DOI:
10.1039/D1FO02619D
(Paper)
Food Funct., 2022,
13, 344-355
UHT treatment and storage of liquid infant formula affects protein digestion and release of bioactive peptides
Received
10th August 2021
, Accepted 6th December 2021
First published on 7th December 2021
Abstract
Ready-to-feed liquid infant formulas (IF) were subjected to direct (D) or indirect (ID) ultra-high-temperature (UHT) treatment and then stored at 40 °C under aseptic conditions for 60–120 days simulating global transportation which accelerates the Maillard reaction. Low pasteurized and unstored IF (LP) was included as a control for the UHT treatments. Simulated infant in vitro digestion was conducted. SDS-PAGE indicated that protein aggregate formation correlated with thermal treatment, being greatest after 60 days of storage. Limited protein digestion was observed after pepsin treatment for 2 h. Beta-lactoglobulin (β-Lg), alpha-lactalbumin (α-La) and protein aggregates remained undigested after 2 h of pepsin digestion in LP and D, but less β-Lg and α-La remained in ID. The digestion of β-Lg and α-La was enhanced in D and ID stored for 60 days, but aggregates remained undigested. After pepsin and pancreatin digestion, large amounts of β-Lg remained undigested in the LP, but digestion increased after UHT treatment (ID > D) and increased further after storage for 60 and 120 days, indicating that heat treatment and storage facilitate the digestion of unaggregated proteins. No aggregates remained after pancreatin digestion of LP, D, ID and D stored for 60 days, but were present in ID stored for 60 days. Aggregates were mainly disulphide-linked, but dityrosine linkages were detected in D and ID stored for 120 days. LC-MS/MS indicated limited proteolysis arising from endogenous milk proteases prior to in vitro digestion, being highest in D. Peptide numbers increased following pepsin and further during pancreatin digestion (β-casein > β-Lg > β-La), and released β-Lg peptides, typically 5–8 amino acids in length, contained several bioactivities, e.g., dipeptidyl-peptidase IV (DPP-IV) and angiotensin converting enzyme (ACE) inhibition.
1. Introduction
Ready-to-feed liquid infant formulas (IF) are designed to mimic human milk, which is rich in many anti-inflammatory proteins, such as immunoglobulins (Ig), lactoferrin (Lf), lactoperoxidase (Lp), milk fat globule membrane proteins (MFGM), osteopontin and lysozyme.1 Not only is IF provided to newborn infants whose mothers cannot breast feed, but also for preterm infants, as the supply of human milk from their own mothers is limited or absent.2 The level of bioactive proteins contained in IF is lower than in human milk and this difference may be related to protein denaturation and aggregate formation induced by thermal processing of IF.1 Such processes involve either spray drying or ultra-high-temperature processing (UHT), in order to provide extended shelf-life outside the cooling chain. Two different types of UHT treatment are commonly applied; direct UHT (D) in which IF is preheated to ∼75 °C, followed by steam injection to reach 135–140 °C for 2–10 s and a subsequent flash cooling. In contrast, indirect UHT (ID) utilises heat exchangers in which preheated IF (∼75 °C) is heated to reach 135 °C at a rate of 1 °C s−1, followed by a holding time of 2–10 s and a subsequent slow cooling at the same rate. Thus, due to the lower heat load, D processing may ensure a higher product quality compared to ID treatment.3 During heating, the free thiol group of β-lactoglobulin (β-Lg, Mw 18 kDa) becomes exposed and binds to caseins (CN, Mw ∼ 25 kDa) forming a disulphide cross-linked complex. α-Lactalbumin (α-La, Mw 14 kDa) then binds to β-Lg by either disulphide cross-linking and/or hydrophobic interactions, forming an aggregate. These protein aggregates are complex and often comprise of other proteins including bovine serum albumin, (BSA, Mw 66 kDa), Lf and Lp (Mw ∼ 80 kDa) forming aggregates with molecular masses of ∼80–260 kDa. In the presence of the high-lactose concentrations added to IF, the Maillard reaction may be initiated due to the reaction of lactose with amino groups of lysine sidechains and protein N-termini, forming a Schiff base (also called lactosylation5). The Schiff base rearranges to form early, advanced and late Maillard reaction products by a complex series of reactions eventually leading to browning, and which may impair protein digestibility.4 Thermal treatment not only promotes the Maillard reaction, but also aggravates aggregation involving both covalent and non-covalent interactions,6,7 and causes denaturation, deamination and oxidation.8 When combined with a long duration of product storage, advanced glycation end products (AGE) accumulate.9 AGEs include carboxymethyllysine (CML) and carboxyethyllysine (CEL), which are lysine-derived AGEs, and hydroimidazolone derivatives (GO-H and MG-H), which are arginine-derived AGEs.10 Additionally, dityrosine cross links, resulting from the oxidation of tyrosine residues, have been detected in IF.11 Ingested Maillard-modified proteins are proposed to bind to AGE receptors located on intestinal epithelial cells, thereby activating the key intracellular inflammatory mediator, NF-κB and induction of inflammation.12,13 These effects are supported by other studies showing that IF is suboptimal in protecting newborn infants against infections as compared to breast fed infants.14 Moreover, very low birth weight preterm infants fed IF have been found to develop serious immune-related illnesses such as necrotizing enterocolitis (NEC), which are not frequently observed in breast-fed neonates.15 Although the reason for this may be linked to the disparate composition of IF compared to breast milk, the denaturation of protective bioactive proteins induced by the severe thermal processes applied to IF may be a causative factor.1,16 Heat-induced structural changes of the same IF as used in the present study have recently been characterized using centrifugal field-flow fractionation with multi-angle light scattering.17 Compared to proteins in native conformation, aggregated proteins are absorbed differently in the gastrointestinal tract and have opposing immune-stimulatory effects in vitro and in vivo.18 Thus, we hypothesized that the severity of UHT treatment induced a greater degree of protein aggregation of bioactive milk proteins contained in IF thereby affecting the conformation of proteins, their digestion and release of bioactive peptides.
2. Materials and methods
2.1. Chemicals
Deionized water was obtained from a Milli-Q pure water system (Millipore Corporation, Bedford, MA, USA) and used for all solutions. Dithiothreitol (DTT), Brilliant Blue G acid blue 90 and iodoacetamide, phosphoric acid were obtained from Sigma-Aldrich Biochemie GmbH (Steinheim, Germany). LDS sample buffer was purchased from Thermo Fischer Scientific (Roskilde, Denmark). Ammonium sulphate, Tween 20 were purchased from PanReac Applichem (Barcelona, Spain). Sodium chloride was purchased from Merck (Damstadt, Germany).
2.2. Protein samples
Model IF were produced by Arla Foods Ingredients, Denmark as described previously,17 and a selection of the same samples were used in the present study. The initial protein content was provided by Arla Foods Ingredients, measured using Kjeldahl analysis. Samples were processed by either LP, D- or ID-UHT. Following production, the samples were immediately stored frozen at −60 °C. D- and ID-treated samples were also stored under aseptic conditions at 40 °C for 30, 60 and 120 days. After storage the samples were kept at −60 °C until analysis. Further details on sample composition and preparation can be found elsewhere.17 All the samples were stored aseptically in 500 mL polyethylene terephthalate bottles. On each sampling day, IF samples were thawed at room temperature and were carefully mixed before being divided into smaller aliquots.
2.3. Protein separation and aggregate analyses
Sodium dodecyl sulphate-polyacrylamide gel electrophoresis (SDS-PAGE) was performed on all samples. For reduction, 0.5 M DTT solution was added to the samples to a final concentration of 50 mM DTT and incubated at 56 °C for 30 min. Alkylation of the reduced samples was performed by addition of a 500 mM iodoacetamide solution to a final concentration of 10 mM iodoacetamide, followed by incubation in the dark for 30 min at ambient temperature before performing SDS-PAGE. Samples were standardized to the same protein concentration by dilution with Milli-Q water to 1% w/v before addition of 4× LDS sample buffer and heat treatment at 80 °C for 10 min. Ten μL of each sample (corresponding to 10 μg of total protein) were loaded in each well of a precast 12% Bis-Tris gel (NuPAGE, Thermo Fischer Scientific, Roskilde, Denmark). Novex Sharp and PageRuler pre-stained protein markers (Thermo Fischer Scientific, Roskilde, Denmark) were also loaded to each gel and proteins were separated at 200 V with NuPAGE MOPS SDS running buffer (Thermo Fischer Scientific) for 1 h. Proteins were stained with 0.2% Coomassie Brilliant Blue R-250 solution in 85% phosphoric acid, ammonium sulphate and 96% ethanol. The gels were destained in Milli-Q water and scanned using an Epson Perfection V750 Pro scanner (Seiko Epson Corporation, Nagano, Japan). Comparison between gel bands was based on volumes computed by using Phoretix TL120 software, with a rolling-ball 90 algorithm for background subtraction.
2.4. Western blot for dityrosine detection
Dityrosine cross-linking was detected by western blot, using a 3,3′-dityrosine monoclonal antibody (Japan Institute for the Control of Aging [JaICA], Shizuoka, Japan, cat. no.: MDT-020P). The secondary antibody was sheep anti-mouse IgG conjugated to Horseradish Peroxidase (NXA931). SDS-PAGE was performed as described above. Proteins were then transferred to a PVDF (Polyvinylidene Difluoride) membrane using an Iblot 2 system (Thermo Fisher, Roskilde, Denmark). The membrane was then rinsed in 25–30 mL TBS (Tris-Buffered Saline) for a few seconds, followed by blocking of the membrane with 25–30 mL TBST-BSA (TBS with 0.1% of Tween 20 and BSA) for 4 h. The PVDF membrane was incubated with primary antibody (1
:
3000 dilution in TBST-BSA) overnight at 4 °C. The membrane was then washed in 25–30 mL TBST for 6 × 5 min at room temperature on a rocking table. The membrane was then incubated with secondary antibody (1
:
3000 dilution in TBST-BSA) for 1 h and washed again in 25–30 mL TBST for 6 × 5 min. The membrane was incubated with ECL substrate (Enhanced Chemiluminescent substrate) and scanned using a ChemiDoc Touch Imaging System (Biorad, CA, US).
A positive control for dityrosine was prepared by oxidation of α-lactalbumin (10 mg mL−1; Sigma L-6010) dissolved in 10 mM ammonium acetate (pH 6.9) and incubated at 37 °C for 60 min in the presence of 50 μL of horseradish peroxidase (10 mg mL−1) and hydrogen peroxide (2 μL, 0.5 M). Catalase (EC 1.11.16, C-100, Megazyme, Ireland) (20 μL) was added after 10 min to stop the reaction. The dityrosine cross-linked apo α-lactalbumin was stored at −20 °C.
2.5.
In vitro digestion of IFs
IFs (LP, D, and ID unstored samples and D and ID stored for 60 days) were subjected to in vitro digestion by pepsin at pH 4, followed by pancreatin at pH 6.5, thereby simulating the conditions in the infant gastrointestinal tract.19 For pepsin digestion, 33.33 mL of each IF (∼0.5 g protein) was added into a 50 mL Falcon tube and incubated at 37 °C in a water bath for 30 min. Magnetic stirring was applied to ensure the homogeneity of samples. The pH was rapidly adjusted to 4 to avoid casein precipitation by 1 M HCl and pepsin (Sigma P7000, 250 units per mg solid) was then added at a protein/enzyme ratio of 1.25% (w/w). After 2 h of pepsin digestion, 1 mL of each digested sample was transferred to an Eppendorf tube stored on ice and 10 μL of 1 mg mL−1 Pepstatin A (Sigma 77170) was added to terminate the reaction. The pH of each sample was adjusted to 6.5 rapidly by 1.43 M sodium bicarbonate (Fluka, Roskilde, Denmark) and then pancreatin (Sigma P7545, 8 × USP units) was added at a protein/substrate ratio of 1.5% (w/w). Following 2, 4 and 6 h of pancreatin digestion, 1 mL of each digested sample was transferred to an Eppendorf tube placed on ice and 100 μL of cOmplete Protease inhibitor cocktail (1 tablet in 25 mL of Milli-Q water, Roche, Basel, Switzerland) was added to terminate the reaction. Samples were stored at −60 °C until analysis.
2.6. Peptide analysis by LC MS/MS and hierarchical clustering analysis
Digested IFs (LP, D, and ID samples and D and ID stored for 60 days) were added trifluoroacetic acid to a final concentration of 1% and placed on ice for 30 min. Samples were centrifuged (20
000g at 4 °C for 15 min) to remove any lipids and precipitated protein and the aqueous peptide-containing phase was collected for LC-MS/MS analysis. The analysis was performed on a UHPLC Ultimate 3000 (Thermo Fisher Scientific, Roskilde, Denmark) using a C18 column (Aeris Peptide C18) of dimensions 150 × 2.1 mm, containing 1.7 μm particles with a pore size of 100 Å operated at 40 °C (Phenomenex, Værløse, Denmark). Sample (50 μL) was injected at a flow rate of 0.25 mL min−1. The gradient was 100% of buffer A (0.1% formic acid in Milli-Q water) for 5 min followed by a linear increase from 0–50% buffer B (0.1% formic acid in 99.9% acetonitrile) over 25 min. The separated peptides were applied to a heated electrospray ionization (HESI) source which was connected to a Q Exactive mass spectrometer (Thermo Fisher Scientific, Bremen, Germany). A full MS scan of the m/z 300–1500 range was acquired in the Orbitrap. The seven most intense ions from the MS were isolated. Ions with unassigned and higher than 8+ charge state were excluded from MS2 selection. The normalized collision energy (NCE) was set at 28. For the analysis of the mass spectra, the software MaxQuant 1.6.6.0 was used.20 The data was searched against the Bos Taurus proteome in the Swissprot database (SwissProt TaxID = 9913). Main search peptide tolerance was set at 4.5 ppm. The standard settings were used in MaxQuant except that the unspecific digestion mode was chosen, the alignment time window was changed to 10 min, the minimum peptide length was set as 4 and the modification lactulosyllysine (+324.105647 Da), oxidation of Met, His, Trp, Tyr and Phe (+15.994915 Da) were specified as variable modifications. The degree of lactosylation of the released peptides was estimated by the percentage of modification among all forms of peptides, i.e., the intensity of the modified peptide divided by the intensities of the sum of unmodified and modified peptides. The average of the peak areas obtained for each peptide was analyzed in six replicates (two biological × three injection replicates). The relative level of lactosylation of the released peptides in the different samples were calculated as an average of different modified peptides. It should be mentioned that this method has a limitation, as it assumes equal ionization of all forms of peptides, which is not often the case for all modifications. All the MS raw data and the results from the search engine have been uploaded to MassIVE database (massive.ucsd.edu), with identifier MSV000088189.
The bioactive peptides were searched against the Milk Bioactive Peptide Database established by Nielsen et al.21 To group peptides, hierarchical clustering was utilized using Perseus version 1.6.5.0.22 Peptides were grouped according to their differences of log2 peptide intensity.
3. Results
The in vitro digestion pattern of LP, D, ID, as well as D and ID stored for 60 days were analysed by SDS-PAGE (Fig. 1). In ascending order of size, the major proteins in undigested LP consisted of α-La (14 kDa), β-Lg (18 kDa), CN (∼25 kDa) and finally protein aggregates which were characterized by a diffuse fusion of protein bands (∼80 kDa–260 kDa). Compared to LP, undigested D samples contained more aggregated protein as observed by a smear extending between the location of the α-La, β-Lg and CN protein bands and an even more pronounced high-molecular weight band. The aggregate band of D extended above 260 kDa. ID clearly contained lower levels of α-La and β-Lg and the fusion of the aggregates was stained more intensely, the molecular weight of the ovally-shaped aggregates was even greater than 260 kDa. D and ID stored for 60 days showed further loss of unaggregated α-La, β-Lg, CN and aggregates.
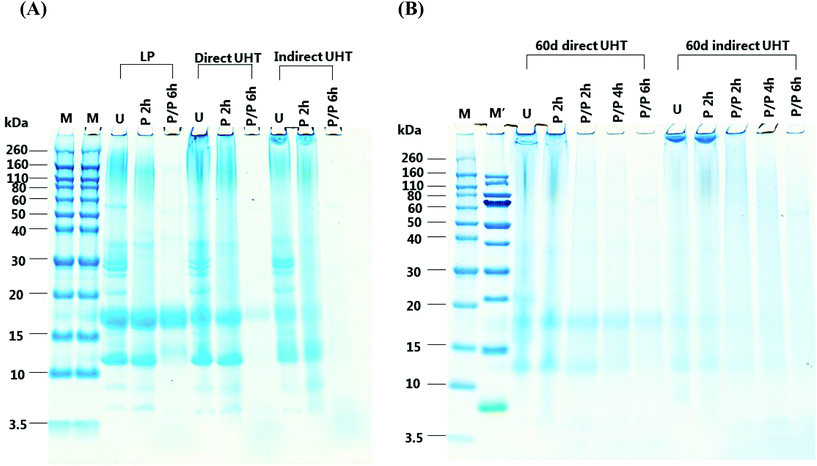 |
| Fig. 1 SDS-PAGE of liquid infant formula (IF) subjected to different thermal treatments and storage followed by in vitro digestion. IF was either subjected to low pasteurization (LP), direct UHT (D) or indirect UHT (ID) treatment. UHT treated IF were either (A) not stored, or (B) stored for 60 days at 40 °C to simulate prolonged storage conditions. U: undigested sample; P 2 h: pepsin digestion for 2 h; P/P 2 h: combined pepsin + pancreatin digestion for 2 h; P/P 4 h: combined pepsin + pancreatin digestion for 4 h; P/P 6 h: combined pepsin + pancreatin digestion for 6 h. Samples were compared to molecular weight markers, M means Novex Sharp marker, M′ means PageRuler marker. | |
For LP, most of the β-Lg and α-La were resistant to pepsin digestion for 2 h, while a proportion of higher molecular weight aggregates (60–160 kDa) remained undigested. Pepsin digested D contained lower levels of α-La and β-Lg compared to pepsin digested LP, and the majority of aggregates in D remained undigested. Pepsin digested ID contained significantly lower amounts of α-La and β-Lg than LP and D. The high molecular weight aggregates in ID samples were overall resistant to pepsin digestion. Pepsin digestion did not have a significant effect on α-La and β-Lg in 60 days-stored D and ID, and compared to undigested samples there was very little loss of high molecular weight aggregates.
Following 6 h of combined pepsin and pancreatic digestion all proteins, including aggregates, were digested with the exception of β-Lg and a ∼13 kDa protein band in the LP sample. For D, significantly lower levels of β-Lg were present than in LP, being the only protein resistant to digestion. In contrast, all protein was digested in ID. In D stored for 60 days, a very small amount of β-Lg remained undigested, whereas all β-Lg was digested in stored ID, however some smeared aggregate bands were observed.
To determine the nature of the interaction involved in aggregate formation, D and ID samples stored for 30, 60 and 120 days were reduced and alkylated (Fig. 2A). Chemical reduction of the samples restricted the mobility of the lower molecular weight proteins (mainly α-La and β-Lg) during the electrophoresis as a consequence of protein unfolding. Lowered amounts of aggregates were remained following reduction and alkylation. Western blot analysis using a monoclonal antibody specific for dityrosine showed no staining of dityrosine in neither D nor ID samples stored for 60 days, but diffuse dityrosine staining was identified after 120 days of storage (Fig. 2B).
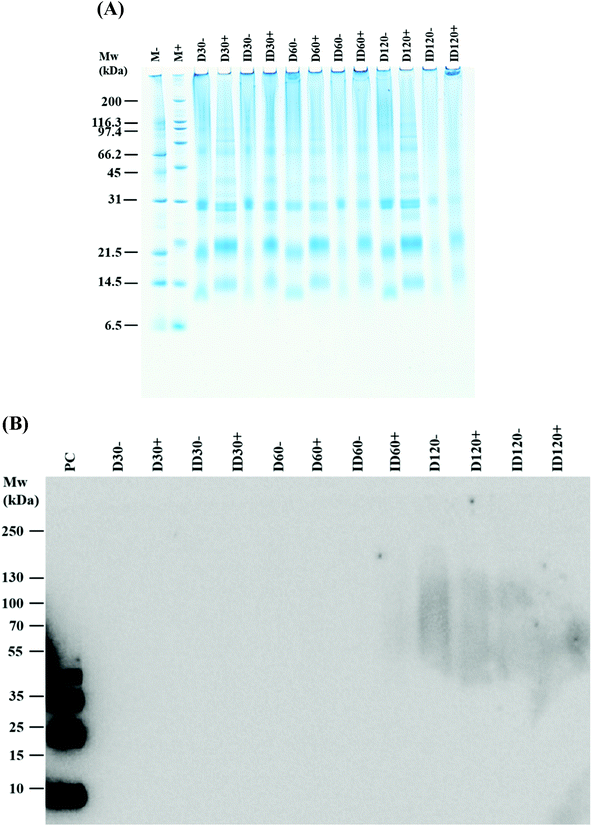 |
| Fig. 2 SDS-PAGE of reduced and alkylated direct (D) and indirect (ID) UHT-treated liquid infant formula (IF) samples (A); (B) identification of dityrosine in UHT-treated IF. Non-reduced and reduced D and ID were probed by a monoclonal antibody raised in mice against 3,3-dityrosine and visualised by a secondary anti-mouse IgG conjugated with horseradish peroxidase. M: molecular weight marker, D30, D60, and D120: D stored for 30, 60 and 120 days, respectively. ID30, ID60, and ID120: ID stored for 30, 60, and 120 days, respectively. Symbols (−) and (+) indicate without and with reduction, respectively. P.C.: dityrosine positive control prepared from apo α-lactalbumin by horseradish peroxidase and hydrogen peroxide. | |
Peptides present in undigested and digested IF were identified by LC-MS/MS. All undigested IFs contained peptides, which were released by endogenous milk protease activity. When comparing the total number of released peptides in undigested IFs, the lowest levels were observed in LP and ID with no significant differences between these, while the highest levels were observed in D and 60-days stored D samples (Fig. 3A). Compared to β-Lg and α-La (Fig. 3C and D), greater number of peptides were released from β-CN due to endogenous protease activity (Fig. 3B), whereas the 60-days stored D contained the greatest peptide numbers (Fig. 3B), and a negligible number of peptides were released from α-La in undigested samples (Fig. 3D). The number of peptides released from β-Lg was less than from β-CN but no significant differences were noted between undigested samples, with the exception of LP (Fig. 3C). Following pepsin digestion, the total number of peptides released was greatest in the unstored and 60-days stored D sample (Fig. 3A). In vitro digestion released a profile of β-CN peptides, which correlated with the distribution of total peptides (Fig. 3A) released from all of the IF samples. This tendency also applied for β-Lg (Fig. 3C). The number of peptides released from α-La was clearly lowest, potentially reflecting the concentration of α-La in the IF samples as compared to the amounts of CN and β-Lg (Fig. 3D). The protein composition of the IFs was determined in a previous study by RP-HPLC-UV to be α-La
:
CN
:
β-Lg = 10%
:
30%
:
45%, respectively.17 For β-Lg, greater peptide numbers were released from D and ID after pepsin digestion, but storage for 60 days lowered the peptide release. Combined pepsin and pancreatin digestion augmented peptide release, but there were no differences between thermal treatments although peptide numbers were greatest in D stored for 60 days (Fig. 3C). The number of α-La peptides increased significantly and storage of D resulted in the greatest release of peptides (Fig. 3D).
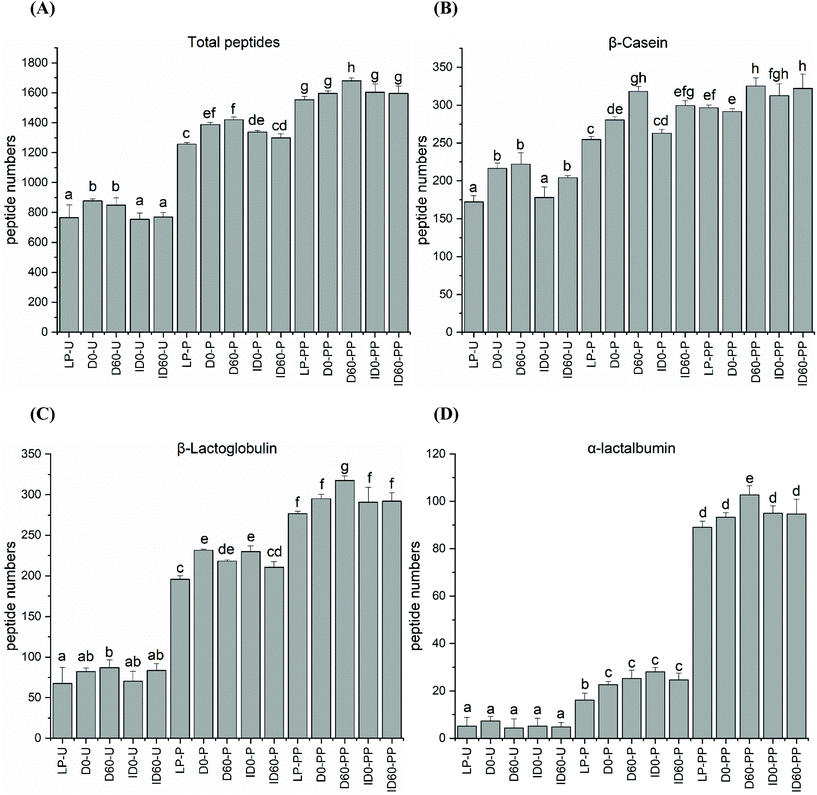 |
| Fig. 3 (A) Total number of peptides released during in vitro digestion of liquid infant formula subjected to different thermal treatments with and without storage; (B) peptides released from β-CN; (C) peptides released from β-Lg; (D) peptides released from α-La. LP-U: undigested low pasteurised; D0-U: undigested D; D60-U: undigested D stored for 60 days; ID0-U: undigested ID; ID60-U: undigested ID stored for 60 days; samples with codes followed by P were digested with pepsin at pH 4 at 37 °C for 2 h; samples with codes followed by PP were digested with pepsin at pH 4 for 2 h followed by pancreatin at pH 6.5 for 6 h at 37 °C. ANOVA with Tukey's post-test and corrections for multiple comparisons was used (P < 0.05). | |
When the numbers of released peptides were correlated to peptide length, the created distribution indicated that the majority of peptides for β-Lg in all IF samples were of 5–8 amino acids in length (Fig. 4). After pepsin digestion, the majority of released peptides contained between 5–8 and 9–12 amino acids. The proportion of these peptides increased after pepsin and pancreatin digestion.
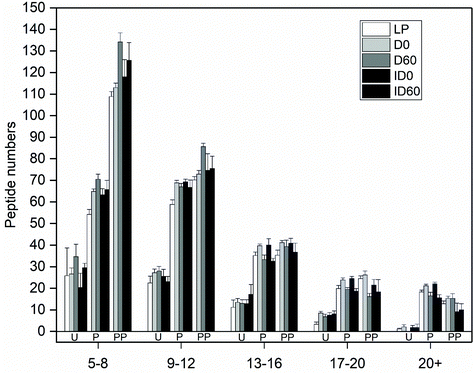 |
| Fig. 4 Distribution of peptide numbers versus peptide lengths released from β-Lg following in vitro digestion of liquid infant formula subjected to different thermal treatments. LP: low pasteurized; D0: unstored direct UHT treated; D60: D stored for 60 days; ID0: unstored indirect UHT treated; ID60: ID stored for 60 days; U: undigested IF; samples with codes followed by P were digested with pepsin at pH 4 at 37 °C for 2 h; samples with codes followed by PP were digested with pepsin at pH 4 for 2 h followed by pancreatin at pH 6.5 for 6 h at 37 °C. The error bar represents the standard deviation of the replicates. | |
Nineteen peptides with reported bioactivity were released from the major protein present in the IF samples, β-Lg. Released peptides had inhibitory effects against the enzymes dipeptidyl-peptidase IV (DPP-IV) and angiotensin converting enzyme (ACE), or with antimicrobial, proliferative or cytotoxic effects, and hypocholesterolemic properties.21 Hierarchical clustering analysis enabled the grouping of peptides released from the differently thermally-treated IFs (Fig. 5). Compared to undigested IF, in which almost no bioactive peptides were detected (green shading) except some antimicrobial peptides (black shading at the bottom right-hand side of the figure), the total number of bioactive peptides released from β-Lg increased during the different stages of digestion with intensities changing from low (green color) to high (red color) in Fig. 5. Following pepsin digestion, large amounts of DPP-IV inhibitory peptides were released, which included peptides IQKVAGTW (amino acid no. 12–19), LKALPMH (amino acid no. 140–146), LKPTPEGDL (amino acid no. 46–54), and LKPTPEGDLEIL (amino acid no. 46–57) (Fig. 5, red colour, middle upper section). UHT-treatment facilitated greater release, but storage had an inhibitory effect on the levels of these bioactive peptides. Following pancreatin digestion, a large amount of ACE inhibitory peptides were released, including peptides LDIQK (amino acid no. 10–14), ALPM (amino acid no. 142–145), YLLF (amino acid no. 102–105), VLDTDY (amino acid no. 94–99), VLDTDYK (amino acid no. 94–100), GLDIQK (amino acid no. 9–14), and VLVLDTDYK (amino acid no. 92–100) (Fig. 5, red colour, bottom left section). The identity of these peptides showed that many of them contained a C-terminal lysine residue, which could be correlated with the tryptic specificity of pancreatin. UHT treatment further increased levels of the majority of these peptides. Storage had a significant effect on peptide release after pepsin and pancreatin digestion, as D and ID stored for 60 days were grouped in a different hierarchy compared to unstored samples. Inspection of the hierarchical clustering shown in Fig. 5, indicates that overall, storage increased the quantities of several peptides but it did not have any significant effects on the release of bioactive peptides ending with C-terminal lysine residue. In general, the magnitude of these peptides was more intense in pepsin and pancreatin digested IF compared with just pepsin digested and undigested IF. Storage of D and ID reduced levels of the DPP-IV and ACE inhibitory peptides IQKVAGTW (amino acid no. 28–35), the DPP-IV and the antimicrobial peptide, VLVLDTDYK (amino acid no. 141–151), and the ACE-inhibitory peptide ALPM (amino acid no. 158–161). While both D and ID induced peptide release from β-Lg, D was the most effective thermal processing to stimulate digestive cleavage as compared to ID. Average relative level of lactosylation in the released peptides from β-Lg was determined by LC-MS/MS in pepsin digested and pancreatin digested IFs, respectively (Fig. 6). Lactosylation was detected in a total of 8 lysine residues; Lys 8, 14, 47, 91, 100, 135, 138 and 141 (Fig. 6A). As expected, the degree of lactosylation in the released peptides from LP and unstored D and ID was lower than for stored samples. In pepsin digested D and ID, the degree of lactosylation in the released peptides clearly increased with storage and was highest in ID (P < 0.05, Fig. 6B). However, the difference in lactosylation degree between unstored and stored D and ID was lower in pepsin and pancreatin digested samples (Fig. 6C).
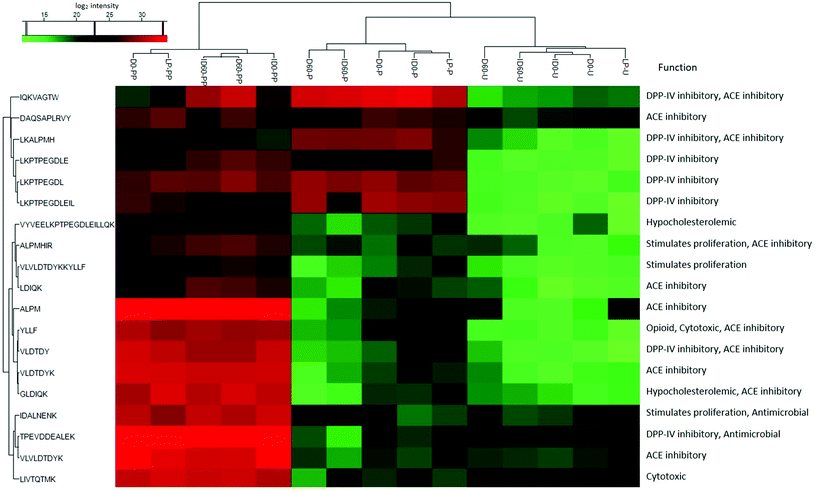 |
| Fig. 5 Distribution of bioactive peptides released from β-Lg following in vitro digestion of liquid infant formula subjected to different thermal treatments. LP: low pasteurized; D0: unstored direct UHT treated; D60: D stored for 60 days; ID0: unstored indirect UHT treated; ID60: ID stored for 60 days; U: undigested IF; samples with codes followed by P were digested with pepsin at pH 4 at 37 °C for 2 h; samples with codes followed by PP were digested with pepsin at pH 4 for 2 h followed by pancreatin at pH 6.5 for 6 h at 37 °C. The colors indicate the differences of the log2 peptide intensity: highest intensity (red), medium intensity (black) and lowest intensity (green). | |
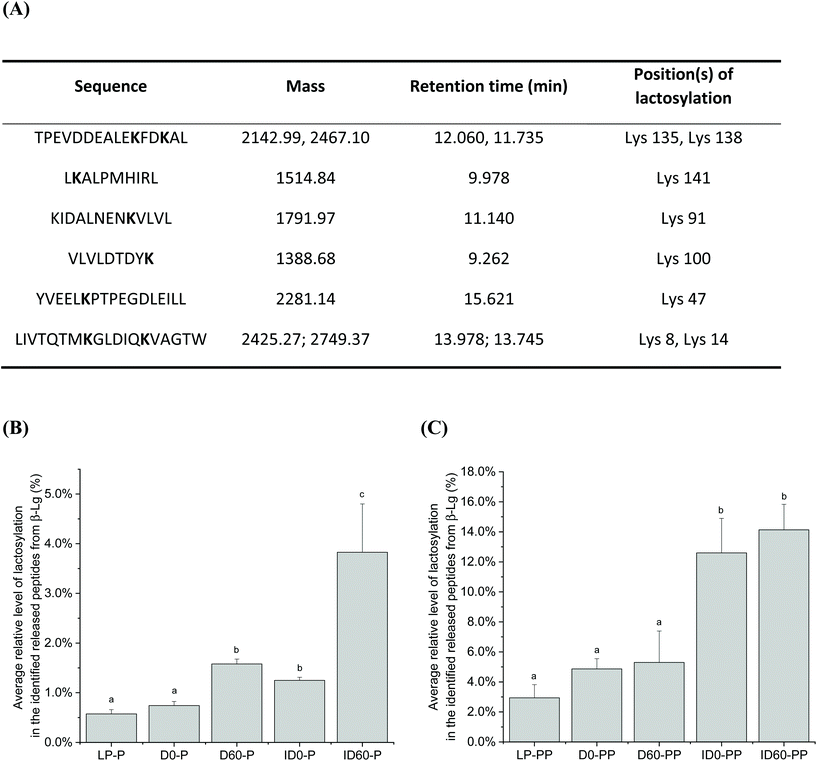 |
| Fig. 6 Average relative level of lactosylation in the identified released peptides from β-Lg following in vitro digestion of liquid infant formula subjected to different thermal treatments. Panel A: Information of the lactosyllysine-modified peptides identified by LC-MS, including the sequence, the detected mass, retention time and the modified position. Panel B: The degree of lactosylation in the released peptides from liquid infant formula after pepsin digestion; Panel C: the degree of lactosylation in the released peptides from liquid infant formula after pepsin followed by pancreatin digestion. LP: low pasteurized; D0: unstored direct UHT treated; D60: D stored for 60 days; ID0: unstored indirect UHT treated; ID60: ID stored for 60 days; U: undigested IF; P: in vitro pepsin digestion; PP: in vitro pepsin and pancreatin digestion. Peptides with two masses represent lactosylation took place in either one or both of the lysine residues. ANOVA with Tukey's post-test and corrections for multiple comparisons was used (P < 0.05). | |
4. Discussion
This study provides information firstly, about the severity of thermal treatments applied to IF and how these treatments and storage affect the stability of bioactive proteins through enhancement of aggregate formation and secondly, how these treatments affect the digestibility of IF proteins. Thus, the results help to elucidate the effects of prolonged storage of UHT-treated IF at temperatures mimicking those during global shipping in containers and during storage in warm ambient conditions.
4.1. Digestive pattern and the nature of aggregates in IFs
Undigested IF contained aggregates of molecular weight ranging from ∼60–260 kDa. Although this formula has only been subjected to low pasteurisation (72 °C, 15 s), the presence of these aggregates is explained by the pasteurisation and spray drying of individual milk-based ingredients which are subsequently supplemented to IF.17 Unlike LP, very pronounced levels of aggregates were observed in the D and ID in agreement with the study by Lund et al.17 For D, the degree of aggregation was surprising considering the short duration of applied heat load (injected steam, ∼135 °C for 6 s). In ID, a more pronounced aggregate formation resulted from the extended heat load applied to the formula. Reduced levels of individual proteins (α-La, β-Lg and CN) were positively correlated with the severity of heat treatment and with a corresponding increase in high molecular weight aggregates (>260 kDa) being most prevalent in unstored ID compared to D and LP (Fig. 1A), and also in stored ID compared to stored D. An increased browning color and decreased pH was also correlated with the severity of heat treatment, which has recently been investigated.17 Chemical reduction of the samples resulted in a loss of aggregate bands and the emergence of increased concentrations of unaggregated α-La, β-Lg and BSA implying the participation of these proteins in aggregate complexes. However, although this suggests that many aggregates were composed of disulphide-linked covalent bonds which are cleaved during reduction, it does not rule out other forms of covalent interactions, as many aggregates still remained intact after chemical reduction. Such non-reducible covalent crosslinks can be derived from β-elimination of cysteine or serine residues leading to dehydroalanine, which then may react with lysine or cysteine to form lysinoalanine or lanthionine, respectively. The formation of dityrosine, another non-reducible cross-link commonly formed during oxidation, was evaluated in the present study, but only diffuse dityrosine-linked protein aggregates were detected after 120 d of storage corresponding to those observed following Coomassie Blue staining. Although non-covalent bonds may also be involved in aggregate formation, these are likely to be prevented due to the presence of LDS in the electrophoresis sample buffer.
4.2. Digestibility of non-aggregated α-La and β-Lg increases with the severity of heat treatment
Compared to adults who have gastric and intestinal pH of ∼2 and 8, respectively, we conducted pepsin digestion at pH 4, followed by pancreatic digestion at pH 6.5 representing pH conditions in the infant stomach and intestine, respectively.19,23 It is known that when pepsin digestion is performed at pH 2 (the pH optimum of pepsin), negligible β-Lg digestion occurs, but all other milk proteins are digested.24 β-Lg is stable under these conditions due to its compact β-barrel structure.25 Under the higher pH (pH 4) employed during this study, both α-La, β-Lg and aggregates resisted pepsin digestion in LP. However, heat treatment induced major structural changes as non-aggregated α-La and β-Lg were digested to a higher degree by pepsin in accordance with the severity of thermal treatment (ID > D), providing increased enzymatic access. Contrastingly, the resistance of aggregates to pepsin digestion in the UHT-treated samples and after storage suggests that aggregates impart a hindrance to pepsin cleavage. Pepsin followed by pancreatin digestion had little effect on cleavage of β-Lg contained in LP, but UHT treatments enhanced β-Lg digestibility, with small amounts of this protein remaining after 6 h of treatment. Although most β-Lg was digested after 60 d of storage in ID, significant aggregates remained undigested. The resistance of β-Lg and aggregates to digestion during the different treatments may have important physiological implications for the infant. Pasteurization of milk proteins (33 min at 63 °C and 2 min at 72 °C) promotes aggregation reducing uptake via intestinal epithelial cells and redirecting uptake to intestinal Peyers patches thus stimulating a Th2 response.18 There is a delicate balance between Th1 and Th2 responses in infants, shifting in time after birth towards an antibody and cytokine productive Th2 response. As Th1 responses involve secretion of anti-viral IFN-γ, this imbalance may impair anti-viral responses and sensitize allergic responses.26 Moreover, a recent study has indicated that whey-based aggregates may induce immunomodulation by stimulating cellular toll-like receptors.27
4.3. Peptide numbers following the digestion of IFs
Heat treatment had an impact on the release of peptides during digestion. Peptide analysis of non-digested samples indicated that approximately 750 unique peptides could already be detected in the LP and ID, with approximately 900 unique peptides in D. The activity of milk-derived enzymes, such as plasmin and Cathepsin D contribute to the release of comparable quantities of peptides in human milk.28 Plasmin, an intrinsic milk enzyme, is controlled by the delicate balance between its precursor plasminogen, plasminogen activators and plasminogen activator inhibitors. While plasminogen activators are known to be heat stable, the inhibitors are heat labile.29,30 Thus, D-UHT activates plasmin-induced proteolysis due to the thermal inactivation of plasmin inhibitors, whereas the heat load applied during ID-UHT treatment is so high that only limited plasmin activity remains.31 These findings explain the increased numbers of peptides that were observed in the undigested D. Sample storage did not increase total peptide numbers, but there were some small protein-dependent differences. CNs were the most susceptible proteins to indigenous milk enzymes, followed by β-Lg and then α-La. The random structure exhibited by CN, compared to globular whey proteins, together with the dissolution of CN micelles at the acidic pH of pepsin digestion can explain these observations. During pepsin digestion, peptides increased in the UHT-treated IF samples compared to LP. However, pepsin followed by pancreatin treatment did not increase the number of peptide released from CN further, presumably because the majority of CN had already been rapidly digested by pepsin. In comparison, β-Lg peptides increased significantly following pancreatin digestion as a consequence of the resistance of this protein during the gastric phase of digestion. Likewise, a very significant increase in peptides derived from α-La occurred during pepsin followed by pancreatin digestion, as α-La was not greatly cleaved by pepsin under these conditions.
4.4. Peptide length distribution and release of bioactive peptides from β-Lg
Pepsin digestion produced approximately 50 long peptides (length >17 amino acids) which were afterwards digested by intestinal proteases contained in pancreatin, resulting in many smaller peptides of 5–8 amino acids in length. This is in agreement with a recent study which reported that at 60 min, intestinally digested IF mostly contained whey-protein derived peptides of 5–9 amino acids in length.32 There were not great differences between D and ID treatment in terms of peptide length distribution in β-Lg. However, storage increased the proteolytic release of short peptides (5–8 amino acids) and lowered the number of long peptides (>13 amino acids) compared with non-stored UHT-treated IF samples. Similar results were found for β-CN-derived peptides. This may be due to proteolysis induced during the 60 days of storage, by milk-derived endogenous enzymes.
Peptide length has an important physiological relevance, as tri- and tetrapeptides are absorbed by the intestinal PepT1 transporter, whereas longer peptides are important for other biological effects. Based on the peptide identity, several β-Lg peptides have reported ACE inhibitory properties, and thus blood pressure lowering effects. An alternative major peptide fraction has reported DPP-IV inhibitory actions. High levels of DPP-IV are present in the jejunum. Although inhibition of DPP-IV is involved in glucose homeostasis via lowering of blood glucagon and through inhibition of GLP-1 and GIP, inhibition also reduces the pro-inflammatory activity of DPP-IV.33 β-Lg-derived bioactive peptides released from IF may therefore result in anti-inflammatory effects reducing oxidative stress and apoptosis.34 Despite this, the yield of these β-Lg peptides may be modulated by the effects of the Maillard reaction, which occurs during heat treatment of high lactose-containing IFs and their subsequent storage. In the present study, 8 lysine residues were identified as being lactosylated, which is a lower number than what has been reported previously, e.g. 15 lysine residues were found to be modified in β-Lg in a study with UHT treated milk.35 Lactosyllysine will further react to form AGEs during storage, which may explain the lower number of lactosylated sites identified here as compared to previous studies. Lactosyllysine and AGEs impede the digestion of milk proteins and peptide release due to steric hindrance and lysine blockage, which impairs enzymatic cleavage.4,36 Overall, pepsin hydrolysis predominantly released peptides from β-Lg with DPP-IV inhibitory activity. The amino acid sequence of these peptides corresponds with residues known to be lactosylated on lysine.35 Compared to LP treated with pepsin, both pepsin-hydrolysed D and ID released increased amounts of the DPP-IV inhibitory peptide IQKVAGTW (amino acids 12–19), having the highest intensity in pepsin hydrolyzed ID. The increased amounts of this peptide are likely to occur due to the structural effects induced by heat treatment, which will allow access for pepsin to induce increased proteolysis. However, peptide yield was lower when the samples were stored (pepsin hydrolysed D and ID stored for 60 days), correlating with increased lactosylation and aggregation compared to pepsin hydrolysed LP (Fig. 6B and 1). Similar lactosylation-induced reductions of peptide release during storage was observed for other peptides.
When IFs were subsequently digested with pancreatin, more bioactive peptides were released which is likely due to the many different enzymes present in pancreatin. Pancreatin induced more cleavages than pepsin digestion alone, as pepsin may increase the accessibility of β-Lg to pancreatin enzymes by cleaving surface β-sheet structures, providing easier to access the β-barrel structure of β-Lg.37 Interestingly, several released bioactive peptides shared lysine at the C-terminal during pancreatin digestion, e.g., VLDTDYK, GLDIQK, IDALNENK in Fig. 5, which could be a consequence of the C-terminal lysine containing peptides being released from the core of β-Lg, corresponding with Lys100, Lys91 and Lys135 in the present study. Although they are lactosylated especially in the stored IF (Fig. 6C), the rest unmodified forms of these peptides could still be cleaved due to the potent tryptic-specified activity of pancreatin.
5. Conclusion
This study demonstrated that UHT treatment and storage of ready-to-feed liquid infant formulas affects the in vitro digestibility and the release of bioactive peptides primarily due to structural changes and induction of covalent protein aggregation. Careful selection of thermal treatment and storage conditions may optimize the physiological effects of bioactive peptides released after the digestion of infant formulas.
Author contributions
Y. Y., K. E. K., Y. F., C. F. N., A. J., M. N. L., and D. E. W. C., contributed to the concept and design of the study. Y. Y., K. E. K., Y. F., A. J. conducted the study and performed the data analysis. All authors contributed to the data interpretation and manuscript writing. M. N. L. and D. E. W. C. were responsible for funding and supervision. D. E. W. C. had primary responsibility for the final content. All authors accepted the final manuscript.
Conflicts of interest
C. F. N. is employed at Arla Foods Ingredients. The remaining authors have no conflicts of interest.
Acknowledgements
The present study was supported by the Green Development and Demonstration Programme (GUDP) under the Ministry of Environment and Food of Denmark [grant no. 34009-17-1278], The Danish Dairy Research Foundation, and Arla Foods Ingredients, Denmark who also provided the IF samples.
References
- D. E. W. Chatterton, D. N. Nguyen, S. B. Bering and P. T. Sangild, Anti-inflammatory mechanisms of bioactive milk proteins in the intestine of newborns, Int. J. Biochem. Cell Biol., 2013, 45, 1730–1747 CrossRef CAS PubMed.
- W. W. Hay Jr. and K. C. Hendrickson, Preterm formula use in the preterm very low birth weight infant, Semin. Fetal Neonatal Med., 2017, 22, 15–22 CrossRef PubMed.
- H. Tran, N. Datta, M. J. Lewis and H. C. Deeth, Predictions of some product parameters based on the processing conditions of ultra-high-temperature milk plants, Int. Dairy J., 2008, 18, 939–944 CrossRef CAS.
- H. E. Zenker, G. A. A. van Lieshout, M. P. van Gool, M. C. E. Bragt and K. A. Hettinga, Lysine blockage of milk proteins in infant formula impairs overall protein digestibility and peptide release, Food Funct., 2020, 11, 358–369 RSC.
- M. N. Lund, K. Olsen, J. Sørensen and L. Skibsted, Kinetics and mechanism of lactosylation of α-lactalbumin, J. Agric. Food Chem., 2005, 53, 2095–2102 CrossRef CAS PubMed.
- K. Aalaei, I. Sjoholm, M. Rayner, C. Teixeira and E. Tareke, Early and advanced stages of Maillard reaction in infant formulas: Analysis of available lysine and carboxymethyl-lysine, PLoS One, 2019, 14, e0220138 CrossRef CAS PubMed.
- S. Cattaneo, F. Masotti and L. Pellegrino, Liquid Infant Formulas: Technological Tools for Limiting Heat Damage, J. Agric. Food Chem., 2009, 57, 10689–10694 CrossRef CAS PubMed.
- M. Teodorowicz, J. van Neerven and H. Savelkoul, Food Processing: The Influence of the Maillard Reaction on Immunogenicity and Allergenicity of Food Proteins, Nutrients, 2017, 9, 835 CrossRef PubMed.
- E. Guerra-Hernandez, C. Leon, N. Corzo, B. Garcia-Villanova and J. M. Romera, Chemical changes in powdered infant formulas during storage, Int. J. Dairy Technol., 2002, 55, 171–176 CrossRef CAS.
- W. Zhang, M. M. Poojary, V. Rauh, C. A. Ray, K. Olsen and M. N. Lund, Quantitation of alpha-Dicarbonyls and Advanced Glycation Endproducts in Conventional and Lactose-Hydrolyzed Ultrahigh Temperature Milk during 1 Year of Storage, J. Agric. Food Chem., 2019, 67, 12863–12874 CrossRef CAS PubMed.
- D. Scheidegger, R. P. Pecora, P. M. Radici and S. C. Kivatinitz, Protein oxidative changes in whole and skim milk after ultraviolet or fluorescent light exposure, J. Dairy Sci., 2010, 93, 5101–5109 CrossRef CAS PubMed.
- N. V. Chuyen, Toxicity of the AGEs generated from the Maillard reaction: on the relationship of food-AGEs and biological-AGEs, Mol. Nutr. Food Res., 2006, 50, 1140–1149 CrossRef PubMed.
- T. van der Lugt, A. R. Weseler, W. A. Gebbink, M. F. Vrolijk, A. Opperhuizen and A. Bast, Dietary Advanced Glycation Endproducts Induce an Inflammatory Response in Human Macrophages in Vitro, Nutrients, 2018, 10, 1868 CrossRef PubMed.
- K. G. Dewey, M. J. Heinig and L. A. Nommsen-Rivers, Differences in morbidity between breast-fed and formula-fed infants, J. Pediatr., 1995, 126, 696–702 CrossRef CAS.
- A. Lucas and T. J. Cole, Breast milk and neonatal necrotising enterocolitis, Lancet, 1990, 336, 1519–1523 CrossRef CAS.
- D. N. Nguyen, P. T. Sangild, Y. Li, S. B. Bering and D. E. W. Chatterton, Processing of whey modulates proliferative and immune functions in intestinal epithelial cells, J. Dairy Sci., 2016, 99, 959–969 CrossRef CAS PubMed.
- P. Lund, S. Bang Nielsen, C. Fiil Nielsen, C. A. Ray and M. N. Lund, Impact of UHT treatment and storage on liquid infant formula: complex structural changes uncovered by centrifugal field-flow fractionation with multi-angle light scattering, Food Chem., 2021, 129145 CrossRef CAS PubMed.
- F. Roth-Walter, M. C. Berin, P. Arnaboldi, C. R. Escalante, S. Dahan, J. Rauch, E. Jensen-Jarolim and L. Mayer, Pasteurization of milk proteins promotes allergic sensitization by enhancing uptake through Peyer's patches, Allergy, 2008, 63, 882–890 CrossRef CAS PubMed.
- O. Donmez, B. A. Mogol, V. Gokmen, N. Tang, M. L. Andersen and D. E. W. Chatterton, Modulation of gastrointestinal digestion of beta-lactoglobulin and micellar casein following binding by (-)-epigallocatechin-3-gallate (EGCG) and green tea flavanols, Food Funct., 2020, 11, 6038–6053 RSC.
- S. Tyanova, T. Temu and J. Cox, The MaxQuant computational platform for mass spectrometry-based shotgun proteomics, Nat. Protoc., 2016, 11, 2301–2319 CrossRef CAS PubMed.
- S. D. Nielsen, R. L. Beverly, Y. Y. Qu and D. C. Dallas, Milk bioactive peptide database: A comprehensive database of milk protein-derived bioactive peptides and novel visualization, Food Chem., 2017, 232, 673–682 CrossRef CAS PubMed.
- S. Tyanova, T. Temu, P. Sinitcyn, A. Carlson, M. Y. Hein, T. Geiger, M. Mann and J. Cox, The Perseus computational platform for comprehensive analysis of (prote)omics data, Nat. Methods, 2016, 13, 731–740 CrossRef CAS PubMed.
- M. Muzolf-Panek, A. Gliszczynska-Swiglo, H. Szymusiak and B. Tyrakowska, The influence of stereochemistry on the antioxidant properties of catechin epimers, Eur. Food Res. Technol., 2012, 235, 1001–1009 CrossRef CAS.
- N. Kitabatake and Y. I. Kinekawa, Digestibility of bovine milk whey protein and beta-lactoglobulin in vitro and in vivo, J. Agric. Food Chem., 1998, 46, 4917–4923 CrossRef CAS.
- M. R. Peram, S. M. Loveday, A. Ye and H. Singh, In vitro gastric digestion of heat-induced aggregates of beta-lactoglobulin, J. Dairy Sci., 2013, 96, 63–74 CrossRef CAS PubMed.
- H. F. J. Savelkoul, Immune parameters in high-risk atopic individuals during early childhood, Am. J. Respir. Crit. Care Med., 2000, 162, S100–S104 CrossRef CAS PubMed.
- M. B. G. Kiewiet, R. Dekkers, L. H. Ulfman, A. Groeneveld, P. de Vos and M. M. Faas, Immunomodulating protein aggregates in soy and whey hydrolysates and their resistance to digestion in an in vitro infant gastrointestinal model: new insights in the mechanism of immunomodulatory hydrolysates, Food Funct., 2018, 9, 604–613 RSC.
- S. D. Nielsen, R. L. Beverly and D. C. Dallas, Milk Proteins Are Predigested Within the Human Mammary Gland, J. Mammary Gland Biol., 2017, 22, 251–261 CrossRef PubMed.
- B. M. Prado, S. E. Sombers, B. Ismail and K. D. Hayes, Effect of heat treatment on the activity of inhibitors of plasmin and plasminogen activators in milk, Int. Dairy J., 2006, 16, 593–599 CrossRef CAS.
- B. M. Prado, B. Ismail, O. Ramos and K. D. Hayes, Thermal stability of plasminogen activators and plasminogen activation in heated milk, Int. Dairy J., 2007, 17, 1028–1033 CrossRef CAS.
- B. Manji, Y. Kakuda and D. R. Arnott, Effect of Storage Temperature on Age Gelation of Ultra-High Temperature Milk Processed by Direct and Indirect Heating Systems, J. Dairy Sci., 1986, 69, 2994–3001 CrossRef.
- H. E. Zenker, G. A. van Lieshout, M. P. van Gool, M. C. Bragt and K. A. Hettinga, Lysine blockage of milk proteins in infant formula impairs overall protein digestibility and peptide release, Food Funct., 2020, 11, 358–369 RSC.
- L. L. Baggio, E. M. Varin, J. A. Koehler, X. Cao, Y. Lokhnygina, S. R. Stevens, R. R. Holman and D. J. Drucker, Plasma levels of DPP4 activity and sDPP4 are dissociated from inflammation in mice and humans, Nat. Commun., 2020, 11, 3766 CrossRef PubMed.
- K. Tomovic, J. Lazarevic, G. Kocic, M. Deljanin-Ilic, M. Anderluh and A. Smelcerovic, Mechanisms and pathways of anti-inflammatory activity of DPP-4 inhibitors in cardiovascular and renal protection, Med. Res. Rev., 2019, 39, 404–422 CrossRef CAS PubMed.
- A. Gasparini, S. Buhler, A. Faccini, S. Sforza and T. Tedeschi, Thermally-Induced Lactosylation of Whey Proteins: Identification and Synthesis of Lactosylated beta-lactoglobulin Epitope, Molecules, 2020, 25, 1294 CrossRef CAS PubMed.
- B. Sheng, L. B. Larsen, T. T. Le and D. Zhao, Digestibility of Bovine Serum Albumin and Peptidomics of the Digests: Effect of Glycation Derived from alpha-Dicarbonyl Compounds, Molecules, 2018, 23, 712 CrossRef PubMed.
- D. A. Madalena, Ó. L. Ramos, R. N. Pereira, A. I. Bourbon, A. C. Pinheiro, F. X. Malcata, J. A. Teixeira and A. A. Vicente, In vitro digestion and stability assessment of β-lactoglobulin/riboflavin nanostructures, Food Hydrocolloids, 2016, 58, 89–97 CrossRef CAS.
|
This journal is © The Royal Society of Chemistry 2022 |
Click here to see how this site uses Cookies. View our privacy policy here.