DOI:
10.1039/D1FO01507A
(Paper)
Food Funct., 2022,
13, 270-279
Influence of extraction technology on rapeseed oil functional quality: a study on rapeseed polyphenols†
Received
14th May 2021
, Accepted 15th July 2021
First published on 10th December 2021
Abstract
Extraction technology can influence the vegetable oil functional quality. Polyphenols in rapeseed oil have been proved to be beneficial for cardiovascular health. In this study, we evaluated the effect of extraction methods on the functional quality of rapeseed oil from the perspective of phenolic compounds. The results showed that hot pressing produces the highest amount of phenolic compounds in rapeseed oil. Its most abundant phenolic compound, sinapine (9.18 μg g−1), showed the highest activity in inhibiting anaerobic choline metabolism with an EC50 value of 1.9 mM, whose downstream products are related to cardiovascular diseases. Molecular docking and molecular dynamics (MD) simulations revealed that sinapine exhibits good binding affinity toward CutC, and CutC–sinapine is a stable complex with fewer conformational fluctuations and similar tightness. Taken together, hot pressing can be considered the best extraction method for rapeseed oil from the perspective of phenolic compounds.
Introduction
Rapeseed is one of the most important oil crops, ranking as the second leading source of edible oil production in the world.1 Rapeseed oil contains a relatively high content of polyunsaturated fatty acids and natural biologically active compounds (tocopherols, phytosterols and polyphenols) with potential health benefits.2 Rapeseeds are rich in polyphenols, and their polyphenol content is 10 to 30 times higher than that in other oil seeds.3 Previous studies have found that polyphenols have various biological activities, such as anti-tumor, hypoglycemic, anti-pathogenic, and anti-inflammatory activities besides lowering blood lipids and preventing cardiovascular diseases (CVD).4 So, polyphenol content has been considered as a biomarker for oil quality.
However, during rapeseed oil processing, most of the polyphenols remain in the rapeseed meal, and only a small amount of the phenolic compounds is transferred to the oil.5 Conventionally, edible oil extraction is carried out by pressing (including hot pressing (HP) and cold pressing (CP)), the leaching process (LP) and aqueous enzymatic extraction (AEE).5 It was found that the extraction methods have an impact on the polyphenol compound types and amounts. CP oil preserves more bioactive compounds compared to HP oil and LP oil because it is produced without chemical and heat treatment.1 However, research suggests that heating the seeds at a suitable temperature before pressing can significantly increase the content of total polyphenols.6 For example, Szydlowska-Czerniak et al. found that rapeseed treatment at 90–120 °C for 30 min can increase the content of bioactive compounds (especially polyphenols).7 Although different extraction methods were used for rapeseed oil in studies,8,9 to date there is no research on the effect of extraction methods on cardiovascular protection by rapeseed polyphenols. So, in this study, these four extraction methods have been adopted for research on the effect of processing methods on the composition of rapeseed oil polyphenols based on cardiovascular protection.
Gut microbiota-dependent trimethylamine-N-oxide (TMAO) has been proposed as a novel and independent risk factor related to CVD.10,11 A decrease in the level of TMAO or its precursor substance trimethylamine (TMA) has been put forward as a new therapeutic target for CVD prevention and treatment. Polyphenols have been found to inhibit the production of TMA and TMAO.12–15 For example, resveratrol (RSV) could change the composition of the intestinal flora, increase the abundance of Lactobacillus and Bifidobacterium bacteria and thus reduce the circulating TMA and TMAO.12 Grape pomace polyphenolic extract also exerted the effect of reducing TMA and TMAO in human serum because of antioxidant and flora remodeling.13 However, there is no investigation on the effect of rapeseed polyphenols on TMA or TMAO production and the mechanism of how rapeseed polyphenols reduce TMA or TMAO.
In this study, liquid chromatography coupled with tandem mass spectrometry (LC-MS/MS) was used to determine the content of polyphenols in rapeseed oil extracted by different methods, and the effects of phenolic compounds on anaerobic choline metabolism were also tested in an in vitro bacterial study. In addition, we tried to explain the mechanism of rapeseed oil polyphenols inhibiting anaerobic choline metabolism using in silico docking and MD simulations. The findings of this research can provide guidance for the preparation of rapeseed oil for cardiovascular health, and also have reference significance for the development of high-quality rapeseed oil.
Materials and methods
Materials
Rapeseed samples were collected from the Oil Crops Research Institute, Chinese Academy of Agricultural Sciences (Wuhan, China). Standards of sinapic acid, sinapine, syringic acid, ferulic acid and salicylic acid were purchased from Meilunbio (Dalian, China); cinnamic acid, caffeic acid, gallic acid, p-hydroxybenzoic acid and vanillic acid were purchased from Yuanye Biotechnology Co., Ltd (Shanghai, China). Cellulase, pectinase, and alcalase 2.4 L were obtained from Novozymes (Beijing, China). A diol-solid phase extraction (SPE) column (200 mg, 3 mL) was purchased from ANPEL Laboratory Technology Inc (Shanghai, China).
Preparation of oil samples and compounds
CP and HP rapeseed oil were obtained by pressing the raw material through a screw press; the specific parameters were as follows: CP at room temperature, HP at 120 °C for 20 min and then pressing, then the crude oil was centrifuged at 5000 rpm for 10 min. Extraction by the leaching process (LP) was conducted as factory described: after crushing the rapeseed, n-hexane (with a material-to-liquid ratio of 1
:
3) was added and stirred overnight, and then the extract was filtered, and the solvent was removed by rotary evaporation. The preparation of rapeseed oil by the AEE method was performed according to our earlier research.9 Briefly, the ratio of rapeseed to water was 1
:
5 (w
:
v), under the conditions of 50 °C and pH 4.5, and 3% composite cell wall polysaccharide enzyme was added. Two hours later, the pH was adjusted to 9.0, and then 1.5% alkaline protease was added for 4 h. Finally, the enzyme was inactivated at 95 °C for 15 min, and after cooling, it was centrifugated at 4500 rpm for 10 min to obtain the oil.
The diol-SPE column was used to extract polyphenols from rapeseed oil. The extraction method was validated according to the instructions. The column was activated with methanol and n-hexane, respectively, and after loading the mixture of 3 g oil and 5 mL n-hexane into the column, the column was washed with 9 mL n-hexane, and finally eluted with 9 mL methanol.16 The eluent was collected and dried with N2, and finally re-dissolved in 2 mL of methanol. The total phenol content was estimated by the Folin–Ciocalteu method.17 Briefly, 1 mL of the extract polyphenols was mixed with 0.5 mL of the Folin–Ciocalteu reagent, 2 mL of 7.5% (w/v) Na2CO3, and 6.5 mL of water, followed by mixing the solution thoroughly on a vortex mixer for 1 min, and then incubating at 70 °C for 30 min for color development. The absorbance was measured at 750 nm using gallic acid as a standard, based on the concentration and absorbance of the gallic acid working solution to quantify the total phenols. For LC-MS/MS analysis, the solution was filtered through a 0.22 μm polytetrafluoroethylene (PTFE) membrane.
LC-MS/MS analysis
The LC-MS/MS method was employed to determine the polyphenol compounds.18 The separation chromatographic column was a reversed-phase Hypersil GOLD C18 (100 mm × 2.1 mm, 1.9 μm) (Thermo Scientific, USA). Elution conditions: phase A was 0.1% (v
:
v) acetic acid in water, and phase B was 0.1% (v
:
v) acetic acid in acetonitrile. The gradient elution process was: 0–0.5 min, 10% B; 0.5–15 min, 10–55% B; 15–15.5 min, 55–100% B; 15.5–17 min, 100% B; 17–17.5 min, 100–10% B; and 17.5–20 min, 10% B. Column temperature: 35 °C; flow rate: 0.3 mL min−1; injection volume: 2 μL. Compounds were identified and quantified by comparing their MS and peak area with the standard samples and standard curve. The mass spectrometry parameters and the verification of the LC-MS/MS method are shown in the ESI.†
TMA-reducing activity
We identified a series of rapeseed polyphenols, and these rapeseed polyphenols were tested in vitro for the inhibition of TMA production from anaerobic choline metabolism by human gut microbiota, Clostridium sporogenes ATCC 15579, which include CutC and CutD genes encoding proteins and can generate TMA.19C. sporogenes ATCC 15579 was incubated at 37 °C in a gut medium containing choline and polyphenols, and after 12 h, the TMA concentration was measured by LC-MS/MS.20
Molecular docking
In order to determine the interaction between choline TMA lyase CutC and rapeseed polyphenols, docking analysis was carried out. The SDFs (structure data files) of rapeseed polyphenol were obtained from the PubChem database (https://pubchem.ncbi.nlm.nih.gov) in SDF format. Then optimization of the lowest energy conformation and conversion of mol2 format files using the Chem 3D software at a MM2 level, for the preparation of the docking ligands, were carried out.
The crystal structure of CutC (PBD ID: 5FAU) was obtained from the PDB Protein Data Bank (http://www.rcsb.org).20 Then solvation parameters, essential hydrogen atoms and the Kollman united atom charges were added using AutoDock Tools.21 After correcting all those aspects, the binding affinities of polyphenols and CutC were calculated in the active site pocket with the center of the grid box being X: −37.750, Y: −11.750, and Z: 57.778, and the size of X, Y and Z being 112, 42, and 126 respectively. According to the docking affinity, combined with in vitro experiments, the polyphenols with the strongest TMA reduction ability were selected for further study. The results were visualized and rendered via VMD 1.9.3 version.22
Molecular dynamics (MD) simulations
The Groningen Machine for Chemical Simulations (GROMACS) package was used for MD simulations.23 The crystal structure of CutC (PBD ID: 5FAU) was selected for the simulation of protein and polyphenols. The AMBER 14SB24 and GAFF2 force field25 were selected in this system to model the interactions26 and all the systems were immersed in a rectangular box of water with a thickness of 10 Å. All the simulations were performed via the GROMACS package version 2019.5.27 The whole simulation process was as follows: firstly, the entire energy of the system was minimized using the steepest descent minimization of 5000 steps combined with a conjugate gradient minimization of 5000 steps to avoid unreasonable contact. Secondly, the NPT ensemble was used for pre-balancing of the system, and V-rescale temperature-coupling and the Parrinello–Rahman pressure coupling were used to maintain a constant temperature of 298 K and a pressure of 1 atm, respectively. The non-bonding cutoff radius was 1.0 nm, and the simulation time step was 2 fs. After balancing the system, the Berendsen method was employed to control the system temperature, and LINear Constraint Solver (LINCS) was used to constrain the bond length and bond angle. A cutoff equal to 1.0 nm was used for short-range electrostatics and van der Waals interactions, and the long-distance electrostatic interaction was created by the particle mesh Ewald method. The total simulation time was 30 ns, and the corresponding track files were saved every 10 ps. The root-mean-squared deviation (RMSD), root-mean-square fluctuation (RMSF), radius of gyration (Rg), and radial distribution function (RDF) were calculated from the MD simulations.26,28,29
Statistical analysis
All data were presented as mean ± standard error, the SPSS 23.0 software was used for one-way analysis of variance, and Dunnett's test was used for statistical significance (P < 0.05).
Results and discussion
The effect of extraction methods on total phenol content
The total content of phenols was measured using gallic acid as a standard, based on the standard curve equation: y = 0.05x + 0.0056 (Fig. 1A). The total phenol content of rapeseed oil prepared by HP, CP, LP, and AEE was analyzed, and the results showed that the content of total phenols in rapeseed oil obtained by HP was significantly higher than that by the other methods, reaching 64.63 μg g−1, indicating that polyphenols were relatively stable under 120 °C heating treatment of rapeseed (Fig. 1B). After 120 °C heating treatment, the content of total phenols in rapeseed oil increased. These results agreed with those in the work by Yang et al.,30 who reported that heating rapeseed increased the phenolic content.
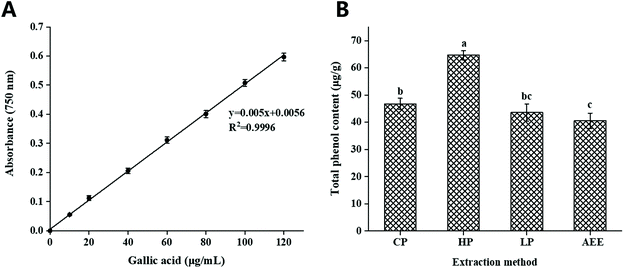 |
| Fig. 1 Total phenol content of rapeseed oils prepared by different extraction methods. (CP: cold pressing; HP: hot pressing; LP: leaching process; AEE: aqueous enzymatic extraction). (A) Standard curve for gallic acid; (B) total phenol content. | |
LC-MS/MS analysis for polyphenol compounds
The LC-MS/MS results of the scan mode, molecular weight, structural formula and retention time of the phenolic compounds are shown in Table 1S,† and the content of phenolic compounds in different rapeseed oils are summarized in Table 1. The method was validated for limit of detection (LOD), limit of quantitation (LOQ) (Table 2S†), recovery rate, accuracy, and precision (Table 3S†). In this study, a total of nine phenolic compounds were detected in HP rapeseed oil, including ferulic acid, sinapine, sinapic acid, syringic acid, cinnamic acid, vanillic acid, p-coumaric acid, gallic acid, and chlorogenic acid. However, caffeic acid, salicylic acid, and p-hydroxybenzoic acid were not detected. Sinapine and sinapic acid were the major phenolic compounds in these four oils, with the highest concentration of 9.18 μg g−1 and 7.69 μg g−1 in HP rapeseed oil, respectively. And these results were in agreement with a previous study (5.83–9.02 μg g−1). Interestingly, although the total content of phenols was similar, the sinapine content in AEE was higher than that of LP and CP treatments. However, the content of phenolic compounds in LP was lower than that in others because the rapeseed polyphenols were more polar than n-hexane, so they might be insoluble in n-hexane. In addition to polyphenol, erucic acid content should also be considered because it might cause harm to human health such as myocardial conduction disorders, lipidosis in children, and elevated blood cholesterol. Regulations have been announced to restrict the maximum level of erucic acid to 2–5% of the total fatty acids. Therefore, the amount of erucic acid should be considered during processing to avoid potential adverse health effects.
Table 1 Effects of extraction methods on the phenolic compounds of rapeseed oila
Compounds |
CP (ng g−1) |
HP (ng g−1) |
LP (ng g−1) |
AEE (ng g−1) |
CP: cold pressing; HP: hot pressing; LP: leaching process; AEE: aqueous enzymatic extraction.
|
Ferulic acid |
287.15 ± 23.66 |
220.41 ± 5.90 |
— |
6.17 ± 0.3 |
Caffeic acid |
— |
— |
— |
— |
Sinapine |
2610 ± 450 |
9180 ± 900 |
2870 ± 270 |
4270 ± 390 |
Sinapic acid |
7251.26 ± 327.52 |
7693.18 ± 651.81 |
105.97 ± 21.19 |
628.68 ± 35 |
Syringic acid |
284.66 ± 8.56 |
277.22 ± 9.06 |
— |
25.50 ± 0.94 |
Cinnamic acid |
496.53 ± 11.07 |
401.93 ± 17.87 |
105.97 ± 6.089 |
27.31 ± 1.23 |
Vanillic acid |
68.48 ± 7.12 |
49.75 ± 5.72 |
— |
— |
p-Coumaric acid |
9.56 ± 0.16 |
5.07 ± 1.72 |
— |
— |
Gallic acid |
26.65 ± 2.27 |
25.29 ± 0.94 |
25.06 ± 0.97 |
42.48 ± 0.94 |
Chlorogenic acid |
181.52 ± 3.55 |
182.60 ± 0.06 |
— |
— |
Salicylic acid |
— |
— |
— |
— |
p-Hydroxybenzoic acid |
— |
— |
— |
— |
TMA-reducing activity
We identified the major rapeseed polyphenols and tested the inhibitory effects of anaerobic choline metabolism using a human gut isolate C. sporogenes in vitro. The cultures were grown anaerobically in a medium containing choline and polyphenols. Results showed that sinapine emerged as a promising inhibitor (Fig. 2A), with an EC50 of 1.9 mM (Fig. 2B). However, we observed no inhibition with 3,3-dimethyl-1-butanol (DMB), a previously reported CutC inhibitor.31 This may be due to the inability of DMB to interact with the active site of CutC.20
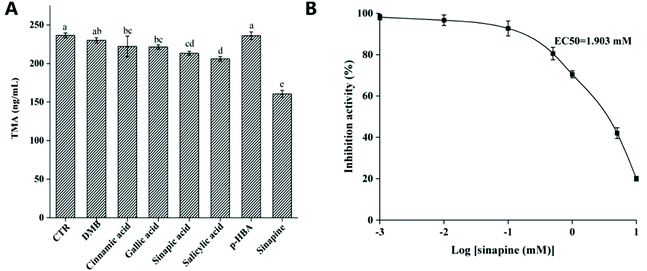 |
| Fig. 2 Rapeseed polyphenols inhibit TMA formation in C. sporogenes. (CTR: control; DMB: 3,3-dimethyl-1-butanol; p-HBA: p-hydroxybenzoic acid). (A) C. sporogenes were incubated in Mega medium with 1 mM choline and 1 mM polyphenol at 37 °C; (B) C. sporogenes were incubated in Mega medium with 0.1, 0.5, or 1 mM sinapine at 37 °C. | |
Molecular docking studies
Choline TMA lyase (CutC) catalyzes the deamination of choline to TMA, which is the main source of TMA in the human intestine. This microbial metabolite and its downstream products (TMAO) are directly related to CVD. CutD is an s-adenosine-methionine (SAM) activating enzyme that can activate CutC to form a glycyl radical.32,33 In CutC, the glycyl radical obtains a hydrogen from cysteine (Cys489) to generate sulfhydryl radicals, and further captures hydrogen atoms from the C1 position of choline, leading to molecular rearrangement to produce TMA and acetaldehyde.33 At the active site of CutC, choline orientation should cause its C1 hydroxyl group to form a hydrogen bond with Glu491. The primary substrate-specific contact is the CH–O hydrogen bond array between the polarized methyl trimethylammonium part and the oxygen atoms of the side chains of Tyr208, Asp216, and Tyr506, and bound water molecules.33
In this investigation, thirteen polyphenols from rapeseed oil that have been proved to have good physiological and biological activities in the prevention of CVD were selected for docking. 3,3-Dimethyl-1-butanol (DMB), which has been found to inhibit the activity of choline TMA lyase, was used as a positive inhibitor. As shown in Table 2, the binding energy of DMB and CutC was −4.0 kcal mol−1, which was the same as in a previous study.34 However, all these polyphenols exhibit better binding affinity (−5.0 to −5.8 kcal mol−1) toward CutC than DMB (Table 2).
Table 2 Structure and binding energy of rapeseed polyphenols with CutC
Name |
Structure |
Molecular weight |
Docking score (kcal mol−1) |
Ferulic acid |
|
194.18 |
−5.8 |
Caffeic acid |
|
180.16 |
−5.8 |
Sinapine |
|
310.369 |
−5.6 |
Sinapic acid |
|
224.21 |
−5.6 |
2-Hydroxy cinnamic acid |
|
164.16 |
−5.6 |
Syringic acid |
|
198.17 |
−5.5 |
Cinnamic acid |
|
148.16 |
−5.5 |
Vanillic acid |
|
168.149 |
−5.5 |
p-Coumaric acid |
|
164.16 |
−5.4 |
Gallic acid |
|
170.12 |
−5.3 |
3,4-Dihydroxybenzoic acid |
|
154.12 |
−5.2 |
Protocatechuic acid |
|
154.12 |
−5.1 |
p-Hydroxybenzoic acid |
|
138.12 |
−5.0 |
3,3-Dimethylbutanol |
|
102.17 |
−4 |
In particular, sinapine was found to have a relatively high affinity (−5.6 kcal mol−1) compared with other polyphenols, which indicated that it can be easily combined with CutC protease. Moreover, considering that sinapine is the most dominant polyphenolic compound in rapeseed oil (Table 1), and its binding affinity toward CutC was higher, from the perspective of economic and nutritional value, sinapine should be chosen as an inhibitor of TMA production. The results of molecular docking showed that sinapine can be combined with the CutC enzyme (Fig. 3A). The residue analysis of the interaction between sinapine and CutC enzyme showed that sinapine binds to the inside of the extremely hydrophobic pocket of the CutC enzyme (Fig. 3B), which includes the hydrophobic residues of Gly151, Phe158, Phe825, Phe826, Pro74, Pro154, Pro157, and Pro209. Sinapine has a hydrophobic aromatic ring, which will enhance the hydrophilicity of these residues. So sinapine could stably exist in the active pocket of the enzyme, thereby inhibiting the binding of choline to CutC.
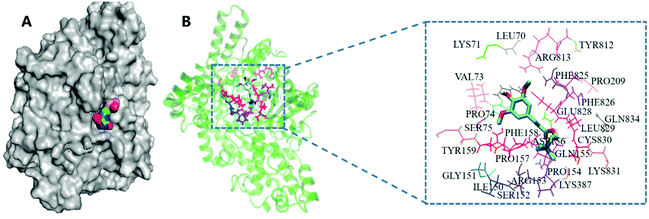 |
| Fig. 3 The binding structure and interaction between the residues of CutC and sinapine. (A) The overall binding structure of CutC and sinapine. (B) Interaction between the amino acid residues of CutC and sinapine. | |
Molecular dynamics (MD) simulations
As sinapine is the most dominant polyphenolic compound in rapeseed oil, and its binding affinity toward CutC was higher, sinapine was chosen as an inhibitor of anaerobic choline metabolism. In order to explore the complex of CutC–sinapine for a more accurate molecular insight, MD simulations were employed in this study. As shown in Fig. 4, the sinapines were randomly placed at the initial stage of the MD simulation and clustered to the surface of CutC, which was in agreement with the results of docking.
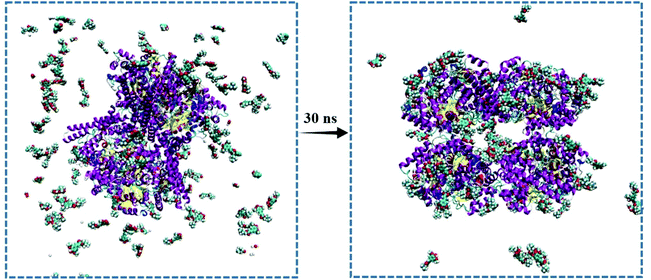 |
| Fig. 4 Molecular structure of CutC–sinapine before and after 30 nm simulation. | |
The RMSD, RMSF and Rg (Fig. 5) values of the protein backbone atoms were also analyzed to evaluate the stability and fluctuations of CutC during the simulation. The average values of RMSD were between 0.1 nm and 0.2 nm (Fig. 5A) for CutC, which indicated that the structure of CutC can remain stable, moreover, the RMSD value became relatively stable in 5 ns in both the CutC system and CutC–sinapine system, which indicated that 30 ns simulation is long enough for these two simulations. Furthermore, the RMSD value of CutC was significantly higher than that of the CutC–sinapine complex system, which indicated that the structure of CutC can be stabilized by sinapine. In addition, Nie et al. also obtained similar results, when they studied the molecular recognition mechanism between the GA molecule and Aβ1–40 monomer; through MD simulation, they found that the RMSD fluctuation of the Aβ1–40 monomer-GA complex was lower than that of the Aβ1–40 monomer.35
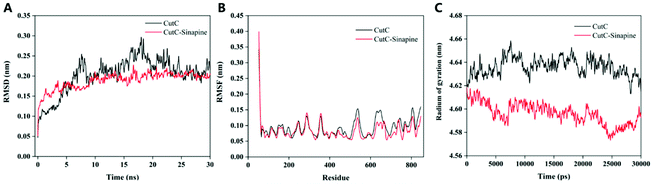 |
| Fig. 5 RMSD, RMSF and Rg as a function of simulation time for CutC in the absence and presence of sinapine. (A) RMSD; (B) RMSF; (C) Rg. | |
The RMSF is calculated to obtain the movement change of amino acids during the MD simulation process,35 where a higher RMSF value represents a more unstable conformation.36 As shown in Fig. 5B, compared with the N-terminal and C-terminal residues, both the normal residue RMSF values of CutC and the CutC–sinapine complex were relatively lower, suggesting that the flexibility of the N- and C-terminal residues was high. After combining with sinapine, the average RMSF value of certain amino acid residues in CutC was significantly lower than the value before combining. Specifically, the RMSF values of residues 500 to 580, 610 to 680 of CutC were significantly lower than those of the CutC–sinapine complex, indicating that these amino acid residues have lower flexibility. It may be because of the interaction of sinapine that restrained the wobbling of these residues.
The Rg values could be used to provide information on the tightness of the structure of the protein.37 As illustrated in Fig. 5C, the Rg of the CutC–sinapine complex started at relatively high values and then decreased to relatively low values during the MD simulations. Moreover, the relatively lower R(g) of the CutC–sinapine complex system also suggested that CutC will become tighter in the presence of sinapine. We proposed that it may be because of the aggregation of sinapine squeezed CutC, which made it become tighter.
RDF describes the variation of atom(s) density as a function of distance from a reference particle, and has been used to reveal the bonding and distribution of the inhibitor relative to the amino acid residues of the reference protein.38,39Fig. 6 represents the RDF values of the enzyme active sites with sinapine, at 0–10, 10–20, and 20–30 nanoseconds. The separation distance of the RDF value of the largest peak of Y208, F395, Y506 and G821 does remain unchanged, and the RDF value of 10–20 and 20–30 is higher than 0–10, which indicates that sinapine can exist stably near these sites. For the interaction of Y208–sinapine, the maximum peak of RDF appeared at 1.275 Å with a g(r) value of 12.85, indicating that there is 12.85 times greater probability that the distance between sinapine and Y208 is 1.275 Å. Similarly, there is a possibility that the distance of 1.98 Å between sinapine and F395 is 13.58, and there is a possibility that the distance of 1.82 Å between sinapine and G821 is 13.69. Overall, among all these interactions, sinapine and Y506 have the highest g(r) value (17.27). The higher g(r) value means that sinapine comes in close contact with the residues enhancing complex stability and strength.
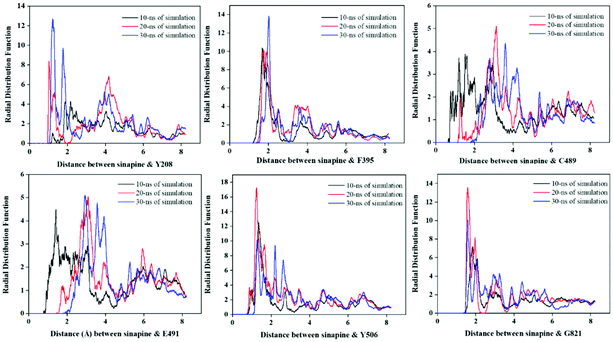 |
| Fig. 6 The radial distribution function of sinapine and enzyme active site residues of CutC. | |
Conclusions
As we all know, extraction technology can exert influences on rapeseed oil polyphenols, which have been proved to be beneficial for cardiovascular health. In this study, polyphenols in rapeseed oil from CP, HP, LP, and AEE were investigated, and the obtained LC-MS/MS results indicated that hot pressing produces the highest content of total phenols and the highest levels of sinapine and sinapic acid. In vitro assays showed that sinapine exhibits activities to inhibit anaerobic choline metabolism, suggesting that it is an inhibitor of TMA production. Both TMA and its downstream products (TMAO) are directly related to CVD. Therefore, TMA reduction is considered to have cardiovascular protective effects. Molecular docking results showed that rapeseed polyphenols exhibit good binding affinity toward CutC, and among them, sinapine has a relatively high affinity compared with other polyphenols, which indicated that it can be easily combined with CutC protease. Furthermore, MD simulation was performed on the complexes of CutC–sinapine. The obtained MD results showed that the CutC–sinapine complex behaves as a stable complex during the simulation. Based on the obtained results, we propose that sinapine is a potential candidate for inhibition of gut microbial TMA production. Among HP, CP, LP and AEE extraction methods for rapeseed oil, HP rapeseed oil has the highest sinapine content, so HP treatment can be considered the best method to extract rapeseed oil from the perspective of phenolic compounds. Certainly, unrefined oil also needs to be considered for the presence of erucic acid, because erucic acid has toxic effects on the heart at high enough doses, and regulations have been imposed to restrict the maximum level of erucic acid to 2–5% of the total fatty acids. Finally, further in vivo evaluations are needed to confirm and expand our findings.
Conflicts of interest
The authors declare no competing financial interest.
Acknowledgements
This work was supported by the Open Project Program of State Key Laboratory of Food Science and Technology, Jiangnan University (No. SKLF-ZZB-202113), Jiangsu distinguished professor project and Talent plan of Taihu Lake. All authors have read and approved the final manuscript.
References
- S. C. Chew, Cold-pressed rapeseed (Brassica napus) oil: chemistry and functionality, Food Res. Int., 2020, 131, 108997 CrossRef PubMed.
- A. Siger, M. Gawrysiak-Witulska and I. Bartkowiak-Broda, Antioxidant (tocopherol and canolol) content in rapeseed oil obtained from roasted yellow-seeded Brassica napus, J. Am. Oil Chem. Soc., 2017, 94, 37–46 CrossRef PubMed.
- Y. J. Xu, F. Jiang, J. Song, X. Yang, N. Shu, L. Yuan, C. P. Tan and Y. Liu, Understanding of the role of pretreatment methods on rapeseed oil from the perspective of phenolic compounds, J. Agric. Food Chem., 2020, 68, 8847–8854 CrossRef PubMed.
- Y. Li, J. Li, Q. Su and Y. Liu, Sinapine reduces non-alcoholic fatty liver disease in mice by modulating the composition of the gut microbiota, Food Funct., 2019, 10, 3637–3649 RSC.
- A. Siger, M. Józefiak and P. Górnaś, Cold-pressed and hot-pressed rapeseed oil: the effects of roasting and seed moisture on the antioxidant activity, canolol, and tocopherol level, Acta Sci. Pol. Technol. Aliment., 2017, 16, 69–81 Search PubMed.
- K. Kraljic, D. Skevin, M. Pospisil, M. Obranovic, S. Nederal and T. Bosolt, Quality of rapeseed oil produced by conditioning seeds at modest temperatures, J. Am. Oil Chem. Soc., 2013, 90, 589–599 CrossRef.
- A. Szydlowska-Czerniak, G. Karlovits, A. Sosna-Sardi, C. Dianoczki and E. Szlyk, Effect of hydrothermal treatment of rapeseed on antioxidant capacity of the pressed rapeseed oil, J. Am. Oil Chem. Soc., 2009, 86, 817–825 CrossRef.
- L. Tian, Y. Ren, R. Yang, Q. Zhao and W. Zhang, Combination of thermal pretreatment and alcohol-assisted aqueous processing for rapeseed oil extraction, J. Sci. Food Agric., 2019, 99, 3509–3516 CrossRef PubMed.
- Y. Li, L. Zhang, Y. J. Xu, J. Li, P. Cao and Y. Liu, Evaluation of the functional quality of rapeseed oil obtained by different extraction processes in a Sprague-Dawley rat model, Food Funct., 2019, 10, 6503–6516 RSC.
- W. H. Tang, Z. Wang, B. S. Levison, R. A. Koeth, E. B. Britt, X. Fu, Y. Wu and S. L. Hazen, Intestinal microbial metabolism of phosphatidylcholine and cardiovascular risk, N. Engl. J. Med., 2013, 368, 1575–1584 CrossRef PubMed.
- G. G. Schiattarella, A. Sannino, E. Toscano, G. Giugliano, G. Gargiulo, A. Franzone, B. Trimarco, G. Esposito and C. Perrino, Gut microbe-generated metabolite trimethylamine-N-oxide as cardiovascular risk biomarker: a systematic review and dose-response meta-analysis, Eur. Heart J., 2017, 38, 2948–2956 CrossRef PubMed.
- M. L. Chen, L. Yi, Y. Zhang, X. Zhou, L. Ran, J. Yang, J. D. Zhu, Q. Y. Zhang and M. T. Mi, Resveratrol attenuates trimethylamine-N-Oxide (TMAO)-induced atherosclerosis by regulating TMAO synthesis and bile acid metabolism via remodeling of the gut microbiota, MBio, 2016, 7, e02210–e02215 Search PubMed.
- G. Annunziata, M. Maisto, C. Schisano, R. Ciampaglia, V. Narciso, G. C. Tenore and E. Novellino, Effects of grape pomace polyphenolic extract (Taurisolo(R)) in reducing TMAO serum levels in humans: preliminary results from a randomized, placebo-controlled, cross-over study, Nutrients, 2019, 11, 139 CrossRef PubMed.
- L. Bresciani, M. Dall’Asta, C. Favari, L. Calani, D. D. Rio and F. Brighenti, An in vitro exploratory study of dietary strategies based on polyphenol-rich beverages, fruit juices and oils to control trimethylamine production in the colon, Food Funct., 2018, 9, 6470–6483 RSC.
- T. Lim, J. Ryu, K. Lee, S. Y. Park and K. T. H. Wang, Protective effects of black raspberry (Rubus occidentalis) extract against hypercholesterolemia and hepatic inflammation in rats fed high-fat and high-choline diets, Nutrients, 2020, 12, 2448 CrossRef PubMed.
- Y. Z. Yao, C. S. Liu, W. Xiong, Q. Liang, P. Xuan, X. D. Zeng, S. Q. Zeng, Q. Zhou and F. H. Huang, Silicon dioxide as an efficient adsorbent in the degumming of rapeseed oil, J. Clean. Prod., 2020, 268, 9 CrossRef.
- M. Koubaa, H. Mhemdi and E. Vorobiev, Influence of canola seed dehulling on the oil recovery by cold pressing and supercritical CO2 extraction, J. Food Eng., 2016, 182, 18–25 CrossRef.
- J. G. Song, C. Cao, J. Li, Y. J. Xu and Y. Liu, Development and validation of a QuEChERS-LC-MS/MS method for the analysis of phenolic compounds in rapeseed oil, J. Agric. Food Chem., 2019, 67, 4105–4112 CrossRef PubMed.
- K. A. Romano, E. I. Vivas, D. Amador-Noguez and F. E. Rey, Intestinal microbiota composition modulates choline bioavailability from diet and accumulation of the proatherogenic metabolite trimethylamine-N-oxide, MBio, 2015, 6, e02481 CrossRef PubMed.
- M. Orman, S. Bodea, M. A. Funk, A. M. Campo, M. Bollenbach, C. L. Drennan and E. P. Balskus, Structure-guided identification of a small molecule that inhibits anaerobic choline metabolism by human gut bacteria, J. Am. Chem. Soc., 2019, 141, 33–37 CrossRef PubMed.
- O. Trott and A. J. Olson, AutoDock Vina: improving the speed and accuracy of docking with a new scoring function, efficient optimization, and multithreading, J. Comput. Chem., 2010, 31, 455–461 Search PubMed.
- W. Humphrey, A. Dalke and K. Schulten, VMD: Visual molecular dynamics, J. Mol. Graph., 1996, 14, 33–38 CrossRef CAS PubMed.
- M. J. Abraham, T. Murtola, R. Schulz, S. Páll, J. C. Smith, B. Hess and E. Lindahl, GROMACS: High performance molecular simulations through multi-level parallelism from laptops to supercomputers, SoftwareX, 2015, 1–2, 19–25 CrossRef.
- J. A. Maier, C. Martinez, K. Kasavajhala, L. Wickstrom, K. E. Hauser and C. Simmerling, ff14SB: improving the accuracy of protein side chain and backbone parameters from ff99SB, J. Chem. Theory Comput., 2015, 11, 3696–3713 CrossRef CAS PubMed.
-
D. A. Case, R. M. Betz, D. S. Cerutti, T. E. Cheatham, T. A. Darden, R. E. Duke, T. J. Giese, H. Gohlke, A. W. Goetz, S. I. N. Homeyer, P. Janowski, J. Kaus, A. Kovalenko, T. S. Lee, S. LeGrand, P. Li, C. Lin, T. Luchko, R. Luo, B. Madej, D. Mermelstein, K. M. Merz, G. Monard, H. Nguyen, H. T. Nguyen, I. Omelyan, A. Onufriev, D. R. Roe, A. Roitberg, C. Sagui, C. L. Simmerling, W. M. Botello-Smith, J. Swails, R. C. Walker, J. Wang, R. M. Wolf, X. Wu, L. Xiao and P. A. Kollman, AMBER 2016, University of California, San Francisco, 2016 Search PubMed.
- J. Wang, R. M. Wolf, J. W. Caldwell, P. A. Kollman and D. A. Case, Development and testing of a general amber force field, J. Comput. Chem., 2004, 25, 1157–1174 CrossRef CAS PubMed.
- M. J. Abraham, T. Murtola, R. Schulz, S. Páll, J. C. Smith, B. Hess and E. Lindahl, GROMACS: high performance molecular simulations through multi-level parallelism from laptops to supercomputers, SoftwareX, 2015, 1–2, 19–25 CrossRef.
- J. Sharma, V. K. Bhardwaj, P. Das and R. Purohit, Identification of naturally originated molecules as γ-aminobutyric acid receptor antagonist, J. Biomol.
Struct. Dyn., 2021, 39, 911–922 CrossRef PubMed.
- R. Singh, V. Bhardwaj and R. Purohit, Identification of a novel binding mechanism of Quinoline based molecules with lactate dehydrogenase of Plasmodium falciparum, J. Biomol. Struct. Dyn., 2021, 39, 348–356 CrossRef PubMed.
- M. Yang, C. Zheng, Q. Zhou, C. Liu, W. Li and F. Huang, Influence of microwaves treatment of rapeseed on phenolic compounds and canolol content, J. Agric. Food Chem., 2014, 62, 1956–1963 CrossRef PubMed.
- Z. Wang, A. B. Roberts, J. A. Buffa, B. S. Levison, W. Zhu, E. Org, X. Gu, Y. Huang, M. Zamanian-Daryoush, M. K. Culley, A. J. DiDonato, X. Fu, J. E. Hazen, D. Krajcik, J. A. DiDonato, A. J. Lusis and S. L. Hazen, Non-lethal inhibition of gut microbial trimethylamine production for the treatment of atherosclerosis, Cell, 2015, 163, 1585–1595 CrossRef PubMed.
- C. Smaranda and E. P. Balskus, Microbial conversion of choline to trimethylamine requires a glycyl radical enzyme, Proc. Natl. Acad. Sci. U. S. A., 2012, 109, 21307–21312 CrossRef PubMed.
- S. Bodea, M. A. Funk, E. P. Balskus and C. L. Drennan, Molecular basis of C-N bond cleavage by the glycyl radical enzyme choline trimethylamine-lyase, Cell Chem. Biol., 2016, 23, 1206–1216 CrossRef PubMed.
-
Z. Apte, J. Richman, D. Almonacid, V. Marquez, I. Araya, M. Alegria, M. Saavedra, L. Gomez, J. Espinoza, J. Gimpel, E. Morales and R. Ortiz, Rieske-type oxygenase/reductase targeted drugs for diagnostic and treatment of diseases, 2019 Search PubMed.
- R. Z. Nie, Y. Q. Huo, B. Yu, C. J. Liu, R. Zhou, H. H. Bao and S. W. Tang, Molecular insights into the inhibitory mechanisms of gallate moiety on the Aβ1-40 amyloid aggregation: a molecular dynamics simulation study, Int. J. Biol. Macromol., 2020, 156, 40–50 CrossRef CAS PubMed.
- H. Zhang, J. Sang, Y. Zhang, T. Sun, H. Liu, R. Yue, J. Zhang, H. Wang, Y. Dai, F. Lu and F. Liu, Rational design of a Yarrowia lipolytica derived lipase for improved thermostability, Int. J. Biol. Macromol., 2019, 137, 1190–1198 CrossRef CAS PubMed.
- S. T. Ngo, X. C. Luu, N. T. Nguyen, V. V. Vu and H. T. T. Phung, Etersalate prevents the formations of 6Aβ16-22 oligomer: an in silico study, PLoS One, 2018, 13, e0204026 CrossRef PubMed.
- H. Ahmad, F. Ahmad, S. Parveen, S. Ahmad, S. S. Azam and A. Hassan, A combine approach of chemical synthesis, biological evaluation and structural dynamics studies revealed thiazole substituted arylamine derivatives as potent FabH enzyme inhibitors, Bioorg. Chem., 2020, 105, 104426 CrossRef CAS PubMed.
- S. Ahmad, S. Raza, S. W. Abbasi and S. S. Azam, Identification of natural inhibitors against Acinetobacter baumannii D-alanine-D-alanine ligase enzyme: a multi-spectrum in silico approach, J. Mol. Liq., 2018, 262, 460–475 CrossRef CAS.
Footnote |
† Electronic supplementary information (ESI) available. See DOI: 10.1039/d1fo01507a |
|
This journal is © The Royal Society of Chemistry 2022 |
Click here to see how this site uses Cookies. View our privacy policy here.