 Blood and tissue collection
After the experiment, SD rats were anaesthetized with Zoletil (75 mg per kg body wt, i.p.). Blood was collected from the abdominal aorta and separated by centrifugation at 3000 rpm/15 minutes. Then the supernatant was collected and kept at −80 °C. Meanwhile, the abdominal cavity was dissected immediately to obtain the liver tissue. Partial liver tissues were fixed in 4% paraformaldehyde solution for histopathological examination, and the rest were stored at −80 °C for further analysis.
2.5. Determination of oxidative stress levels in the liver
The liver tissue was separated from liquid nitrogen and homogenized in a pre-cooled PBS buffer. The homogenized tissue fluid was mixed with 2.3% KCl solution and then centrifuged at 3000 rpm/15 min for separation. The supernatant was gathered and used for the determination of SOD, GSH-Px, and MDA levels.
2.6. Cell cultures
HepG2 cells were provided by the Xinjiang Key Laboratory of Bioresources and Genetic Engineering. The cells were cultured in DMEM containing 10% fetal bovine serum, and cultured in an incubator containing 5% CO2 at 37 °C.
2.7. Cell counting MTT assay
We used the 3-(4,5-dimethylthiazol-2-yl)-2,5-diphenyltetrazolium bromide (MTT) method to determine cell viability. Briefly, HepG2 cells were plated into 96-well plates with 1 × 105 cells per well and incubated in an incubator overnight. The cells were then cultured in DMEM medium containing different concentrations of CWP for 12 hours. Subsequently, MTT work solution (0.50 mg mL−1 concentration) was added to every well and the cells were cultured for 4 h. After that, the medium was discarded and 150 μL DMSO was added to each well. The absorbance of each well at 490 nm was measured using an ELX808 microplate reader (BioTek Instruments, USA).
2.8. Determination of glucose uptake
The uptake of glucose in HepG2 cells was detected using a glucose oxidase assay kit (glucose oxidase method). Briefly, HepG2 cells were plated into 96-well plates at a concentration of 1 × 105 per well in an incubator containing 5% CO2 at 37 °C. Subsequently, the CWP sample was diluted with culture medium to the desired concentration, and incubated with the cells for 12 h. Untreated cells were used as a blank control and 0.001 mmol L−1 metformin-treated cells were used as a positive control. The glucose concentration in the cell supernatant was measured using a glucose measurement kit.
2.9. Induction of an insulin resistant HepG2 cell model
The model for inducing insulin resistance has been improved by referring to the method of Peng Jing et al.16 According to the cell viability and cell morphology and glucose uptake, the optimal treatment concentration and time of palmitic acid to induce HepG2 insulin resistance were determined and an insulin resistant HepG2 cell model was established.
2.10. Determination of lipid accumulation in IR HepG2 cells
The cells that grew to 70–80%confluence were digested, and the digested cells were diluted to prepare a cell suspension of 1 × 105 mL−1 and inoculated in a 24-well plate to establish an insulin-resistant HepG2 cell model. HepG2 cells were treated with CWP for 12 h. After treatment, HepG2 cells were fixed with 4% fixative for 30 min and washed 3 times with PBS buffer, immediately rinsed with 60% isopropanol for 30 s followed by Oil Red O staining for 30 min, washed with PBS, and immediately observed and photographed using a microscope (Olympus, Tokyo, Japan).
2.11. Determination of glycogen synthesis in IR HepG2 cells
The cells that grew to 70–80% confluence were digested, and the digested cells were diluted to prepare a cell suspension of 1 × 105 mL−1 and inoculated in a 24-well plate to establish an insulin-resistant HepG2 cell model. HepG2 cells were treated with CWP for 12 h. PAS staining was used to detect glycogen synthesis in HepG2 cells. The treated HepG2 cells were fixed with fixative for 10 min and then washed with water and dried. A solution of periodate was added to the cells and the cells were left at room temperature for 15–20 minutes, followed by two rinses each with tap water and distilled water. Schiff reagent was added to the cells and the cells were placed in a dark place at room temperature for 10–20 min. The cells were washed with sodium sulfite solution, which was added dropwise twice for 2 min each time. Mayer hematoxylin staining solution was added to the cells, and after staining, the cells were washed with water for 1 to 2 min, and then washed with distilled water three times and immediately observed with a microscope (Leica, Wetzlar, Germany) and photographed.
2.12. Quantitative real-time PCR (qRT-PCR) analysis
The total RNA was extracted from the cells using TRIzol reagent (Tiangen Biotech, Beijing, China) and complementary DNA (cDNA) was synthesized using a Fast Quant cDNA reagent kit (Takara Biomedical Technology, Beijing, China). After that, the cDNA was invoked as the template to amplify the target genes using Roche Light Cycler 480 (Roche, USA) and a Talent qPCR PreMix (SYBR Green) kit (Tiangen Biotech, Beijing, China). The internal reference gene was established for each sample, and three biological replicates were carried out. The primer sequences are given in Table 2.
Table 2 Primer sequence of the RT-qPCR gene
Gene |
Primer |
Sequence |
IRS2 |
Forward primer |
5′-CATCGACTTCCTGTCCCATCA-3′ |
Reverse primer |
5′-CCCATCCTCAAGGTCAAAGG-3′ |
PI3K |
Forward primer |
5′-GGCTTGGACCTGCGAAT-3′ |
Reverse primer |
5′-TTGTTGAAGGCTGCTGTGGC-3′ |
Akt |
Forward primer |
5′-AGTGACTCCTCCACGACTGAG-3′ |
Reverse primer |
5′-GGTGACTGTGTGAGCGACTTC-3′ |
GSK-3β |
Forward primer |
5′-TCGTCCATCGATGTGTGGTC-3′ |
Reverse primer |
5′-TTGTCCAGGGGTGAGCTTTG-3′ |
GS |
Forward primer |
5′-TTGCCAGAATGCACGCAGAA-3′ |
Reverse primer |
5′-TGCCTGCATCATCTGTTGAC-3′ |
FoxO1 |
Forward primer |
5′-AAGAGGCTCACCCTGTCGCA-3′ |
Reverse primer |
5′-ACTGTTGTTGTCCATGGACG-3′ |
G6Pase |
Forward primer |
5′-CATTGACACCACACCCTTTGC-3′′ |
Reverse primer |
5′-CCCTGTACATGCTGGAGTTGAG-3′ |
SREBP1c |
Forward primer |
5′-CTCCGGCCACAAGGTACACA-3′ |
Reverse primer |
5′-GAGGCCCTAAGGGTTGACACAG-3′ |
GAPDH |
Forward primer |
5′-CCACCCATGGCAAATTCCATGGCA-3′ |
Reverse primer |
5′-TCTAGACGGCAGGTCAGGTCCAC-3′ |
2.13. Western blot analysis
The total proteins of HepG2 cells were obtained utilizing an enhanced RIPA lysis buffer purchased from Boster Biological Technology (Wuhan, China). Protein samples were separated by SDS-PAGE and transferred to PVDF membranes, and then blocked with 5% fat-free milk for 1 h at room temperature. Afterward, the membranes were incubated with primary antibodies against IRS-2, p-PI3K, and p-Akt and incubated at 4 °C overnight. The membranes were washed with TBST buffer and incubated with secondary antibodies for 2 h at room temperature. Membranes were visualized using an ultrasensitive ECL chemiluminescent reagent (Saigo Biotech). The band intensities were analyzed using ImageQuant LAS 4000 imaging systems (GE, USA). The levels of protein were then normalized to β-actin expression.
2.14. Protein identification of camel whey protein 8 (CWP8)
The sample (CWP8) was incorporated into 30 mL SDT buffer (4% SDS, 100 mM DTT, 150 mmol L−1 Tris-HCl, pH 8.0), and was centrifuged at 10
000 rpm/30 min for separation in a water bath at 100 °C for 10 min. The supernatant was taken and the protein was quantified by the BCA method. According to the quantitative results, 300 μg proteins were digested following the filter-aided sample preparation (FASP digestion) protocol. The digested samples were analyzed by chromatography–mass spectrometry. A Q Exactive mass spectrometer (Thermo Scientific, USA) and an EasynLC (Thermo Fisher Scientific, USA) were used for the analysis.
2.15. Statistical analysis
All the experimental data are presented as the mean ± SD and were analyzed using appropriate statistical methods using GraphPad Prism 8.3 software. To analyze the differences between 2 groups, t-test was used, while one-way ANOVA was applied for multiple comparisons. A P-value of less than 0.05 was considered to indicate statistical significance. The statistical methods used and the corresponding P values for the data are shown in each figure panel and are included in the figure legends.
3. Results
3.1. Effects of CWP on body weight, fasting blood glucose (FBG), and fasting insulin (FINS) levels in T2DM rats
One week after the intraperitoneal injection of STZ, SD rats showed typical symptoms of diabetes such as polydipsia, polyphagia, body weight loss, and increased fasting blood glucose levels, confirming the successful establishment of the diabetic model. Along with the illness development, the body weight loss of the DM group became more significant, compared with the control group (Fig. 1A). Compared with the DM rats, the body weights of the treated rats significantly increased over time. However, there were no significant differences among the control rats, the DM rats treated with CWP and the DM rats treated with metformin hydrochloride (Met HCl). Moreover, the FBG levels of the control group decreased, within the normal range. The FBG levels of the DM group were significantly higher than the control group (P < 0.01) (Fig. 1B). Compared with the control group, the FINS levels in the DM group significantly decreased (P < 0.01) (Fig. 1C). These results suggest that the treatment of CWP could effectively increase the serum insulin levels in T2DM rats, inhibiting the body weight loss and FBG level increase.
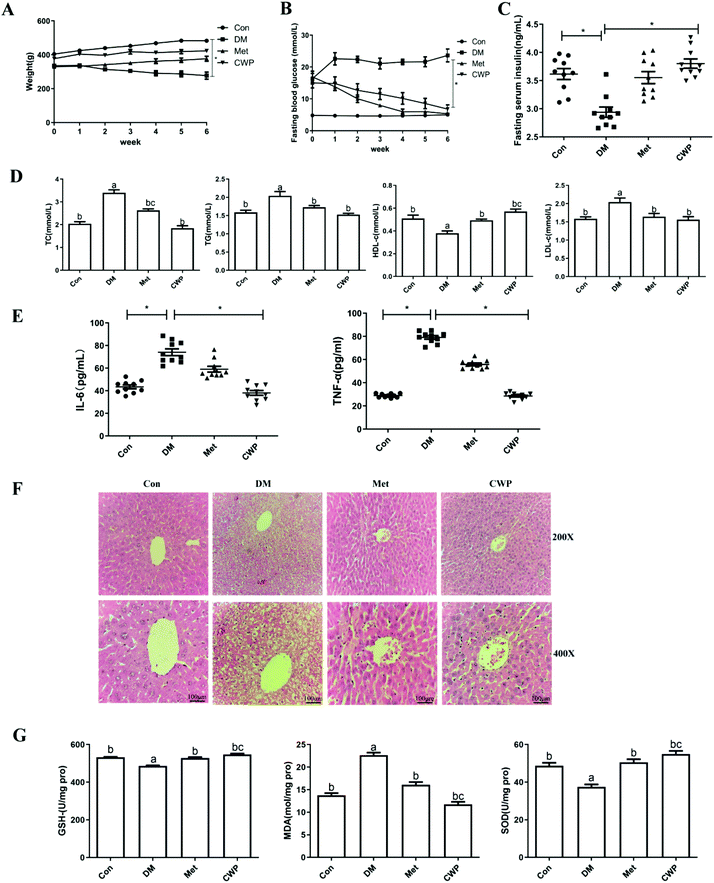 |
| Fig. 1 Camel whey protein attenuated liver injury in type 2 diabetes mellitus rats induced by STZ and HFD diet. (A) Body weight. n = 10 per group. (B) Fasting blood glucose levels. n = 10 per group. (C) Fasting insulin levels. n = 10 per group. (D) Liver lipid (TG, TC, LDL-C and HDL-C) levels. n = 10 per group. (E) Inflammatory cytokine (IL-6 and TNF-α) levels. n = 10 per group. (F) Hepatic pathological sections were measured by H&E staining in the indicated groups. n = 6 per group. Scale bar, 100 μm. (G) Liver oxidative stress and injury (SOD, GSH-Px, and MDA) levels. | |
3.2 Effect of CWP on dyslipidemia and inflammatory cytokine levels in T2DM rats
Dyslipidemia is a crucial feature of T2DM.17 Our results showed that the serum-TC, TG, HDL-C and LDL-C levels in the DM group were significantly different from the control group (P < 0.05) (Fig. 1D). However, the CWP treatment significantly reduced the serum levels of TC, TG, and LDL-C, compared with the DM group. Moreover, the HDL-C levels in the CWP and Met groups were higher than that in the DM group. Inflammation has been widely recognized as an important cause for T2DM.18 Therefore, the impact of CWP on inflammatory cytokines in T2DM rats was investigated. Our results showed that, compared with the control group, the DM group showed a significant increase in the serum IL-6, IL-10, and TNF-α levels. In contrast, the CWP treatment inhibited the inflammatory response, as evidenced by the significant reduction in the IL-6 and TNF-α levels (Fig. 1E). These data indicated CWP supplementation could improve dyslipidemia and inflammatory responses in T2DM rats.
3.3. Effect of CWP on liver injury in T2DM rats
Liver plays a major role in glucose and lipid metabolism. It has been shown that diabetes can cause liver damage in rats.19 As shown in Fig. 1F, the tissue texture of the rats in the control group was normal, in terms of liver lobule structure, and neat lines of liver cells, with no obvious pathological changes. Moreover, in the liver of STZ-induced T2DM rats, damage to hepatocytes was observed. The structure of liver lobules was blurred, and the arrangement of liver cells was disorganized, with many ballooning hepatocytes. Oxidative stress is a key cause for the pathogenesis of liver damage in T2DM. As shown in Fig. 1G, DM induced a 1.6-fold increase in the content of MDA, as compared to the control group. Meanwhile, at the antioxidant defense, the levels of SOD and GSH-Px decreased by 8% and 6%, respectively, compared with the control group. After the CWP treatment, the pathological changes in the liver tissue were significantly mitigated, and the liver lobule structure and liver cell arrangement, congestion, edema, and vacuolization were also improved. Compared with the DM group, the levels of SOD and GSH-Px increased, while the level of MDA decreased in the CWP group, indicating that CWP would be able to inhibit liver oxidative stress. These results suggest that the CWP treatment can improve liver damage by inhibiting oxidative stress in T2DM rats.
3.4. Effects of CWP on the expression of the PI3K/Akt signaling pathway in T2DM rats
In order to further explore the possible molecular mechanism of CWP in improving liver injury in T2DM rats, the effects of CWP on the mRNA and protein expression levels of the key molecules in the PI3K/Akt pathway were investigated. As shown in Fig. 2, compared with the control group, the mRNA and protein expression levels of IRS2, AKT, PI3K, and GS significantly decreased, and the mRNA and protein expression levels of FOXO1 and G6Pase increased, in the DM group (P < 0.05). The CWP treatment effectively counteracted these above changes. These results suggest that the PI3K/Akt pathway might be involved in the CWP-mediated pathways in regulating glycolipid metabolism in diabetic rats.
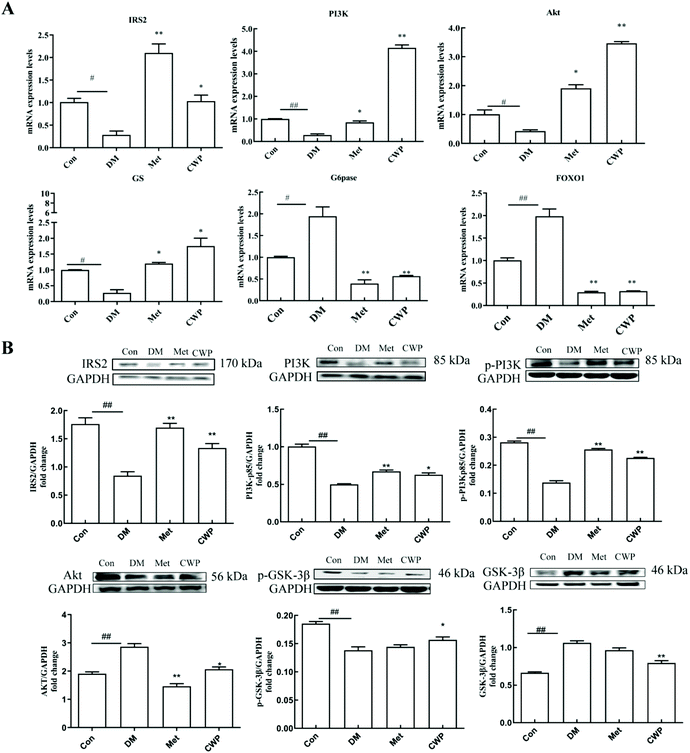 |
| Fig. 2 Camel whey protein ameliorates liver injury in type 2 diabetes mellitus rats induced by STZ and HFD diet via activation of the PI3K/Akt signaling pathway. (A) qPCR analysis of the transcript levels of genes related to the PI3K/Akt signaling pathway (IRS2, PI3K, Akt, FOXO1, G6Pase, and, SREBP1c). Gene expression was normalized to Actb mRNA levels. n = 6 per group. (B) Western blotting of proteins involved in the PI3K/Akt signaling pathway (IRS2, PI3K, p-PI3K, Akt, p-GSK-3β and GSK-3β). GAPDH served as a loading control. The data are presented as the mean ± SD. # indicates a significant difference between the control group and the DM group; #P < 0.05, ##P < 0.01. * indicates a significant difference between the DM group and the CWP group; *P < 0.05, **P < 0.01. | |
3.5 Establishment of the IR HepG2 cell model
Palmitic acid (PA) is a commonly used inducer for in vitro insulin resistance. In this study, different concentrations of PA were used to intervene in the HepG2 cells for 12 and 24 h, respectively, and the cell viability and glucose uptake were then analyzed. As shown in Fig. 3A, after the PA treatment for 12 h, the PA concentration >1.000 mmol L−1 significantly inhibits the HepG2 cell proliferation (P < 0.01). Moreover, different concentrations of PA reduced the cell glucose uptake by 17.4%, 26.3%, 37.5%, and 42.7%, 51.2% and 60.5%, respectively (P < 0.01) (Fig. 3B). As shown in Fig. 3C, after the PA treatment of HepG2 cells for 24 h, the PA concentration >0.100 mmol L−1 can significantly inhibit the HepG2 cell proliferation (P < 0.01). The glucose uptake levels of the cells were reduced by 19.1%, 35.3%, 39.4%, 46.3%, and 51.7%, respectively (P < 0.01). For the HepG2 cells treated with 0.1000 mmol L−1 PA for 12 hours, the glucose consumption was significantly inhibited, with no significant effects on cell viability and morphology. Therefore, the following conditions were used for establishing the IR model of HepG2 cells: induction with palmitic acid (PA) at the concentration of 0.100 mmol L−1 for 12 h.
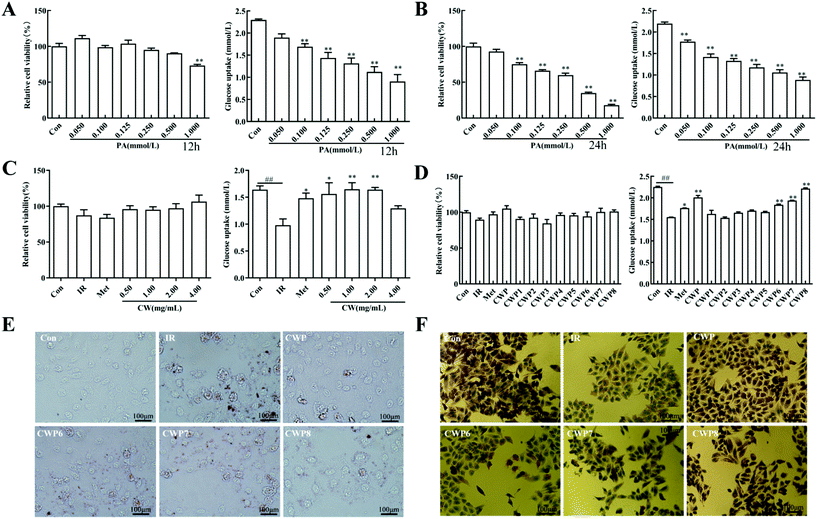 |
| Fig. 3 Effect of camel whey protein on lipid accumulation and glucose uptake in palmitic acid (PA)-induced insulin-resistant (IR) HepG2 cells. (A) Cell viability and glucose uptake in the HepG2 cells stimulated with different concentrations (0.050, 0.100, 0.150, 0.250, 0.500 and 1.000 mmol L−1) of PA for 12 h. n = 3 per group. (B) Cell viability and glucose uptake in the HepG2 cells stimulated with different concentrations (0.050, 0.100, 0.150, 0.250, 0.500 and 1.000 mmol L−1) of PA for 24 h. n = 3 per group. (C) Cell viability and glucose uptake after treatment of IR HepG2 cells with different concentrations (0.50, 1.00, 2.00 and 4.00 mg mL−1) of CWP. n = 3 per group. (D) Cell viability and glucose uptake after treatment of IR HepG2 cells with different CWPs (CWP1, CWP2, CWP3, CWP4, CWP5, CWP6, CWP7 and CWP8) at concentrations of 1.00 mg mL−1 for 12 h. n = 3 per group. # indicates a significant difference between the control HepG2 cells and the IR HepG2 cells; #P < 0.05, ##P < 0.01.* indicates a significant difference between the IR HepG2 cells and the CWP HepG2 cells; *P < 0.05, **P < 0.01. (E) Oil Red O staining shows the degrees of lipid accumulation in the IR HepG2 cells treated with different CWPs (CWP, CWP5, CWP6, CWP7 and CWP8) at concentrations of 1.00 mg mL−1 for 12 h. n = 3 per group. Scale bar, 100 μm. (F) PAS staining shows the degrees of glycogen synthesis in the IR HepG2 cells treated with different CWPs (CWP, CWP5, CWP6, CWP7 and CWP8) at concentrations of 1.00 mg mL−1 for 12 h. n = 3 per group. Scale bar, 100 μm. | |
3.6 Effects of different concentrations of CWP on IR HepG2 cells
Different concentrations of CWP (i.e., 0.50, 1.00, 2.00, and 4.00 mg mL−1) were utilized to intervene in the IR HepG2 cells, and the cell viability and glucose consumption were analyzed. The viabilities of HepG2 cells after the treatment with different concentrations of CWP are shown in Fig. 3C. When the concentration was greater than 2 mg mL−1, the CWP treatment induced significant growth inhibition on HepG2 cells. These results suggest that CWP at the concentration of 0.5–2 mg mL−1 was safe for HepG2 cells. The glucose uptake in the IR group was significantly reduced in comparison with the control group (P < 0.01) (Fig. 3C). Different concentrations of CWP (i.e., 0.5 mg mL−1, 1.00 mg mL−1 and 2.00 mg mL−1) increased the glucose uptake levels of IR HepG2 cells by 59.5%, 68.4%, and 67.6%, respectively. Our results show that camel whey protein has hypoglycemic activity at a concentration of 0.5–2 mg mL−1, which does not influence the cellular proliferation of cells.
3.7 Effects of different CWPs on IR HepG2 cells
To further determine the active ingredient in camel whey protein, it was graded and precipitated using ammonium sulfate to obtain different camel whey proteins, i.e. CWP1-CWP8. The IR HepG2 cells were treated with CWP1–CWP8 at the concentration of 1.00 mg mL−1, and the functional components of hypoglycemic activity were screened by detecting HepG2 cell viability and glucose uptake. After the treatment of CWPs, there were no significant differences in HepG2 cell viability in comparison with the control group (P > 0.05) (Fig. 3D). After the treatment of CWP1, CWP2, CWP3, CWP4, and CWP5, there were no significant differences in the glucose uptake levels in comparison with the IR group (P > 0.05). After treatment with CWP6, CWP7 and CWP8, compared with the IR group, the glucose uptake levels increased by 18.5%, 25.0% and 42.9%, respectively (P < 0.01). These results suggested that CWP6, CWP7, and CWP8 can significantly improve glucose uptake and improve the IR of HepG2 cells.
3.8 Effect of CWP on lipid accumulation and glycogen synthesis in IR HepG2 cells
As shown in Fig. 3E, compared with the normal HepG2 cells, the lipid droplets significantly accumulated in the IR HepG2 cells. After the treatment of CWP, CWP6, CWP7, and CWP8, the intracellular lipid droplets were significantly reduced. As shown in Fig. 3F, compared with the normal HepG2 cells, the glycogen synthesis in the IR HepG2 cells was significantly reduced. After the treatment with CWP, CWP6, CWP7 and CWP8, the glycogen synthesis in the HepG2 cells significantly increased. These results suggested that CWP8 can significantly reduce lipid accumulation and promote glycogen synthesis in IR HepG2 cells.
3.9 Effects of CWP8 on the PI3K/Akt signaling pathway in IR HepG2 cells
In order to further explore the possible molecular mechanism of the effects of CWP8 on IR HepG2 cells, the effects of CWP8 on the mRNA and protein expression levels of the PI3K/Akt pathway were investigated. As shown in Fig. 4A, compared with the control group, the PA induction significantly down-regulated the mRNA expression levels of PI3K, Akt, and GluT4, while up-regulated the mRNA expression levels of GSK-3β, G6Pase, and SREBP1c. After the CWP8 treatment, the mRNA expression levels of PI3K, Akt and GluT4 significantly increased, and the mRNA expression levels of GSK-3β, G6Pase, and SREBP1c decreased (P < 0.01). As shown in Fig. 4B, compared with the normal HepG2 cells, the PA treatment significantly decreased the protein expression levels of IRS2, PI3Kp85, p-PI3Kp85, PDK1, Akt, and p-Akt, and increased the protein expression level of GSK-3β (P < 0.05). After the CWP8 treatment, the protein expression levels of IRS2, PI3Kp85, p-PI3Kp85, PDK1, Akt, and p-Akt significantly increased, and the protein expression level of GSK-3β was reduced (P < 0.05). These results indicated that CWP8 might activate the PI3K/Akt signaling pathway in HepG2 cells to exert its hypoglycemic effects in PA-treated HepG2 cells.
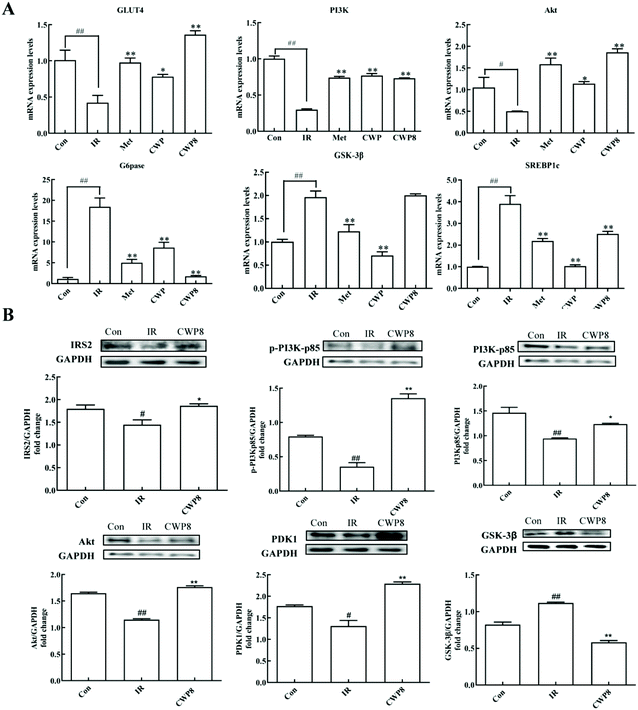 |
| Fig. 4 Camel whey protein ameliorates lipid accumulation and glycogen synthesis in PA-induced IR HepG2 cells via activation of the PI3K/Akt signaling pathway. (A) qPCR analysis of the transcript levels of genes related to the PI3K/Akt signaling pathway (GLUT4, PI3K, Akt, FOXO1, G6Pase, and SREBP1c). Gene expression was normalized to Actb mRNA levels. n = 6 per group. (B) Western blotting of proteins involved in the PI3K/Akt signaling pathway (IRS2, PI3K, p-PI3K, Akt, PDK1 and GSK-3β). GAPDH served as a loading control. The data are presented as the mean ± SD. # indicates a significant difference between the control group and the DM group; #P < 0.05, ##P < 0.01. * indicates a significant difference between the control group and the CWP group; *P < 0.05, **P < 0.01. | |
3.10 The LC-MS/MS identification of CWP8
Based on the results from SDS-PAGE, CWP8 was mainly composed of proteins with molecular weights of 60–90 kDa and 5–22 kDa (Fig. 5A). According to the results from LC-MS/MS, CWP8 was composed of 117 kinds of proteins, including 91 kinds of known proteins and 26 unidentified proteins. There were 14 proteins with molecular weights of 60–90 kDa (table 3), including lactoferrin, serum albumin, serpin A3-8, heat shock cognate protein, glucose-regulated protein, and so on. There were 40 proteins with molecular weights of 5–22 kDa (table 4), including glycosylation-dependent cell adhesion molecule 1 (GlyCAM-1), copper transport protein (ATOX1), protein/nucleic acid deglycase (DJ-1), thioredoxin (TRX), zinc-α2-glycoprotein (ZAG), superoxide dismutase (Cu–Zn), phosphatidylethanolamine-binding protein 1 (PEBP1), lactoferrin (LF), and peptidoglycan recognition protein 1 (PGRP-1).
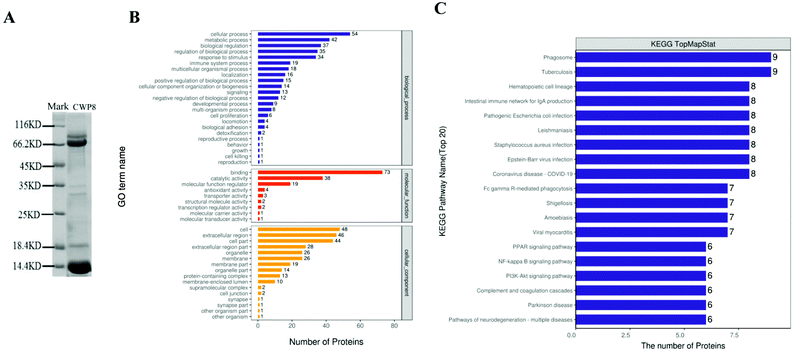 |
| Fig. 5 LC-MS/MS identification, GO analysis, and pathway analysis of identified proteins of camel whey protein (CWP8). (A) SDS-PAGE pattern of CWP8. (B) GO analysis of identified proteins of CWP8. (C) The top twenty KEGG pathways of identified proteins of CWP8. | |
Table 3 Proteins with a molecular weight of 60–90 kDa in CWP8
Numerical order |
Protein description (fasta headers) |
Number of proteins |
Peptides |
Unique peptides |
Sequence coverage [%] |
Mol. weight [kDa] |
1 |
Serum albumin |
4 |
32 |
29 |
59.5 |
69.293 |
2 |
Sulfhydryl oxidase |
2 |
19 |
14 |
46 |
62.974 |
3 |
Lactoferrin |
15 |
31 |
16 |
53.5 |
76.274 |
4 |
Vitamin K-dependent protein S |
2 |
3 |
3 |
5.5 |
75.132 |
5 |
Tripeptidyl-peptidase 1 |
2 |
4 |
4 |
8.5 |
61.351 |
6 |
Polymeric immunoglobulin receptor |
2 |
19 |
2 |
43.1 |
82.521 |
7 |
Heat shock cognate protein |
8 |
18 |
16 |
34.8 |
71.24 |
8 |
Lactoperoxidase |
2 |
15 |
11 |
25.8 |
80.641 |
9 |
Glucose-regulated protein |
4 |
4 |
3 |
8.3 |
72.209 |
10 |
Moesin |
1 |
13 |
2 |
22 |
67.974 |
11 |
Stress-induced-phosphoprotein 1 |
3 |
2 |
2 |
3.3 |
62.481 |
12 |
Lactoperoxidase isoform 1 preproprotein |
1 |
15 |
2 |
25.1 |
87.868 |
13 |
Serpin A3-8 |
1 |
8 |
7 |
17.7 |
75.054 |
14 |
Complement factor B |
1 |
14 |
10 |
26.7 |
85.343 |
Table 4 Proteins with a molecular weight of 5–22 kDa in CWP8
Numerical order |
Protein description (fasta headers) |
Number of proteins |
Peptides |
Unique peptides |
Sequence coverage [%] |
Mol. weight [kDa] |
1 |
Casein |
2 |
4 |
1 |
44.7 |
15.772 |
2 |
Cytokeratin 18 |
4 |
1 |
1 |
11 |
9.192 |
3 |
Nucleoside diphosphate kinase |
1 |
1 |
1 |
7.1 |
19.079 |
4 |
Motif chemokine |
1 |
1 |
1 |
11.6 |
14.556 |
5 |
Cofilin 1 |
5 |
3 |
3 |
25.9 |
18.518 |
6 |
Lactoglobulin |
1 |
10 |
1 |
65.2 |
19.969 |
7 |
Alpha lactalbumin |
5 |
5 |
5 |
39.8 |
14.156 |
8 |
Thymosin beta 4 |
5 |
1 |
1 |
25 |
5.0967 |
9 |
Profilin-1 |
5 |
4 |
4 |
33.6 |
15.057 |
10 |
Cytochrome c |
5 |
1 |
1 |
10.5 |
11.647 |
11 |
Metallothionein |
11 |
1 |
1 |
19.7 |
5.9811 |
12 |
Ubiquitin |
9 |
4 |
4 |
56.6 |
8.5647 |
14 |
Phosphatidylethanolamine-binding protein 1 |
1 |
6 |
4 |
48.1 |
20.985 |
15 |
Protein/nucleic acid deglycase DJ-1 |
2 |
6 |
6 |
41.3 |
20.035 |
17 |
Peptidoglycan recognition protein 1 |
2 |
7 |
7 |
66.8 |
21.377 |
18 |
Phosphatidylethanolamine-binding protein |
1 |
5 |
3 |
38.9 |
21.109 |
19 |
Zinc-alpha-2-glycoprotein-like protein |
1 |
8 |
6 |
51.3 |
21.903 |
22 |
Putative 42-9-9 protein |
2 |
2 |
2 |
22.1 |
11.774 |
23 |
Small ubiquitin-related modifier 2 |
3 |
1 |
1 |
10.5 |
10.871 |
24 |
Domain-binding glutamic acid-rich-like protein |
3 |
4 |
4 |
40 |
11.339 |
25 |
Thioredoxin |
3 |
2 |
2 |
22.7 |
10.868 |
27 |
Superoxide dismutase [Cu–Zn] |
2 |
4 |
4 |
41.2 |
15.742 |
28 |
Whey acidic protein |
3 |
6 |
6 |
76.1 |
12.564 |
29 |
Fatty acid-binding protein, heart |
5 |
9 |
1 |
65.4 |
14.779 |
30 |
Glycosylation-dependent cell adhesion molecule 1 |
1 |
8 |
8 |
49.7 |
17.291 |
31 |
Tubulin-specific chaperone A |
1 |
1 |
1 |
9.3 |
12.707 |
32 |
Myotrophin-like protein |
2 |
1 |
1 |
16.1 |
12.895 |
33 |
Nucleoside diphosphate kinase B |
2 |
6 |
2 |
44.7 |
17.316 |
34 |
Acyl-CoA-binding protein |
1 |
2 |
2 |
29.6 |
13.008 |
35 |
Beta-2-microglobulin |
1 |
4 |
4 |
43.2 |
13.655 |
36 |
Nucleoside diphosphate kinase |
1 |
4 |
1 |
40.5 |
14.197 |
37 |
Ig lambda chain C regions isoform 19-like protein |
1 |
6 |
5 |
58.3 |
14.906 |
38 |
Transthyretin |
1 |
5 |
4 |
49.3 |
16.085 |
39 |
Cystatin |
1 |
1 |
1 |
15.8 |
12.027 |
40 |
Copper transport protein ATOX1 |
2 |
3 |
3 |
64.7 |
7.3514 |
3.11 Gene ontology (GO) annotation and KEGG pathway annotation of 91 identified proteins in CWP8
All 91 identified proteins in CWP8 were classified using the gene ontology (GO) annotation and the results are presented in Fig. 5B. They were classified into biological processes (BP), cellular components (CC), and molecular functions (MF). Frequently encountered biological processes included the metabolic processes, biological regulation, biological process regulation, and immune system processes. The most common molecular function was the binding activity, and another functional category was the catalytic activity and molecular function regulators. The prevalent cellular components were placed in the intracellular part and extracellular region. The identified proteins in CWP8 in the top 20 pathways of the KEGG annotation are shown in Fig. 5C. Many signaling pathways closely correlated with DM development were involved, such as the PI3K-Akt signaling pathway and the PPAR signaling pathway.
4. Discussion
In the present study, the hypoglycemic and liver-protective effects of CWP on HFD/STZ-induced T2DM were investigated. Our results demonstrated that CWP exhibits a potential anti-hyperglycemic effect and exhibits significant protective effects on the liver of T2DM rats, in agreement with previous findings indicating that CWP is beneficial for diabetics. To investigate the antidiabetic effect of CWP in vivo, a HFD and STZ-induced T2DM rat model was established herein. Our results indicated that intragastric administration of CWP to T2DM rats resulted in significant inhibition of body weight loss, as well as the increase of FBG and FINS levels. Abnormal metabolism of glucose and lipids represented the principal features of T2DM. The TC, TG, and HDL-C levels of DM patients (T1DM and T2DM) may have normalized after the treatment with camel milk, as described previously.20,21 In our experiments, our results showed that CWP can improve dyslipidemia in T2DM rats, in line with the previous findings.
Current research studies on diabetes have shown that liver damage is a serious complication of diabetes.22 When the liver is damaged in diabetic patients, the antioxidant enzyme activity of hepatocytes decreases, and a large number of oxygen free radicals are produced, leading to oxidative stress and lipid peroxidation in the liver.23 Diabetes and its complications can cause pathological changes in liver tissue, leading to damage to liver function.24 In this study, the levels of MDA in the liver of diabetic rats significantly increased, and the levels of SOD and GPx decreased, with significantly changed histopathological features. However, the CWP treatment could significantly improve the tissue pathologically, reduce the MDA levels, and restore the SOD and GPx activities (to near-normal levels). Diabetes is generally considered to be a pro-inflammatory state. Oxidative stress would increase and stimulate the release of inflammatory cytokines, while the release of cellular inflammatory factors would induce systemic inflammation, accelerating diabetes development and the occurrence of diabetic complications.25 It is worth noting that the CWP treatment significantly decreased the serum levels of inflammatory cytokines (such as IL-6 and TNF-α) in the T2MD rats. The enhanced activity of SOD and GPx may help CWP scavenge the oxygen free radicals to reduce the oxidative stress, thereby reducing the release of inflammatory factors to improve liver cell damage. Therefore, the CWP treatment can protect the liver of T2DM rats by reducing oxidative stress in the liver and inflammation.
In the process of insulin signal transduction, insulin mainly regulates glucose and lipid metabolism in the body via the PI3K/Akt signaling pathway. Insulin stimulates autophosphorylation of the insulin receptor, thereby phosphorylating and activating IRS, which subsequently activates PI3K, as well as the downstream PDK1 and Akt. The activated Akt would be released from the plasma membrane, transferred to the nucleus, cytoplasm, and mitochondria, and act on glycogen synthase kinase 3β (GSK3β). The transcription factor substrates (such as forkhead boxO1 (FoxO1)) participate in the insulin-mediated regulation of glucose metabolism through a variety of ways. Phosphorylated Akt can promote the phosphorylation and inactivation of GSK3β. The GS activity would be increased by inhibiting the phosphorylation of glycogen synthase, promoting the insulin-stimulated liver glycogen synthesis. Phosphorylated Akt can also inhibit the transport of FoxO1 to the nucleus, thereby reducing the level of phosphorylation, decreasing the gluconeogenesis protein pyruvate carboxylase (PC), phosphoenolpyruvate carboxykinase (PEPCK), and glucose-6-phosphatase (G6Pase) expressions, in turn inhibiting liver gluconeogenesis and reducing the liver glucose output.26,27 In this study, our results showed that CWP increased the expression levels of IRS2, PI3K, Akt, GSK3, and GS in T2DM rats, indicating that the PI3K/Akt pathway was activated. The activated PI3K would improve insulin sensitivity through feedback from IRS-2. The activated GSK3, GS, FoxO1, and G6Pase were able to regulate the glucose metabolism. We speculated that CWP could increase the serum insulin concentration and activate the insulin/PI3K/Akt signaling pathway in the liver, thereby promoting glycogen synthesis and inhibiting gluconeogenesis to lower the blood glucose levels.
Camel whey protein (CWP) contains immunoglobulin (Ig), serum albumin, α-lactalbumin (α-LA), and peptidoglycan recognition proteins. CWP also contains lactoferrin (LF), lactoperoxidase (LPO), and lysozyme as well as other proteins with biological functions.5 To further confirm the active protein component in CWP, a PA-induced IR HepG2 cell model was used to filter and evaluate the efficacy of CWP, and was used for the identification of specific active protein components. IR is one of the key factors in the pathogenesis of T2DM. The PA treatment can induce cells to produce IR.28 The PA treatment can inhibit the PI3K/Akt signaling pathway, which in turn triggers inflammation and lipotoxicity, leading to insulin resistance in cells.29 The PA-induced IR cell model has been extensively used in vitro. In this study, 0.01 mM PA was used to induce the IR HepG2 cell model. Our results from the MTT assay showed that 0.5–2 mg ml−1 CWP was safe for the IR HepG2 cells. Noticeably, CWP8 was the most outstanding compound when compared to other CWPs. CWP8 significantly enhanced the glucose uptake by upregulating the expression of GLU4 in PA-induced IR HepG2 cells. Moreover, the CWP8 administration significantly decreased lipid deposition by inhibiting the expression levels of FoxO1 and SREBP1c in PA-induced HepG2 cells, as well as promoted glycogen synthesis by up-regulating the phosphorylation of GSK3β in IR HepG2 cells.
Totally, 91 proteins were identified in CWP8, according to the results of the gene ontology (GO) annotation and KEGG pathway annotation. Our results showed that the proteins in CWP8 components were widely involved in the regulation of cell glucose and lipid metabolism, oxidative stress, signal transmission, etc. Therefore, the proteins in CWP8 were further analyzed.
The pathogenesis of diabetic complications has been confirmed to be associated with the body's oxidative damage caused by excessive free radicals. Superoxide dismutase (Cu–Zn), copper transport protein (ATOX1), protein/nucleic acid deglycase (DJ-1), and thioredoxin (TRX) exhibit antioxidant activities and play crucial roles in scavenging oxygen free radicals and inhibiting oxidative damage. Cu–Zn (as SOD) is an antioxidant metal enzyme that exists in organisms, which is a metalloenzyme that can be distributed in the tissues and can effectively eliminate free radicals in the body.30 SOD can catalyze the disproportionation of superoxide anion radicals to generate oxygen and hydrogen peroxide, which play a crucial role in keeping the balance of oxidation and antioxidation in the body. ATOX1 is an antioxidant against hydrogen peroxide and superoxide and can significantly inhibit the production of cellular reactive oxygen species, DNA damage, and activation of MAPKs, which has significant protective effects on STZ-induced apoptosis in vivo and in vitro.31 DJ-1, an antioxidant that scavenges reactive oxygen species, protects cells from undergoing oxidative stress-induced apoptosis. It has been shown that DJ-1 is widely involved in the pathogenesis of oxidative stress-related diseases, such as cancer, neurodegenerative diseases, and T2DM. DJ-1 would inhibit excessive apoptosis of pancreatic islet cells via anti-oxidation and regulation of apoptosis, promoting the release of insulin and maintaining blood glucose homeostasis, and ultimately participating in the occurrence and development of diabetes.32,33 Studies have shown that siRNA to silence the expression of DJ-1 in pancreatic β cells would accelerate the death of pancreatic islet cells; however, the increase of DJ-1 expression by adenovirus vectors could alleviate cell death.34 Thioredoxin (TRX) is a redox-active protein that regulates reactive oxidative metabolism and plays a crucial role in regulating the reduction/oxidation balance by scavenging the reactive oxygen species.35 TRX plays a vital role in regulating various protein functions, such as enzyme activity, cell growth, proliferation and redox regulation, thus inhibiting inflammatory factors and cell apoptosis.36
Lipotoxicity refers to the excessive deposition of free fatty acid (FFA) in non-adipose tissue in the form of triglyceride. The increased level of FFA in the blood exceeds the storage and oxidation capacities of the adipose tissue, resulting in tissue damage. Lipid toxicity is mainly related to dysregulation of functional signals and insulin resistance in non-fat tissues (such as myocardium, pancreas, skeletal muscle, liver, and kidneys).36 Zinc-α2-glycoprotein (ZAG) and phosphatidylethanolamine-binding protein 1 (PEBP1) play important roles in lipid metabolism. ZAG is an important histocompatibility complex I molecule and a lipid-mobilizing factor. Expanding evidence has shown that ZAG can enhance lipid metabolism and glucose utilization, and regulate insulin sensitivity.37 Studies have shown that ZAG can promote fatty acid oxidation and lipid degradation, and regulate lipid metabolism, and affect the expression levels of other adipokines via autocrine or paracrine pathways (such as promoting adiponectin and inhibiting the expression of leptin in adipose tissue).38 Besides, experiments such as those in AZG-deficient mice demonstrate that AZG can control body weight and lipolysis. It has been shown that serum ZAG levels decreased in the subjects of obesity, impaired glucose tolerance, and T2DM groups. ZAG may have an important relationship with glucose and lipid metabolism disorders and obesity.39 PEBP1 has also been known as RAF1 kinase inhibitory protein (RKIP1). Studies have shown that PEBP1 not only participates in various cellular processes (such as proliferation, differentiation, survival, and movement), but also is related to a variety of diseases (including cancer, Alzheimer's disease, diabetes, and retinal diseases).40 In the fenofibrate-fed T2DM rats, PEBP1 is significantly up-regulated in the insulin-targeted tissues (liver, muscle, and fat), suggesting that PEBP1 can improve insulin sensitivity and control lipid metabolism.41
Glycosylation-dependent cell adhesion molecule 1 (GlyCAM-1), lactoferrin (LF), and peptidoglycan recognition protein 1 (PGRP-1) are antibacterial proteins in milk-related to innate immunity. GlyCAM-1, also known as lactokeratin, acts as an endothelial cell surface ligand for the lymphocyte homing receptor L-selectin, which indirectly participates in the immune response.42 LF, as a natural antibacterial peptide in milk, is a non-heme iron-binding glycoprotein with immune function. LF can inhibit the generation of free radicals, and exhibits broad-spectrum antibacterial and antiviral activities.43 Besides, LF also has anti-cancer effects, inhibits cholesterol production, promotes cell growth, improves anemia, and promotes wound healing activities. Studies concerning the hypoglycemic mechanism of LF have shown that LF can increase the phosphorylation of Akt protein and reduce lipid production in the HepG2 cells.44 Studies have found that camel milk-derived LF can significantly improve insulin sensitivity in T2DM patients, exerting anti-inflammatory and immune-regulating effects.8 PGRP-1 is a highly conserved family of pattern recognition receptors (PRRs) that can recognize peptidoglycan, activating and regulating the body's innate immunity. PGRP-1 has been shown to be able to combine with peptidoglycan on the cell wall of pathogens to inhibit their growth.42
Heat shock proteins (HSPs) are conserved molecules promoting the correct folding of other proteins. Most HSPs are stress-induced and have cytoprotective effects in cells exposed to stress. As a kind of cellular stress protein, the expression levels of HSPs would be rapidly increased under physiological or pathological conditions, reducing the body damage. Besides, HSPs are related to a variety of immunomodulatory functions.45 The HSP70 family (including GRP75, GRP78, HSP72 and HSP73) is one of the most extensively researched in the HSP family. The HSP70 family exhibits versatile biological activities (such as anti-stress, anti-oxidation, and regulation of cell apoptosis), which are also closely related to the occurrence and development of diabetes. HSP72 can block the c-Jun N-terminal kinase signal of skeletal muscle, enhance cell oxidative metabolism, decrease fat accumulation, and prevent insulin resistance.46
Previous studies have shown that the functions of these proteins in CWP8 are closely related to glucose and lipid metabolism, as well as the insulin signaling pathways, which needs further functional and clinical investigation. Moreover, the role of a certain protein component of CWP/CWP8 or CWP8 in the treatment of diabetes needs to be confirmed based on a larger subject size. Based on the evidence available right now, it is recommended that diabetic patients add CWP/CWP8 to their daily diet as a nutritional supplement and an adjuvant, as part of anti-diabetic management strategies.
5. Conclusion
In summary, our results showed that intragastric administration with CWP reduced body weight loss and decreased the increase of FBG and FINS in T2DM rats. Moreover, CWP treatment reduced serum levels of inflammatory cytokines and improved dyslipidemia. Besides, CWP protected the T2DM rats against liver injury via the mitigation of oxidative stress. An active protein component CWP8 was isolated herein, and our results indicated that CWP8 enhanced glycogen synthesis and ameliorated lipid accumulation in PA-induced IR HepG2 cells. Moreover, the hypoglycemic effect of CWP and CWP8 could regulate glucose metabolism by activating the PI3K/Akt pathway, and could inhibit gluconeogenesis and promote glycogen synthesis.
Author contributions
Zhihua Dou: conceptualization, investigation, formal analysis, and writing – original draft. Xinhuan Feng and Yutong Xie: data curation and investigation. Chen Liu and Zhongkai Zhao: software and writing – review and editing. Jing Dong: methodology and resources. Haitao Yue: project administration, supervision and funding. Jie Yang: conceptualization, funding acquisition, supervision, and writing – review and editing. All authors approved the final manuscript and agreed to be accountable for all the aspects of the work.
Conflicts of interest
The authors declare no conflicts of interest.
Acknowledgements
This study was funded by the projects of the National Natural Science Foundation of China (81860339) and the program of the Key Research and Development Projects in Xinjiang Province (2018B01003).
References
- A. I. Aqib, M. Fakhar-e-Alam Kulyar, K. Ashfaq, Z. A. Bhutta, M. Shoaib and R. Ahmed, Camel milk insuline: Pathophysiological and molecular repository, Trends Food Sci. Technol., 2019, 88, 497–504 CrossRef CAS.
- N. H. Cho, J. E. Shaw, S. Karuranga, Y. Huang, J. D. da Rocha Fernandes, A. W. Ohlrogge and B. Malanda, IDF Diabetes Atlas: Global estimates of diabetes prevalence for 2017 and projections for 2045, Diabetes Res. Clin. Pract., 2018, 138, 271–281 CrossRef CAS PubMed.
- M. E. Hamad, A. E. Abdel-Rahim and A. E. Romeih, Beneficial Effect of Camel Milk on Liver and Kidneys Function in Diabetic Sprague-Dawley Rats, Int. J. Dairy Sci., 2011, 6(3), 190–197 CrossRef.
- V. T. Samuel and G. I. Shulman, Mechanisms for insulin resistance: common threads and missing links, Cell, 2012, 148, 852–871 CrossRef CAS PubMed.
- G. Badr, N. Ramadan, L. Sayed, B. Badr, H. Omar and Z. Selamoglu, Why whey? Camel whey protein as a new dietary approach to the management of free radicals and for the treatment of different health disorders, Iran. J. Basic Med. Sci., 2017, 20, 338–349 Search PubMed.
- A. H. Fahaid, Camel’s Milk Protects Against Aluminum Chloride-Induced Normocytic Normocromic Anemia, Lipid Peroxidation and Oxidative Stress in Erythrocytes of White Albino Rats, Am. J. Biochem. Biotechnol., 2009, 5(3), 126–136 CrossRef.
- B. P. Kilari, P. Mudgil, S. Azimullah, N. Bansal, S. Ojha and S. Maqsood, Effect of camel milk protein hydrolysates against hyperglycemia, hyperlipidemia, and associated oxidative stress in streptozotocin (STZ)-induced diabetic rats, J. Dairy Sci., 2021, 104, 1304–1317 CrossRef CAS PubMed.
- A. A. Korish and M. M. Arafah, Camel milk ameliorates steatohepatitis, insulin resistance and lipid peroxidation in experimental non-alcoholic fatty liver disease, BMC Complementary Altern. Med., 2013, 13, 1–12 CrossRef PubMed.
- H. S. Ejtahed, A. N. Naslaji, P. Mirmiran, M. Z. Zraif Yeganeh, M. Hedayati, F. Azizi and A. M. Moosavi Movahedi, Effect of camel milk on blood sugar and lipid profile of patients with type 2 diabetes: a pilot clinical trial, Int. J. Endocrinol. Metab., 2015, 13(1), 21160–21166 Search PubMed.
- A. A. Korish, The antidiabetic action of camel milk in experimental type 2 diabetes mellitus: an overview on the changes in incretin hormones, insulin resistance, and inflammatory cytokines, Horm. Metab. Res., 2014, 46, 404–411 CrossRef CAS PubMed.
- H. B. Lindner, A. Zhang, J. Eldridge, M. Demcheva, P. Tsichlis, P. Tsichilis, A. Seth, J. Vournakis and R. C. Muisehelmericks, Camel whey protein enhances diabetic wound healing in a streptozotocin-induced diabetic mouse model: the critical role of β-Defensin-1, -2 and -3, Lipids Health Dis., 2013, 12(1), 46–57 CrossRef PubMed.
- J. Ajarem, A. A. Allam, H. Ebaid, S. N. Maodaa, S. M. Al-Sobeai, A. M. Rady, A. Metwalli, N. G. Altoom, K. Ibrahim and M. I. Sabri, Neurochemical, structural and neurobehavioral evidence of neuronal protection by whey proteins in diabetic albino mice, Behav. Brain Funct., 2015, 11, 1–8 CrossRef PubMed.
- A. Ashraf, P. Mudgil, A. Palakkott, R. Iratni, C. Y. Gan, S. Maqsood and M. A. Ayoub, Molecular basis of the anti-diabetic properties of camel milk through profiling of its bioactive peptides on dipeptidyl peptidase IV (DPP-IV) and insulin receptor activity, J. Dairy Sci., 2021, 104, 61–77 CrossRef CAS PubMed.
- C. Liu, Z. H. Dou, S. J. Sun, M. Liu and Z. M. Hou, Protective Effect of Xinjiang Bactrian Camel Milk on the Liver of Streptozotocin-Induced Diabetic Mice, Food Sci., 2020, 41, 83–89 Search PubMed.
- Z. M. Hou, L. Min, D. Xue and W. Guiling, Protective Effect of Whey Protein from Xinjiang Bactrian Camel on Palmitic Acid Injury of MIN6 Cells, China Dairy Ind., 2021, 49, 6 Search PubMed.
- J. Peng, J. K. Zheng and X. M. Peng, Study on the Improving Effect of Quercetin
on Glucose Metabolism in Insulin Resistant HepG2 Cells, Pharm. Today, 2017, 27, 436–440 CAS.
- H. H. Arab, S. A. Salama and I. A. Maghrabi, Camel Milk Ameliorates 5-Fluorouracil-Induced Renal Injury in Rats: Targeting MAPKs, NF-κB and PI3K/Akt/eNOS Pathways, Cell. Physiol. Biochem., 2018, 46, 1628–1642 CrossRef CAS PubMed.
- M. Halim and A. Halim, The effects of inflammation, aging and oxidative stress on the pathogenesis of diabetes mellitus (type 2 diabetes), Diabetes Metab. Syndr., 2019, 13, 1165–1172 CrossRef PubMed.
- G. Wu, Z. Bai, Y. Wan, H. Shi, X. Huang and S. Nie, Antidiabetic effects of polysaccharide from azuki bean (Vigna angularis) in type 2 diabetic rats via insulin/PI3K/AKT signaling pathway, Food Hydrocolloids, 2020, 101, 10456–10464 Search PubMed.
- H. S. Ejtahed, A. N. Naslaji, P. Mirmiran, M. Z. Yeganeh, M. Hedayati, F. Azizi and A. M. Movahedi, Effect of Camel Milk on Blood Sugar and Lipid Profile of Patients With Type 2 Diabetes: A Pilot Clinical Trial, Int. J. Endocrinol. Metab., 2015, 13(1), 21160–21166 Search PubMed.
- R. H. Mohamad, Z. K. Zekry, H. A. Al-Mehdar, O. Salama, S. E. El-Shaieb, A. A. El-Basmy, M. G. Al-said and S. M. Sharawy, Camel milk as an adjuvant therapy for the treatment of type 1 diabetes: verification of a traditional ethnomedical practice, J. Med. Food, 2009, 12, 461–465 CrossRef CAS PubMed.
- C. Komiya, K. Tsuchiya, K. Shiba, Y. Miyachi, S. Furuke, N. Shimazu, S. Yamaguchi, K. Kanno and Y. Ogawa, Ipragliflozin Improves Hepatic Steatosis in Obese Mice and Liver Dysfunction in Type 2 Diabetic Patients Irrespective of Body Weight Reduction, PLoS One, 2016, 11(3), 1–19 CrossRef PubMed.
- A. Besse-Patin and J. L. Estall, An Intimate Relationship between ROS and Insulin Signalling: Implications for Antioxidant Treatment of Fatty Liver Disease, Int. J. Cell Biol., 2014, 2014, 519153 CrossRef PubMed.
- A. Tang, R. Rabasa-Lhoret, H. Castel, C. Wartelle-Bladou, G. Gilbert, K. Massicotte-Tisluck, G. Chartrand, D. Olivié, A. S. Julien, J. de Guise, G. Soulez and J. L. Chiasson, Effects of Insulin Glargine and Liraglutide Therapy on Liver Fat as Measured by Magnetic Resonance in Patients With Type 2 Diabetes: A Randomized Trial, Diabetes Care, 2015, 38, 1339–1346 CrossRef CAS PubMed.
- M. A. Ayoub, A. R. Palakkott, A. Ashraf and R. Iratni, The molecular basis of the anti-diabetic properties of camel milk, Diabetes Res. Clin. Pract., 2018, 146, 305–312 CrossRef CAS PubMed.
- K. Wang, Z. Tang, Z. Zheng, P. Cao, W. Shui, Q. Li and Y. Zhang, Protective effects of Angelica sinensis polysaccharide against hyperglycemia and liver injury in multiple low-dose streptozotocin-induced type 2 diabetic BALB/c mice, Food Funct., 2016, 7, 4889–4897 RSC.
- X. Huang, G. Liu, J. Guo and Z. Su, The PI3K/AKT pathway in obesity and type 2 diabetes, Int. J. Biol. Sci., 2018, 14, 1483–1496 CrossRef CAS PubMed.
- J. Li, X. Ding, T. Jian, H. Lü, L. Zhao, J. Li, Y. Liu, B. Ren and J. Chen, Four sesquiterpene glycosides from loquat (Eriobotrya japonica) leaf ameliorates palmitic acid-induced insulin resistance and lipid accumulation in HepG2 Cells via AMPK signaling pathway, PeerJ, 2020, 8, 10413–10430 CrossRef PubMed.
- M. Morgan, S. D. Boyd, D. D. Winkler and D. R. Winge, Oxygen-dependent activation of Cu,Zn-superoxide dismutase-1, Metallomics, 2017, 9, 1047–1059 CrossRef PubMed.
- E. H. Ahn, D. W. Kim, M. J. Shin, E. J. Ryu, J. I. Yong, S. Y. Chung, H. J. Cha, S. J. Kim, Y. J. Choi, D. S. Kim, S. W. Cho, K. Lee, Y. S. Cho, H. Y. Kwon, J. Park, W. S. Eum and S. Y. Choi, Tat-ATOX1 inhibits streptozotocin-induced cell death in pancreatic RINm5F cells and attenuates diabetes in a mouse model, Int. J. Mol. Med., 2016, 38, 217–224 CrossRef CAS PubMed.
- L. Zhang, J. Wang, J. Wang, B. Yang, Q. He and Q. Weng, Role of DJ-1 in Immune and Inflammatory Diseases, Front. Immunol., 2020, 11, 994 CrossRef CAS PubMed.
- Q. Zhou, H. L. Wei, H. C. Deng and D. O. Endocrinology, Research progress on the relationship between DJ-1 protein and diabetes, Med. J. Chin. People’s Liberation Army, 2018, 530–533 Search PubMed.
- A. Inberg and M. Linial, Protection of pancreatic beta-cells from various stress conditions is mediated by DJ-1, J. Biol. Chem., 2010, 285, 25686–25698 CrossRef CAS PubMed.
- W. Ito, N. Kobayashi, M. Takeda, S. Ueki, H. Kayaba, H. Nakamura, J. Yodoi and J. Chihara, Thioredoxin in allergic inflammation, Int. Arch. Allergy Appl. Immunol., 2011, 155(Suppl 1), 142–146 CrossRef CAS PubMed.
- M. Venojärvi, A. Korkmaz, S. Aunola, K. Hällsten, K. Virtanen, J. Marniemi, J. P. Halonen, O. Hänninen, P. Nuutila and M. Atalay, Decreased thioredoxin-1 and increased HSP90 expression in skeletal muscle in subjects with type 2 diabetes or impaired glucose tolerance, BioMed Res. Int., 2014, 2014, 386351 Search PubMed.
- D. Yazici and H. Sezer, Insulin Resistance, Obesity and Lipotoxicity, Adv. Exp. Med. Biol., 2017, 960, 277–304 CrossRef CAS PubMed.
- H. M. Pearsey, J. Henson, J. A. Sargeant, M. J. Davies, K. Khunti, T. Suzuki, K. A. Bowden-Davies, D. J. Cuthbertson and T. E. Yates, Zinc-alpha2-glycoprotein, dysglycaemia and insulin resistance: a systematic review and meta-analysis, Rev. Endocr. Metab. Disord., 2020, 21, 569–575 CrossRef CAS PubMed.
- L. Xu, W. Yu, M. Niu, C. Zheng, B. Qu, Y. Li, J. Wang, P. Huang, O. Wang and F. Gong, Serum ZAG Levels Were Associated with eGFR Mild Decrease in T2DM Patients with Diabetic Nephropathy, Int. J. Endocrinol., 2017, 2017, 5372625 Search PubMed.
- T. Mracek, D. Gao, T. Tzanavari, Y. Bao, X. Xiao, C. Stocker, P. Trayhurn and C. Bing, Downregulation of zinc-{alpha}2-glycoprotein in adipose tissue and liver of obese ob/ob mice and by tumour necrosis factor-alpha in adipocytes, J. Endocrinol., 2010, 204, 165–172 CAS.
- S. E. Wenzel, Y. Y. Tyurina, J. Zhao, C. M. St Croix, H. H. Dar, G. Mao, V. A. Tyurin, T. S. Anthonymuthu, A. A. Kapralov, A. A. Amoscato, K. Mikulska-Ruminska, I. H. Shrivastava, E. M. Kenny, Q. Yang, J. C. Rosenbaum, L. J. Sparvero, D. R. Emlet, X. Wen, Y. Minami, F. Qu, S. C. Watkins, T. R. Holman, A. P. VanDemark, J. A. Kellum, I. Bahar, H. Bayır and V. E. Kagan, PEBP1 Wardens Ferroptosis by Enabling Lipoxygenase Generation of Lipid Death Signals, Cell, 2017, 171, 628–641 CrossRef CAS PubMed.
- J. R. Hahm, J. S. Ahn, H. S. Noh, S. M. Baek, J. H. Ha, T. S. Jung, Y. J. An, D. K. Kim and D. R. Kim, Comparative analysis of fat and muscle proteins in fenofibrate-fed type II diabetic OLETF rats: the fenofibrate-dependent expression of PEBP or C11orf59 protein, BMB Rep., 2010, 43, 337–343 CrossRef CAS PubMed.
- H. E. Hatmi, Z. Jrad, T. Khorchani, J. Jardin, C. Poirson, C. Perrin, C. Cakir-Kiefer and J. M. Girardet, Identification of bioactive peptides derived from caseins, glycosylation-dependent cell adhesion molecule-1 (GlyCAM-1), and peptidoglycan recognition protein-1 (PGRP-1) in fermented camel milk, Int. Dairy J., 2016, 159–168 CrossRef.
- L. Moreno-Expósito, R. Illescas-Montes, L. Melguizo-Rodríguez, C. Ruiz and E. D. Luna-Bertos, Multifunctional capacity and therapeutic potential of lactoferrin, Life Sci., 2018, 195, 61–64 CrossRef PubMed.
- J. M. Moreno-Navarrete, F. J. Ortega, W. Ricart and J. M. Fernandez-Real, Lactoferrin increases (172Thr)AMPK phosphorylation and insulin-induced (p473Ser)AKT while impairing adipocyte differentiation, Int. J. Obes., 2009, 33, 991–1000 CrossRef CAS PubMed.
- T. Zininga, L. Ramatsui and A. Shonhai, Heat Shock Proteins as Immunomodulants, Molecules, 2018, 23(11), 2846–2863 CrossRef PubMed.
- D. C. Henstridge, C. R. Bruce, B. G. Drew, K. Tory, A. Kolonics, E. Estevez, J. Chung, N. Watson, T. Gardner, R. S. Lee-Young, T. Connor, M. J. Watt, K. Carpenter, M. Hargreaves, S. L. McGee, A. L. Hevener and M. A. Febbraio, Activating HSP72 in rodent skeletal muscle increases mitochondrial number and oxidative capacity and decreases insulin resistance, Diabetes, 2014, 63, 1881–1894 CrossRef CAS PubMed.
|
This journal is © The Royal Society of Chemistry 2022 |