DOI:
10.1039/D1FO01484F
(Paper)
Food Funct., 2022,
13, 316-326
Tannin extract from maritime pine bark exhibits anticancer properties by targeting the epigenetic UHRF1/DNMT1 tandem leading to the re-expression of TP73†
Received
12th May 2021
, Accepted 1st November 2021
First published on 18th November 2021
Abstract
Maritime pine bark is a rich source of polyphenolic compounds and is commonly employed as a herbal supplement worldwide. This study was designed to check the potential of maritime pine tannin extract (MPTE) in anticancer therapy and to determine the underlying mechanism of action. Our results showed that MPTE, containing procyanidin oligomers and lanostane type terpenoids, has an inhibitory effect on cancer cell proliferation through cell cycle arrest in the G2/M phase. Treatment with MPTE also induced apoptosis in a concentration-dependent manner in human cancer cell lines (HeLa and U2OS), as evidenced by the enhanced activation of caspase 3 and the cleavage of PARP along with the downregulation of the antiapoptotic protein Bcl-2. Interestingly, human non-cancerous fibroblasts are much less sensitive to MPTE, suggesting that it preferentially targets cancer cells. MPTE played a pro-oxidant role in cancer cells and promoted the expression of the p73 tumor suppressor gene in p53-deficient cells. It also downregulated the protooncogenic proteins UHRF1 and DNMT1, mediators of the DNA methylation machinery, and reduced the global methylation levels in HeLa cells. Overall, our results show that maritime pine tannin extract can play a favorable role in cancer treatment, and can be further explored by the pharmaceutical industry.
1. Introduction
Cancer related diseases are among the major causes of death around the world. Though modern therapies have improved patient care and therapeutic outcomes, the majority of tumors are still untreatable.1,2 Chemotherapy is one of the most widely used treatments to cure cancer, although one of its frequently encountered disadvantages is cancer recurrence associated with drug resistance. Nature has demonstrated that it is the best source of anticancer drugs, but the grail has not yet been discovered as severe side effects and relapses undermine quality of life during and after treatment.3 Naturally occurring compounds from plants are being thoroughly explored for this purpose and many drugs of natural origin have entered clinical use.4 Indeed, some of the most effective anticancer drugs such as vincristine, vinblastine, docetaxel, paclitaxel are derivatives of the plant kingdom and are in clinical use today for treating diverse types of cancers.5 Treatment with these anticancer drugs inhibits the proliferation of tumors by halting cell cycle and inducing apoptosis.6,7 All these drugs were first tested as an extract and later purified.
The Pinus pinaster tree (synonym Pinus maritimus, maritime pine) is well-known in traditional herbal medicine for its multiple biological activities. Maritime pine trees are commonly found in Mediterranean countries such as France, Italy, Spain and Portugal, and in some northern African countries including Tunisia, Algeria and Morocco.8 Its bark is rich in polyphenolic compounds and is believed to have anti-inflammatory, antioxidant, antidiabetic, anticancer and antiallergic properties.9 The dry extract from the bark is available commercially (Pycnogenol®) and is commonly indicated for multiple diseases including asthma, allergies, skin diseases, diabetes, osteoarthritis, erectile dysfunction and venous disease.10 The polyphenolic constituents of this extract are divided into monomers or condensed (procyanidin) flavonoids. Monomers are generally catechin, epicatechin, epigallocatechin and epicatechin gallate along with small proportions of fisetinidin and taxifolin while the procyanidins are polymers of the flavan-3-ol units of (+)-catechin or epicatechin of various lengths.8,11,12 Similar constituents are also present in the barks of other Pinus species including Pinus massoniana, Pinus strobus and Pinus radiata which are native to Southeast Asia (particularly southern China), North America and New Zealand, respectively.13–15Pinus massoniana bark extract (PMBE), Pinus strobus bark extract and Pinus radiata bark extract (PRE also called Enzogenol) are also well known to possess cardio-cerebrovascular protective, and antitumor, anti-inflammatory and antimicrobial properties.13,15–17 Besides polyphenolic compounds, carbohydrates, terpenoids and some other phytochemical compounds have also been identified in the bark of Pinus trees.18–20
The anticancer activities of the French maritime pine bark have been outlined in a few studies as due to its polyphenolic content. It induces apoptosis in breast cancer cells, and leukemic and fibrosarcoma cells and also prevents the oncogenic transformation of ovarian cells on exposure to carcinogenic talc.21–24 Treatment with bark extract also lowers the incidence of side effects related to anticancer therapy.25 Despite the beneficial role of maritime pine bark in cancer suggested by these studies, the pathways involved in this beneficial effect remain elusive. The UHRF1/DNMT1 tandem is known to play an important role in the transmission of the cancer signature from a mother cell to the daughter cells.26–28 One of these signatures includes the silencing of the tumor suppressor genes.27–30
Here, we characterized the anticancer activity of the tannin extract from the bark of maritime pine and investigated whether the UHRF1/DNMT1 tandem could be a central target to explain the re-expression of tumor suppressor genes such as TP73. The polyphenolic compounds and lanosterol based compound present in this bark inhibit the proliferation of cancer cells by inducing arrest in the G2/M phase of the cell cycle. MPTE treatment also activates the p73 tumor suppressor gene and the apoptotic pathway in HeLa cells. This extract also downregulates the levels of epigenetic proteins like UHRF1 and DNMT1 involved in the maintenance of DNA methylation, ultimately leading to a global hypomethylation of the treated cells.
2. Materials and methods
2.1. Maritime pine tannin extract preparation
Maritime pine (Pinus maritimus) bark was obtained from “Les Landes”, a region located in the southwest of France. It was dried initially and crushed mildly to obtain coarse chips of bark which were later completely dried till a constant weight was obtained. Tannins were extracted from the dried ground bark by completely immersing it in 2% sodium bisulphite and 0.5% sodium bicarbonate water solution with continuous stirring in an industrial reactor (Biolandes, France). The final solution was spray-dried to obtain tannins in the form of a dark reddish-brown powder which was later used for studies.
2.2. Chemical characterization
The extract was analyzed by NMR spectroscopy and HPLC/MS techniques for chemical characterization. For NMR spectroscopy, the extract solutions were prepared using DMSO-d6 and D2O as solvents and measured on a Bruker 500 MHz NMR spectrometer equipped with a BBO 5 mm probe head with a Z-gradient. The extract was also analyzed using an Agilent 6520 mass spectrometer coupled with an Agilent 1200 series HPLC equipped with a Zorbax C18-SB column. The system was run using acetonitrile + 0.05% formic acid/H2O + 0.05% formic acid with a linear gradient. The analysis was run both in the ESI positive and negative modes of ionization.
2.3. Cell culture and MPTE treatment
HeLa cells (ATCC, CCL-2), U2OS and human fibroblasts were cultured in DMEM (Dulbecco's Modified Eagle's Medium) supplemented with 10% FBS (fetal bovine serum), in addition to penicillin (100 U ml−1) and streptomycin (100 U ml−1) (Invitrogen). Cells were maintained at 37 °C in a humid atmosphere with a continuous supply of CO2 maintained at 5%.31–33 The MPTE solution was always freshly prepared for the treatment of cells. 10 mg of the extract powder was first mixed with 50 μL of DMSO by sonication, and then this solution was diluted in 10 mL of preheated DMEM by brief vortexing to obtain a concentration of 1 mg mL−1. The extract solution was then sterilized by passing through Millex-GP, 0.22 μm syringe filters (Merck-Millipore) and diluted to the required concentrations with additional DMEM. The prepared solutions of the desired concentration were then added to seeded cells while the controls were incubated with fresh medium, without the addition of the extract.
2.4. Antibodies and chemicals
Different antibodies used in this study include mouse monoclonal anti-PARP (BD Biosciences Pharmingen), rabbit polyclonal anti-caspase3 (Cell Signaling), mouse monoclonal anti-UHRF1 engineered as described previously,34 mouse monoclonal anti-DNMT1 (Proteogenix France), mouse monoclonal anti-BCL-2 (Merck-Millipore), mouse monoclonal anti-GAPDH (Merck-Millipore), polyclonal anti-mouse (Promega) and polyclonal anti-rabbit (Promega) antibodies.
2.5. Cellular proliferation test
The effect of MPTE treatment on cellular proliferation was evaluated by using the colorimetric MTT assay. In this assay, viable cells are identified by their ability to reduce the tetrazolium dye MTT 3-(4,5-dimethylthiazol-2-yl)-2,5-diphenyltetrazolium bromide to form insoluble purple colored formazan crystals. These crystals are later dissolved in DMSO and quantified by measuring light absorption at 570 nm. Cells were seeded in a 96 well plate at a density of 5 × 103 cells per well and incubated with different concentrations of the MPTE extract. For negative controls, the medium was replaced with fresh medium without MPTE. After 24 h of treatment, the medium was replaced with 100 μL of the MTT (5 mg/10 mL) containing medium in each well and incubated for further 4 h. The formazan crystals formed after incubation with MTT were then dissolved in 100 μL of DMSO and the absorption at 570 nm was determined by using a Xenius plate reader. The percentages of viable cells in the treated samples were calculated with reference to untreated samples. The inhibition of proliferation was expressed as IC50, which is the concentration required to reduce the absorbance of the treated cells by 50% with reference to the control (untreated cells). The average IC50 values were statistically determined from the dose–response curves obtained using the Origin software (version 8.6).
2.6. Cell cycle and apoptosis analysis
For cell cycle analysis, HeLa cells were seeded in 6-well plates at a density of 105 cells per well and were treated with 75, 150 and 300 μg mL−1 of MPTE along with the control samples. After 24 h of treatment, the cells were washed with PBS and mildly trypsinized. The collected cells were then fixed in the BD CellFIX (BD Biosciences) reagent and further incubated with the FxCycle™ PI/RNase staining solution (Thermo Fisher Scientific) for 20 min before analysis using the Guava 12HT capillary cytometer with the easyCyte™ software (Merck-Millipore). Fractions of cells in different phases of the cell cycle were quantified by using the InCyte Software for Guava® (Merck-Millipore). For apoptosis analysis, cells were seeded and treated as mentioned above. Cells were collected and incubated with PI and annexin V-FITC™ (Miltenyi Biotec) for 20 min to label cells undergoing apoptosis. Cells were then analyzed using the Guava capillary cytometer. The percentage of cells in different phases of apoptosis was obtained by using the easyCyte™ software (Merck-Millipore).
2.7. Analysis of reactive oxygen species (ROS) production
HeLa cells were seeded and treated in 6-well plates, as described in the previous section. ROS production was determined by flow cytometry using dihydroethidium (DHE) staining. Cells were incubated with 10 μM of DHE for 30 min at 37 °C before collection and analyzed using the Guava 12HT cytometer (Merck-Millipore). PEG-catalase (Sigma-Aldrich) and N-acetylcysteine (NAC, Sigma-Aldrich) were added 1 h before MPTE.
2.8. Western blotting
HeLa cells were seeded in a 6-well plate and treated with 75, 150 and 300 μg mL−1 of MPTE for 24 h, as described earlier. After treatment, the cells were collected by trypsinization and incubated with lysis buffer, 10 mM Tris-HCl pH 7.5, 150 mM NaCl, 1 mM EDTA and 1% NP40 supplemented with protease inhibitors (complete mini EDTA free protease inhibitor cocktail tablets, Roche, Germany), for 30 min on ice. Forty (40) μg of the total protein lysate from each sample was resolved on 10% SDS-PAGE and transferred onto PVDF membranes. The membranes were blocked for 1 h with 3% blotting-grade blocker (Bio-Rad) in TBST buffer before incubating them overnight with primary antibodies at 4 °C. The membranes were then washed with TBST three times and incubated with secondary antibodies for 1 h at room temperature. After washing the membranes with TBST, they were imaged with the chemiluminescent ECL system (ClarityTM ECL western blotting substrate, Biorad, 170-5060) using the ImageQuant™ LAS 4000 system (GE Healthcare). Images were quantified using Image Studio Lite (LiCore Biosciences, USA).
2.9. Global methylation assay
For the global methylation assay, HeLa cells were seeded in six well plates and treated as described above. DNA was extracted from the treated and non-treated samples by using the QIAamp® DNA Kit (Qiagen). 200 μg of the purified DNA from each sample was then analyzed for global methylation levels by using Sigma's Imprint® methylated DNA quantification kit (Sigma-Aldrich), according to the manufacturer's protocol.
2.10. Statistical analysis
All experiments were repeated three times and the results between groups were statistically compared by one-way ANOVA with the post-hoc Tukey test using the GraphPad-Prism (version 5.04) and Origin (version 8.6) software.
3. Results
3.1. Chemical characterization of MPTE
Maritime pine tannin extract from the bark of pine trees has been well-characterized previously by MALDI-TOF and 13C NMR techniques, which are useful techniques for the identification of polyflavonoid tannin compounds and their oligomers. MPTE was reported to contain procyanidin oligomers formed by catechin/epicatechin, epigallocatechin and epicatechin gallate monomers (with up to 20 to 21 repeating units) as well as small amounts of fisetinidine and carbohydrates.11 However, MALDI-MS is not suitable to detect masses smaller than m/z 500 because of the interference of the matrix peaks. Therefore, we also aimed to identify compounds with smaller masses through NMR spectroscopy and HPLC/MS techniques.
The NMR analysis of MPTE solutions in D2O and DMSO-d6 revealed very complex spectra, indicating the presence of several compounds in the extract. In the D2O based solution, the chemical shifts are compatible with carbohydrate compounds (presence of many peaks in the 3–4 ppm range compatible with CH(OH) protons), while in the DMSO based MPTE solution, the presence of signals at a higher frequency can be in line with the presence of procyanidins, as already reported in maritime pine.11 For the HPLC/MS analysis, the extract was solubilized at 1 mg mL−1 in H2O/DMSO 95
:
5, which created a blind spot for very polar compounds such as catechins or sugars. While no compound could be detected in the ESI+ modus with significant intensity, two co-eluting compounds (m/z 457.4 and m/z 385.3) could be detected in the ESI− modus, at a retention time tR = 10.458 min (ESI Table 1 and Fig. 1†).
The selective fragmentation of the m/z 457 peak led to the formation of the [m/z 385
:
m/z 367] product couple. Increasing the fragmentation voltage stepwise led to the complete disappearance of the parent peak, and the increase of the [m/z 385
:
m/z 367] and [m/z 313
:
m/z 295] parent peaks derivative peaks. A possible explanation for the difference of 18 within each pair is provided by the loss of H2O, such that these compounds probably contain at least 2 hydroxyl groups. This also tends to indicate that the two ESI-detected compounds probably share the same core motif (ESI Fig. 2†).
A search in the Dictionary of Natural Products within the Pinus genre shows only one hit for an exact mass between 458 and 459: the lanost-9(11)-ene-3,24,25-triol, with the chemical formula C30H50O3.
3.2. Effect of maritime pine tannin extract (MPTE) on cell proliferation
First, the MPTE effect on cellular proliferation was determined by MTT assay on different cell lines including the cervical cancer HeLa cells, osteosarcoma U2OS cells, and normal fibroblast cells. Cells were treated with 12.5, 25, 50, 75, 100, 150, 300, 400 and 500 μg mL−1 of the extract for 24 h and the inhibitory effect was determined by comparing the number of viable cells with the untreated cells. The results of the MTT assay showed that MPTE inhibited cell proliferation in a dose dependent manner (Fig. 1). The IC50 values were graphically determined for each cell line, and it was observed that MPTE reduced the proliferation of HeLa and U2OS cells at a relatively low concentration (Fig. 1A and B) as compared to normal fibroblast cells (Fig. 1C). The IC50 values of the HeLa and U2OS cells were 150 ± 20 μg mL−1 and 220 ± 10 μg mL−1, respectively. For normal fibroblast cells, the IC50 value was 490 ± 30 μg mL−1, showing the selective response of MPTE towards cancer cells, which prompted us to analyze the mechanism of action of MPTE in HeLa cells.
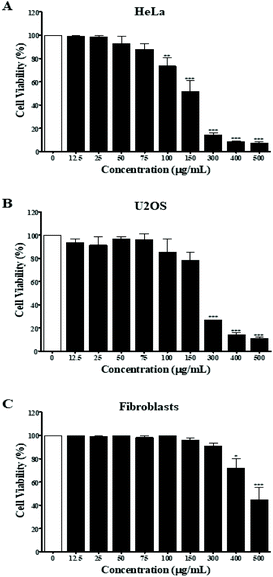 |
| Fig. 1 Inhibition of cell proliferation by MPTE. HeLa (A), U2OS (B) and fibroblast cells (C) were treated with MPTE for 24 h and the inhibition of proliferation was determined by colorimetric MTT assay. Values are represented as a percentage of the control, i.e., in the absence of MPTE. Values are means ± SEM of three independent experiments. Statistical significance is represented as *P < 0.05, **P < 0.01, and ***P < 0.001 versus control. | |
3.3. MPTE induces cell cycle arrest in the G2/M phase
In order to further explore the inhibitory effect of MPTE on cell proliferation, we analyzed the effect of 75, 150 and 300 μg mL−1 of MPTE on different phases of the cell cycle. Cytometric analysis revealed that treatment with MPTE for 24 h reduced the cell population in the G0/G1 phase while the population in the G2/M phase was increased in a dose dependent manner (Fig. 2). Indeed, the cell fraction in the G0/G1 phase was reduced from 57% in control cells to 30% and 27% in cells treated with 150 and 300 μg mL−1 of MPTE, respectively. Conversely, the cell population in the G2/M phase increased from 18% in control cells to 38% and 34% in HeLa cells treated with 150 and 300 μg mL−1 of MPTE, respectively. This shows that MPTE inhibits the proliferation of cells by inducing cell cycle arrest at the G2/M phase.
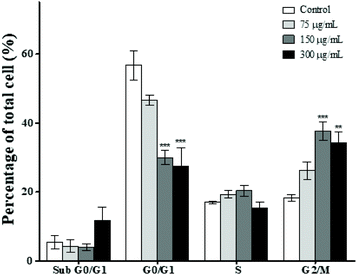 |
| Fig. 2 Cell cycle arrest induced by MPTE treatment. HeLa cells were treated with the indicated concentrations of MPTE for 24 h and the distribution of cells in different phases of the cell cycle was determined by cytometric analysis. The cellular distribution in each phase was represented in terms of percentage relative to the total cell number. Values are expressed as means ± SEM of three independent experiments. Statistical significance is represented as *P < 0.05, **P < 0.01, and ***P < 0.001 versus control. | |
3.4. MPTE treatment induces apoptosis in cells
The induction of apoptosis by MPTE treatment was investigated by cytometry using annexin-FITC and propidium iodide labelling. Treatment with MPTE decreased the number of viable cells and induced apoptosis in HeLa cells in a concentration dependent manner (Fig. 3A). Indeed, viable cells were reduced from 92% in control cells to 76%, 48% and 27% in cells treated with 75, 150 and 300 μg mL−1 of MPTE, respectively. In parallel, early apoptotic cells were increased from 1.8% in control to 8%, 8.7% and 5.2% in cells treated with 75, 150 and 300 μg mL−1 of MPTE, respectively (Fig. 3A). The percentage of late apoptotic cells and necrotic cells also increased significantly with 150 and 300 μg mL−1 of MPTE, showing the ability of this extract to induce apoptosis (Fig. 3A).
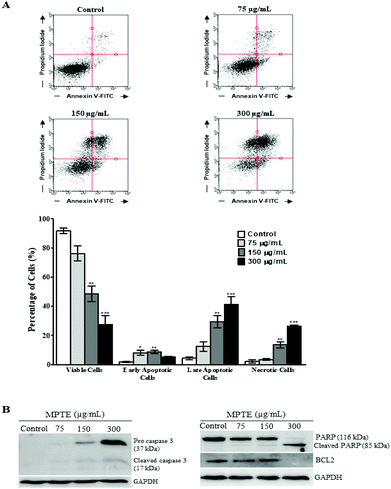 |
| Fig. 3 Apoptosis induced by MPTE treatment in HeLa cells. (A) HeLa cells were incubated with the indicated concentrations of MPTE for 24 h. Viable cells along with fraction of cells undergoing apoptosis were determined by annexin V-FITC and PI labelling through cytometry. Values are expressed as means ± SEM of three independent experiments. Statistical significance is represented as *P < 0.05, **P < 0.01, and ***P < 0.001 versus control. (B) Western blotting showing the cleavage of procaspase 3 and PARP along with the downregulation of the Bcl-2 protein in MPTE-treated HeLa cells. | |
We confirmed the induction of apoptosis by analysing the activation of caspase 3 and the cleavage of PARP as well as Bcl-2 downregulation in MPTE treated cells (Fig. 3B). Western blotting analysis revealed the activation of caspase 3 from its precursor protein after the cells were treated with ≥150 μg mL−1 of MPTE. PARP cleavage was also observed in response to MPTE treatment and became more prominent at a higher concentration of MPTE. Finally, Bcl-2 levels were found to decrease with MPTE treatment. These results confirmed the induction of apoptosis in response to MPTE treatment.
3.5. MPTE treatment induces ROS generation
In order to further determine the mechanism of apoptosis, we checked by cytometric analysis the levels of the reactive oxygen species (ROS) generated in cells treated with MPTE by using dihydroethidium (DHE) staining. DHE gets oxidized inside cells upon exposure to ROS and changes to 2-hydroxyethidium or ethidium which gets incorporated into the DNA and fluorescently labels the cells. The cytometric analysis of HeLa cells treated with MPTE for 24 h revealed significant production of ROS when they were incubated with the highest concentrations of MPTE (Fig. 4A and B). Indeed, the ROS levels increased by 1.5 and 2 fold upon 24 h treatment with 150 and 300 μg mL−1 of MPTE, respectively, as compared to the controls (Fig. 4B). The cell granularity is usually increased with ROS generation and is often considered as an indicator of senescence or apoptosis.35 Side scatter plots revealed that cell granularity increased after 24 h of treatment with 150 and 300 μg mL−1 of MPTE, in a pattern similar to the increase in ROS levels, suggesting that the high ROS levels caused by MPTE treatment induce apoptosis in these cells (Fig. 4C and D). The generation of the ROS induced by 75 μg mL−1 and 150 μg mL−1 of MPTE, assessed by determining DHE-positive cells, was significantly inhibited by the antioxidants NAC and PEG-catalase (Fig. 5A and B). However, at 300 μg mL−1 of MPTE, the inhibition was not evidenced, probably because the generated ROS concentrations became too high over a period of 24 h. These results suggest that the formation of H2O2 plays a fundamental role in conferring an anticancer activity to MPTE.
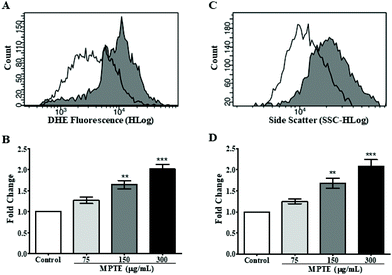 |
| Fig. 4 Effect of MPTE treatment on ROS levels and granularity in treated cells. (A) Cytogram showing DHE labelling in the control (represented by a white area under the graph) and MPTE-treated cells (300 μg mL−1, represented by a gray area under the graph). (B) Bar graph showing the fold change in DHE fluorescence by MPTE treatment at different concentrations compared to the control. (C) Cytogram showing side scatter in the control (represented by a white area under the graph) and MPTE-treated cells (300 μg mL−1, represented by a gray area under the graph). (D) Bar graph showing fold change in the side scatter by MPTE treatment at different concentrations with respect to the control. Values were obtained from three independent experiments and analyzed by one-way ANOVA with the post-hoc Tukey test (*P < 0.05, **P < 0.01, ***P < 0.001). | |
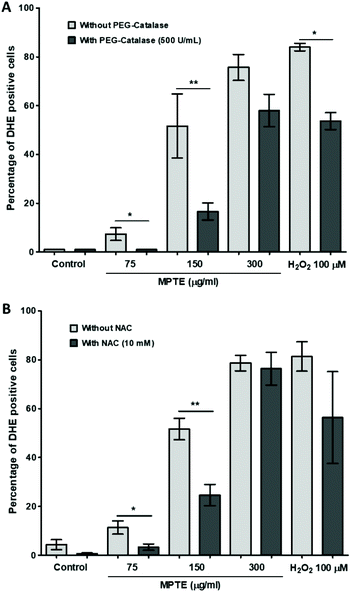 |
| Fig. 5 Effect of PEG-catalase (A) and alpha NAC (B) on ROS production induced by MPTE. PEG-catalase (500 U mL−1) and NAC (10 mM) were added 1 h prior to MPTE incubation and analysis of ROS production was performed 24 h later, as described in “Materials and Methods”. Values were obtained from three independent experiments and were analyzed by one-way ANOVA with the post-hoc Tukey test (*P < 0.05, **P < 0.01, ***P < 0.001). | |
3.6. MPTE treatment upregulates p73 and downregulates UHRF1 and DNMT1 in HeLa cells
Previously, it has been reported that naturally occurring polyphenolic compounds inhibit cell proliferation and activate apoptosis in p53-deficient cancer cells by inducing the expression of the analogue p73.36,37 To check this effect as a possible mechanism for the induction of cell cycle arrest and apoptosis, we evaluated the effect of MPTE treatment on the expression of p73 in these cells. HeLa cells were treated with 75, 150 and 300 μg mL−1 MPTE for 24 h. Western blotting data of the proteins isolated from these cells revealed that MPTE treatment induced the upregulation of p73 in HeLa cells (Fig. 6A and B). The increase in p73 expression was found to be the highest with 300 μg mL−1 of MPTE which led to a fivefold upregulation in p73 levels as compared to controls (Fig. 6B).
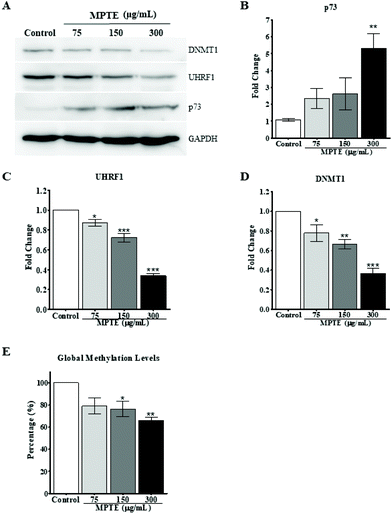 |
| Fig. 6 MPTE upregulates p73 along with the downregulation of UHRF1 and DNMT1. (A) Western blotting analysis of proteins isolated from HeLa cells treated with 75, 150 and 300 μg mL−1 MPTE for 24 h along with the untreated cells. (B) Effect of MPTE treatment on p73 levels versus controls. (C) Effect of MPTE treatment on UHRF1 levels with respect to controls. (D) Effect of MPTE treatment on DNMT1 levels versus controls. (E) Effect of MPTE treatment on global methylation levels. Values are obtained from three independent experiments and were analyzed by one-way ANOVA with the post-hoc Tukey test (*P < 0.05, **P < 0.01, ***P < 0.001). | |
In our previous studies, we have shown that p73 is under the regulation of the UHRF1/DNMT1 tandem.36,37 Thus, we hypothesized that the MPTE-induced up-regulation of p73 could be the result of the down-regulation of the UHRF1/DNMT1 tandem. Therefore, we also analyzed the levels of these epigenetic proteins after MPTE exposure to cells and observed a clear downregulation of UHRF1 and DNMT1 in a dose dependent manner (Fig. 6A, C and D). Treatment with 300 μg mL−1 of MPTE induced more than 60% decrease in UHRF1 and DNMT1 levels as compared to controls (Fig. 6C and D).
Since UHRF1 and DNMT1 are primarily involved in the maintenance of the DNA methylation pattern during the replication, we also checked the effect of MPTE treatment on the global DNA methylation level in cells by using the Imprint® methylated DNA quantification assay. After treatment with different concentrations of MPTE for 24 h, our data indicated a decrease in the global methylation levels (Fig. 6E). For MPTE concentrations of 150 μg mL−1 and 300 μg mL−1, the global methylation levels were, respectively, 25% and 33% less than those in the control samples.
4. Discussion
Many studies have shown a beneficial role of polyphenolic plant products in the prevention and cure of cancers. Such products are now being thoroughly explored for their possible application in the treatment of tumors by identifying their active ingredients and their possible mechanism of action.38,39 Maritime pine bark has been previously reported to induce differentiation and apoptosis in cancer cells.21–24 The aim of this study was to investigate the underlying mechanism by drawing attention to the epigenetic actors, considering that differentiation and apoptosis are regulated by epigenetic mechanisms such as DNA methylation. We focused on the DNMT1/UHRF1 tandem as it is responsible for DNA methylation maintenance and DNA methylation pattern inheritance.28
MPTE has been well characterized chemically. MALDI-TOF and 13C NMR analysis of this extract revealed that it contains a mixture of condensed tannins (procyanidins) made up of varying subunits of catechin, epicatechin, epigallocatechin, epicatechin gallate and fisetinidin monomers (Fig. 7A).11 Our HPLC/MS analysis also revealed the presence of lanost-9(11)-ene-3,24,25-triol from the terpenoid class of compounds (Fig. 7B).
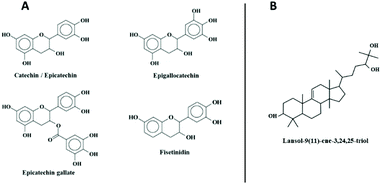 |
| Fig. 7 Major phytochemicals in maritime pine tannin extract. | |
The different physiological properties of maritime pine bark extract such as antioxidant, anti-inflammatory, antidiabetic and cardioprotective effects have been attributed to the presence of these polyphenolic compounds.9,10 Other pine bark extracts such as the Pinus massoniana bark extract, Pinus strobus bark extract and Pinus radiata bark extract are also rich in procyanidins and possess similar bioactivities.13,17 These compounds are also present in different combinations in green tea leaves and their anticancer properties have been shown in different studies.37,40 It is also observed that these compounds work better in combination with a plant product rather than individually, because in combination, they are effective at a lower dose and cause less toxicity.41 In MPTE, we also observed the presence of lanoste based terpenoids which have been previously observed in Pinus monticola and Pinus luchuensis.18,19
Here, we observed that treatment with MPTE inhibited the proliferation of cervical cancer HeLa and osteosarcoma U2OS cell lines at a low concentration compared to primary fibroblasts, showing a specific response towards cancer cells. The inhibition of proliferation under MPTE treatment also resulted in the accumulation of cells in the G2/M phase, which is commonly seen with anticancer compounds.42–44 MPTE induced apoptosis in HeLa cells, as indicated by flow cytometry analysis using annexin V and PI labelling. Western blotting results confirmed the induction of apoptosis by showing the activation of caspase 3 and the cleavage of PARP in a dose dependent manner in MPTE treated cells. The levels of the pro-survival protein Bcl-2 which is necessary for the maintenance of the integrity of the mitochondrial membrane were also decreased during treatment with MPTE. The loss of Bcl-2 results in the release of cytochrome c and thus leads to apoptosis.
Similar results have been observed in cervical, hepatoma, sarcoma and lung cancer cells by treatment with P. massoniana bark extract (PMBE) which also contains B-type procyanidin as the major constituent.45–50 The treatment of cancer cells with PMBE reduced the migration of tumor cells and induced apoptosis by the downregulation of the Bcl-2 protein.45,48 Similar effects were also observed with P. radiata bark extract, which contains a high amount of polyphenolic compounds such as quercetin, dihydroquercetin, catechin, epicatechin, taxifolin and other procyanidin oligomers and polymers.13,15 This extract also induces apoptosis in lymphoma cells when used in combination with aquamin and green tea extract in a dose-dependent manner by increasing caspase 3 activity.51
In a similar manner, a number of polyphenolic compounds, e.g. (−) epigallocatechin gallate, butein and curcumin, have been shown to induce apoptosis in a variety of cancer cells by activating caspase 3 and PARP cleavage along with the downregulation of the Bcl-2 protein.4,52–55 Along with polyphenolic compounds, lanosterol derived compounds are also well known for their potential to exert cytotoxic effects and induce apoptosis. These compounds can potentiate the effect of tumor suppressor proteins and activate the BAX protein for apoptosis in cancer cells.56,57
In contrast to the previously reported antioxidant activity of MPTE,9 we observed a pro-oxidant role of MPTE in cancer cells, which was evidenced by a dose dependent increase in ROS staining which was sensitive to the antioxidants PEG-catalase and N-acetylcysteine. These results show that H2O2 is an important factor in mediating the anti-cancer activity of MPTE as has been reported, for instance, for white grape pomace extract.58 Furthermore, it suggests that superoxide dismutase is involved in mediating such an effect as this enzyme is responsible for the generation of H2O2 from superoxide anions, themselves scavenged in the presence of NAC. This explains the subsequent cascade of events, i.e. DNA damage and cell apoptosis.59,60
In agreement with our study, polyphenol-rich extracts from tea leaves, grapes, fruits and berries have also been reported to induce ROS production and apoptosis in cancer cells despite their predominantly antioxidant effects in normal cells.61 Polyphenols can act both as an antioxidant and a pro-oxidant depending on the nature of cells. In normal cells, polyphenolic compounds protect the cellular material from oxidative stress because of their antioxidant activity. In cancer cells, they behave as pro-oxidant compounds and kill the cells as a result of the high pH and the increased level of redox active transient metals.61,62 This specific targeting of cancer cells makes the polyphenolic compounds interesting candidates for anticancer therapy. Increased ROS levels are also associated with increased granularity in cell-induced senescence, indicating early signs of apoptosis.35,63 Similar results have also been observed with Pinostrobin, a flavonoid from Pinus strobus which induces apoptosis in HeLa cells by excessive ROS production.64
Interestingly, MPTE treatment was observed to induce the expression of the tumor suppressor protein p73 in p53-deficient HeLa cells. Previously, it has been reported that ROS production can activate p73 expression in HeLa cells and induce their apoptosis through a mitochondrial activation of caspases 9 and 3.65 The activation of p73 and apoptosis induced by a p73-dependent mechanism have also been observed in a number of plant products including thymoquinone, epigallocatechin-3-gallate and polyphenolic extracts from grapes and berries added to p53-deficient cancer cells.30,36,37,58,61,66–68
Earlier, we have reported in several studies that p53 and p73 also negatively regulate the epigenetic mediator UHRF1 in cancer cells.36,37 In this study, we also observed the downregulation of UHRF1 along with the upregulation of p73 upon MPTE treatment in HeLa cells, in line with the previous data. UHRF1 is mostly found upregulated in cancers, where it promotes cell oncogenesis by facilitating their passage through the cell cycle.69 Elevated levels of UHRF1 directly interfere with the function of the tumor suppressor genes (TSGs) and downregulate a variety of TSGs through their promoter hypermethylation. UHRF1 upregulation also makes cancer cells resistive to anticancer therapy by facilitating DNA damage repair, highlighting its potential as a target for anticancer therapy.27,69 Therefore, the knockdown of UHRF1 by siRNA or other plant products such as EGCG, thymoquinone or polyphenolic extracts induced apoptosis in cancer cells and improved the response of resistive tumor cells to anticancer therapy.27,36,37 In our current study, we observed a MPTE-induced downregulation of UHRF1 and blockade of cells in the G2/M phase, in agreement with a previous finding where UHRF1 depletion resulted in a cell cycle arrest in the G2/M phase.70 MPTE also downregulated the expression of DNMT1. The downregulation of both DNMT1 and UHRF1 contributed to recovery of the expression of TSGs from a silenced state.27,71 This re-expression likely results from a global genome hypomethylation. In our case, the downregulation of UHRF1 and DNMT1 was followed by global hypomethylation after treatment with MPTE. In agreement with our observations, catechol-containing dietary polyphenols have been previously described to interfere with the DNA methylation process.72
5. Conclusions
In conclusion, we reported here that MPTE inhibited the proliferation of HeLa cells by inducing cell cycle arrest in the G2/M phase along with a ROS mediated activation of the mitochondrial apoptotic pathway. It is concluded that the formation of H2O2 plays a major role in the increase of the expression of the tumor suppressor gene TP73 as a result of the downregulation of the UHRF1/DNMT1 tandem and a reduced genome global methylation.
Author contributions
C. B. and M. M. conceived the project. W. A., T. A., N. A. R. A., C. D. M., M. K., A. P., A. H. and L. Z. performed the experiments. Data were curated by W. A. and T. A. The original draft was written by W. A., T. A., Y. M., C. B. and M. M. Funding acquisition was done by M. M. and Y. M. All authors were involved in the proofreading of the manuscript.
Conflicts of interest
The authors declare no conflict of interest.
Acknowledgements
The authors thank Dr Delphine Garnier from the technical platform PACSI of the Faculty of Pharmacy for performing the NMR spectroscopy and HPLC/MS analysis of the PMTE. Funding: This research was supported by ANR (SMFLUONA, ANR-17-CE11-0036-01). W. A. and T. A. were supported by fellowships from the Higher Education Commission (HEC), Pakistan. Y. M. is grateful to the Institut Universitaire de France (IUF) for support and providing additional time to be dedicated to research.
References
- J. Ferlay, I. Soerjomataram, R. Dikshit, S. Eser, C. Mathers, M. Rebelo, D. M. Parkin, D. Forman and F. Bray, Cancer Incidence and Mortality Worldwide: Sources, Methods and Major Patterns in GLOBOCAN 2012, Int. J. Cancer, 2015, 136, E359–E386 CrossRef CAS PubMed.
- H. Gali-Muhtasib, R. Hmadi, M. Kareh, R. Tohme and N. Darwiche, Cell Death Mechanisms of Plant-Derived Anticancer Drugs: Beyond Apoptosis, Apoptosis, 2015, 20, 1531–1562 CrossRef CAS PubMed.
- A. Rayan, J. Raiyn and M. Falah, Nature Is the Best Source of Anticancer Drugs: Indexing Natural Products for Their Anticancer Bioactivity, PLoS One, 2017, 12, e0187925 CrossRef PubMed.
- J. Wang, Y. Pan, J. Hu, Q. Ma, Y. Xu, Y. Zhang, F. Zhang and Y. Liu, Tea Polyphenols Induce S Phase Arrest and Apoptosis in Gallbladder Cancer Cells, Rev. Bras. Pesqui. Med. Biol., 2018, 51, e6891 Search PubMed.
- M. Greenwell and P. K. S. M. Rahman, Medicinal Plants: Their Use in Anticancer Treatment, Int. J. Pharma Sci. Res., 2015, 6, 4103–4112 CAS.
- J. Iqbal, B. A. Abbasi, T. Mahmood, S. Kanwal, B. Ali, S. A. Shah and A. T. Khalil, Plant-Derived Anticancer Agents: A Green Anticancer Approach, Asian Pac. J. Trop. Biomed., 2017, 7, 1129–1150 CrossRef.
- S. Xie and J. Zhou, Harnessing Plant Biodiversity for the Discovery of Novel Anticancer Drugs Targeting Microtubules, Front. Plant Sci., 2017, 8, 1–8 Search PubMed.
- L. Chupin, A. Pizzi, B. Charrier, C. Motillon and F. Charrier-El Bouhtoury, Characterisation of Maritime Pine (Pinus Pinaster) Bark Tannins Extracted under Different Conditions by Spectroscopic Methods, FTIR and HPLC, Ind. Crops Prod., 2013, 49, 897–903 CrossRef CAS.
- L. Packer, G. Rimbach and F. Virgili, Antioxidant Activity and Biologic Properties of a Procyanidin-Rich Extract from Pine (Pinus Maritima) Bark, Pycnogenol, Free Radicals Biol. Med., 1999, 27, 704–724 CrossRef CAS PubMed.
- P. J. Rohdewald, Update on the Clinical Pharmacology of Pycnogenol(R), Med. Res. Arch., 2015, 3, 1–11 Search PubMed.
- P. Navarrete, A. Pizzi, H. Pasch, K. Rode and L. Delmotte, MALDI-TOF and 13C NMR Characterization of Maritime Pine Industrial Tannin Extract, Ind. Crops Prod., 2010, 32, 105–110 CrossRef CAS.
- M. de la Luz Cádiz-Gurrea, S. Fernández-Arroyo and A. Segura-Carretero, Pine Bark and Green Tea Concentrated Extracts: Antioxidant Activity and Comprehensive Characterization of Bioactive Compounds by HPLC-ESI-QTOF-MS, Int. J. Mol. Sci., 2014, 15, 20382–20402 CrossRef PubMed.
- Y.-Y. Li, J. Feng, X.-L. Zhang and Y.-Yu. Cui, Pine bark extracts: nutraceutical, pharmacological, and toxicological evaluation, J. Pharmacol. Exp. Ther., 2015, 353, 9–16 CrossRef CAS PubMed.
- A. Theadom, S. Mahon, S. Barker-Collo, K. McPherson, E. Rush, A. C. Vandal and V. L. Feigin, Enzogenol for cognitive functioning in traumatic brain injury: a pilot placebo-controlled RCT, Eur. J. Neurol., 2013, 20, 1135–1144 CrossRef CAS PubMed.
- T. Ghaffari, J.-H. Hong, S. Asnaashari, S. Farajnia, A. Delazar, H. Hamishehkar and K. H. Kim, Natural Phytochemicals Derived from Gymnosperms in the Prevention and Treatment of Cancers, Int. J. Mol. Sci., 2021, 22, 6636–6652 CrossRef CAS PubMed.
- Y. Cui, H. Xie and J. Wang, Potential biomedical properties of Pinus massoniana bark extract, Phytother. Res., 2005, 19, 34–38 CrossRef PubMed.
- Y. Yazaki, Utilization of Flavonoid Compounds from Bark and Wood: A Review, Nat. Prod. Commun., 2015, 10, 1934578X1501000333 CAS.
- D. M. Fradinho, C. P. Neto, D. Evtuguin, F. C. Jorge, M. A. Irle, M. H. Gil and J. Pedrosa de Jesus, Chemical characterisation of bark and of alkaline bark extracts from maritime pine grown in Portugal, Ind. Crops Prod., 2002, 16, 23–32 CrossRef CAS.
- A. H. Conner, B. A. Nagasampagi and J. W. Rowe, Terpenoid and other extractives of western white pine bark, Phytochemistry, 1980, 19, 1121–1131 CrossRef CAS.
- J. L. C. Sousa, P. A. B. Ramos, C. S. R. Freire, A. M. S. Silva and A. J. D. Silvestre, Chemical Composition of Lipophilic Bark Extracts from Pinus pinaster and Pinus pinea Cultivated in Portugal, Appl. Sci., 2018, 8, 1–11 Search PubMed.
- H. T. Huynh and R. W. Teel, Selective Induction of Apoptosis in Human Mammary Cancer Cells (MCF-7) by Pycnogenol, Anticancer Res., 2000, 20, 2417–2420 CAS.
- W. W. Huang, J. S. Yang, C. F. Lin, W. J. Ho and M. R. Lee, Pycnogenol Induces Differentiation and Apoptosis in Human Promyeloid Leukemia HL-60 Cells, Leuk. Res., 2005, 29, 685–692 CrossRef CAS PubMed.
- A. R. Buz'Zard and B. H. S. Lau, Pycnogenol Reduces Talc-Induced Neoplastic Transformation in Human Ovarian Cell Cultures, Phytother. Res., 2007, 21, 579–586 CrossRef PubMed.
- K. Harati, P. Slodnik, A. M. Chromik, B. Behr, O. Goertz, T. Hirsch, N. Kapalschinski, L. Klein-Hitpass, J. Kolbenschlag, W. Uhl, M. Lehnhardt and A. Daigeler, Pro-apoptotic Effects of Pycnogenol on HT1080 Human Fibrosarcoma Cells, Int. J. Oncol., 2015, 46, 1629–1636 CrossRef CAS PubMed.
- G. Belcaro, M. R. Cesarone, D. Genovesi, A. Ledda, G. Vinciguerra, A. Ricci, L. Pellegrini, G. Gizzi, E. Ippolito and M. Dugall, Pycnogenol May Alleviate Adverse Effects in Oncologic Treatment, Panminerva Med., 2008, 50, 227–234 CAS.
- C. Bronner, G. Fuhrmann, F. L. Chédin, M. Macaluso and S. Dhe-Paganon, UHRF1 Links the Histone Code and DNA Methylation to Ensure Faithful Epigenetic Memory Inheritance, Genet. Epigenet., 2010, 2009, 29–36 Search PubMed.
- C. Bronner, M. Krifa and M. Mousli, Increasing Role of UHRF1 in the Reading and Inheritance of the Epigenetic Code as Well as in Tumorogenesis, Biochem. Pharmacol., 2013, 86, 1643–1649 CrossRef CAS PubMed.
- C. Bronner, M. Alhosin, A. Hamiche and M. Mousli, Coordinated Dialogue between UHRF1 and DNMT1 to Ensure Faithful Inheritance of Methylated DNA Patterns, Genes, 2019, 10, 65 CrossRef PubMed.
- M. Alhosin, T. Sharif, M. Mousli, N. Etienne-Selloum, G. Fuhrmann, V. B. Schini-Kerth and C. Bronner, Down-Regulation of UHRF1, Associated with Re-Expression of Tumor Suppressor Genes, Is a Common Feature of Natural Compounds Exhibiting Anti-Cancer Properties, J. Exp. Clin. Cancer Res., 2011, 30, 1–10 CrossRef PubMed.
- M. Alhosin, Z. Omran, M. A. Zamzami, A. L. Al-Malki, H. Choudhry, M. Mousli and C. Bronner, Signalling Pathways in UHRF1-Dependent Regulation of Tumor Suppressor Genes in Cancer, J. Exp. Clin. Cancer Res., 2016, 35, 1–11 CrossRef PubMed.
- Y. Amrani, C. Magnier, J. Enouf, F. Wuytack and C. Bronner, Role of Ca2+ Pools Controlled by Sarco-Endoplasmic Reticulum Ca(2+)-ATPase 2 Isoform, Br. J. Pharmacol., 1995, 115, 1204–1210 CrossRef CAS PubMed.
- Y. Amrani, R. A. J. Panettieri, N. Frossard and C. Bronner, Activation of the TNF Alpha-P55 Receptor Induces Myocyte Proliferation and Modulates Agonist-Evoked Calcium Transients in Cultured Human Tracheal Smooth Muscle Cells, Am. J. Respir. Cell Mol. Biol., 1996, 15, 55–63 CrossRef CAS PubMed.
- L. Zaayter, M. Mori, T. Ahmad, W. Ashraf, C. Boudier, V. Kilin, K. Gavvala, L. Richert, S. Eiler, M. Ruff, M. Botta, C. Bronner, M. Mousli and Y. Mély, A Molecular Tool Targeting the Base-Flipping Activity of Human UHRF1, Chem. – Eur. J., 2019, 25, 13363–13375 CrossRef CAS PubMed.
- R. Hopfner, M. Mousli, J. M. Jeltsch, A. Voulgaris, Y. Lutz, C. Marin, J. P. Bellocq, P. Oudet and C. Bronner, ICBP90, a Novel Human CCAAT Binding Protein, Involved in the Regulation of Topoisomerase II alpha Expression, Cancer Res., 2000, 60, 121–128 CAS.
- K. Gosselin, E. Deruy, S. Martien, C. Vercamer, F. Bouali, T. Dujardin, C. Slomianny, L. Houel-Renault, F. Chelli, Y. De Launoit and C. Abbadie, Senescent Keratinocytes Die by Autophagic Programmed Cell Death, Am. J. Pathol., 2009, 174, 423–435 CrossRef CAS PubMed.
- M. Alhosin, A. Abusnina, M. Achour, T. Sharif, C. Muller, J. Peluso, T. Chataigneau, C. Lugnier, V. B. Schini-Kerth, C. Bronner and G. Fuhrmann, Induction of Apoptosis by Thymoquinone in Lymphoblastic Leukemia Jurkat Cells Is Mediated by a P73-Dependent Pathway Which Targets the Epigenetic Integrator UHRF1, Biochem. Pharmacol., 2010, 79, 1251–1260 CrossRef CAS PubMed.
- M. Achour, M. Mousli, M. Alhosin, A. Ibrahim, J. Peluso, C. D. Muller, V. B. Schini-Kerth, A. Hamiche, S. Dhe-Paganon and C. Bronner, Epigallocatechin-3-Gallate up-Regulates Tumor Suppressor Gene Expression via a Reactive Oxygen Species-Dependent down-Regulation of UHRF1, Biochem. Biophys. Res. Commun., 2013, 430, 208–212 CrossRef CAS PubMed.
- M. Asensi, A. Ortega, S. Mena, F. Feddi and J. M. Estrela, Natural Polyphenols in Cancer Therapy, Crit. Rev. Clin. Lab. Sci., 2011, 48, 197–216 CrossRef CAS PubMed.
- Y. Zhou, J. Zheng, Y. Li, D.-P. Xu, S. Li, Y.-M. Chen and H.-B. Li, Natural Polyphenols for Prevention and Treatment of Cancer, Nutrients, 2016, 8, 1–35 CrossRef CAS PubMed.
- C. S. Yang and H. Wang, Cancer Preventive Activities of Tea Catechins, Molecules, 2016, 21, 1–19 Search PubMed.
- A. M. Bode and Z. Dong, Epigallocatechin 3-Gallate and Green Tea Catechins: United They Work, Divided They Fail, Cancer Prev. Res., 2009, 2, 514–517 CrossRef CAS PubMed.
- S. Senese, Y. C. Lo, D. Huang, T. A. Zangle, A. A. Gholkar, L. Robert, B. Homet, A. Ribas, M. K. Summers, M. A. Teitell, R. Damoiseaux and J. Z. Torres, Chemical Dissection of the Cell Cycle: Probes for Cell Biology and Anti-Cancer Drug Development, Cell Death Dis., 2014, 5, e1462 CrossRef CAS PubMed.
- H.-M. Shan, Y. Shi and J. Quan, Identification of Green Tea Catechins as Potent Inhibitors of the Polo-Box Domain of Polo-like Kinase 1, ChemMedChem, 2015, 10, 158–163 CrossRef CAS PubMed.
- K. Takanashi, M. Suda, K. Matsumoto, C. Ishihara, K. Toda, K. Kawaguchi, S. Senga, N. Kobayashi, M. Ichikawa, M. Katoh, Y. Hattori, S. Kawahara, K. Umezawa, H. Fujii and H. Makabe, Epicatechin Oligomers Longer than Trimers Have Anti-Cancer Activities, but Not the Catechin Counterparts, Sci. Rep., 2017, 7, 7791 CrossRef PubMed.
- Y.-Y. Cui, H. Xie, K.-B. Qi, Y.-M. He and J.-F. Wang, Effects of Pinus massoniana bark extract on cell proliferation and apoptosis of human hepatoma BEL-7402 cells, World J. Gastroenterol., 2005, 11, 5277–5282 CrossRef CAS PubMed.
- J.-H. Zhang, D.-R. Feng, H.-L. Ma, B. Liu, H.-B. Wang, H. Xie, R.-D. Li and J.-F. Wang, Antitumor
Effects of Pinus massoniana Bark Extract in Murine Sarcoma S180 Both in Vitro and in Vivo, Am. J. Chin. Med., 2012, 40, 861–875 CrossRef PubMed.
- H. Ma, B. Liu, D. Feng, H. Xie, R. Li, Y. Yuchi, H. Wang and J. Wang, Pinus massoniana bark extract selectively induces apoptosis in human hepatoma cells, possibly through caspase-dependent pathways, Int. J. Mol. Med., 2010, 25, 751–759 Search PubMed.
- D.-C. Wu, S. Li, D.-Q. Yang and Y.-Y. Cui, Effects of Pinus massoniana bark extract on the adhesion and migration capabilities of HeLa cells, Fitoterapia, 2011, 82, 1202–1205 CrossRef PubMed.
- J. Liu, J. Bai, G. Jiang, X. Li, J. Wang, D. Wu, L. Owusu, E. Zhang and W. Li, Anti-Tumor Effect of Pinus massoniana Bark Proanthocyanidins on Ovarian Cancer through Induction of Cell Apoptosis and Inhibition of Cell Migration, PLoS One, 2015, 10, e0142157 CrossRef PubMed.
- P. Mao, E. Zhang, Y. Chen, L. Liu, D. Rong, Q. Liu and W. Li,
Pinus massoniana bark extract inhibits migration of the lung cancer A549 cell line, Oncol. Lett., 2017, 13, 1019–1023 CrossRef CAS PubMed.
- Y. C. O'Callaghan, E. Drummond, D. M. O'Gorman and N. M. O'Brien, Antioxidant and pro-apoptotic effects of marine-derived, multi-mineral aquamin supplemented with a pine bark extract, Enzogenol, and a green tea extract, Sunphenon, J. Med. Food, 2013, 16, 920–926 CrossRef PubMed.
- B. Halder, U. Bhattacharya, S. Mukhopadhyay and A. K. Giri, Molecular Mechanism of Black Tea Polyphenols Induced Apoptosis in Human Skin Cancer Cells: Involvement of Bax Translocation and Mitochondria Mediated Death Cascade, Carcinogenesis, 2008, 29, 129–138 CrossRef CAS PubMed.
- Y.-H. Chen, C.-W. Yeh, H.-C. Lo, S.-L. Su, Y.-C. Hseu and L.-S. Hsu, Generation of Reactive Oxygen Species Mediates Butein-Induced Apoptosis in Neuroblastoma Cells, Oncol. Rep., 2012, 27, 1233–1237 CrossRef CAS PubMed.
- J. Wang, Y. Xie, Y. Feng, L. Zhang, X. Huang, X. Shen and X. Luo, (-)-Epigallocatechingallate Induces Apoptosis in B Lymphoma Cells via Caspase-Dependent Pathway and Bcl-2 Family Protein Modulation, Int. J. Oncol., 2015, 46, 1507–1515 CrossRef CAS PubMed.
- L. Zhu, M. B. Han, Y. Gao, H. Wang, L. Dai, Y. Wen and L. X. Na, Curcumin Triggers Apoptosis via Upregulation of Bax/Bcl-2 Ratio and Caspase Activation in SW872 Human Adipocytes, Mol. Med. Rep., 2015, 12, 1151–1156 CrossRef CAS PubMed.
- J.-L. Ríos, I. Andújar, M.-C. Recio and R.-M. Giner, Lanostanoids from Fungi: A Group of Potential Anticancer Compounds, J. Nat. Prod., 2012, 75, 2016–2044 CrossRef PubMed.
- D. Darwati, A. Tsamrotul, T. Herlina, T. Mayanti, N. Nurlelasari, K. Haikal and U. Supratman, Triterpenoids from The Bark of Garcinia porecta and their Cytotoxic Activity against MCF7 Breast Cancer Lines, ALCHEMY J. Penelit. Kim., 2019, 15, 1–9 CrossRef.
- A. J. León-González, M. J. Jara-Palacios, M. Abbas, F. J. Heredia and V. B. Schini-Kerth, Role of Epigenetic Regulation on the Induction of Apoptosis in Jurkat Leukemia Cells by White Grape Pomace Rich in Phenolic Compounds, Food Funct., 2017, 8, 4062–4069 RSC.
- L. Qi, X.-C. Wu and D.-Q. Zheng, Hydrogen peroxide, a potent inducer of global genomic instability, Curr. Genet., 2019, 65, 913–917 CrossRef CAS PubMed.
- J. N. Cobley, N. V. Margaritelis, J. P. Morton, G. L. Close, M. G. Nikolaidis and J. K. Malone, The basic chemistry of exercise-induced DNA oxidation: oxidative damage, redox signaling, and their interplay, Front. Physiol., 2015, 6, 182–182 Search PubMed.
- A. J. León-González, C. Auger and V. B. Schini-Kerth, Pro-Oxidant Activity of Polyphenols and Its Implication on Cancer Chemoprevention and Chemotherapy, Biochem. Pharmacol., 2015, 98, 371–380 CrossRef PubMed.
- S. Eghbaliferiz and M. Iranshahi, Prooxidant Activity of Polyphenols, Flavonoids, Anthocyanins and Carotenoids: Updated Review of Mechanisms and Catalyzing Metals, Phytother. Res., 2016, 30, 1379–1391 CrossRef CAS PubMed.
- G. V. Raghuram, N. Pathak, D. Jain, H. Panwar, H. Pandey, S. K. Jain and P. K. Mishra, Molecular Mechanisms of Isocyanate Induced Oncogenic Transformation in Ovarian Epithelial Cells, Reprod. Toxicol., 2010, 30, 377–386 CrossRef CAS PubMed.
- X. Sun, X. Liu and S. Chen, The Pharmacokinetics, Tissue Distribution, Metabolism, and Excretion of Pinostrobin in Rats: Ultra-High-Performance Liquid Chromatography Coupled With Linear Trap Quadrupole Orbitrap Mass Spectrometry Studies, Front. Pharmacol., 2020, 11, 574638–574638 CrossRef CAS PubMed.
- M. Singh, H. Sharma and N. Singh, Hydrogen Peroxide Induces Apoptosis in HeLa Cells through Mitochondrial Pathway, Mitochondrion, 2007, 7, 367–373 CrossRef CAS PubMed.
- A. Abusnina, T. Keravis, I. Yougbaré, C. Bronner and C. Lugnier, Anti-Proliferative Effect of Curcumin on Melanoma Cells Is Mediated by PDE1A Inhibition That Regulates the Epigenetic Integrator UHRF1, Mol. Nutr. Food Res., 2011, 55, 1677–1689 CrossRef CAS PubMed.
- T. Sharif, M. Alhosin, C. Auger, C. Minker, J.-H. Kim, N. Etienne-Selloum, P. Bories, H. Gronemeyer, A. Lobstein, C. Bronner, G. Fuhrmann and V. B. Schini-Kerth, Aronia Melanocarpa Juice Induces a Redox-Sensitive P73-Related Caspase 3-Dependent Apoptosis in Human Leukemia Cells, PLoS One, 2012, 7, e32526 CrossRef CAS PubMed.
- A. Ibrahim, M. Alhosin, C. Papin, K. Ouararhni, Z. Omran, M. A. Zamzami, A. L. Al-Malki, H. Choudhry, Y. Mély, A. Hamiche, M. Mousli and C. Bronner, Thymoquinone Challenges UHRF1 to Commit Auto-Ubiquitination: A Key Event for Apoptosis Induction in Cancer Cells, Oncotarget, 2018, 9, 28599–28616 CrossRef PubMed.
- W. Ashraf, A. Ibrahim, M. Alhosin, L. Zaayter, K. Ouararhni, C. Papin, T. Ahmad, A. Hamiche, Y. Mély, C. Bronner and M. Mousli, The Epigenetic Integrator UHRF1: On the Road to Become a Universal Biomarker for Cancer, Oncotarget, 2017, 8, 51946–51962 CrossRef PubMed.
- A. L. Tien, S. Senbanerjee, A. Kulkarni, R. Mudbhary, B. Goudreau, S. Ganesan, K. C. Sadler and C. Ukomadu, UHRF1 Depletion Causes a G2/M Arrest, Activation of DNA Damage Response and Apoptosis, Biochem. J., 2011, 435, 175–185 CrossRef CAS PubMed.
- M. Unoki, Current and Potential Anticancer Drugs Targeting Members of the UHRF1 Complex Including Epigenetic Modifiers, Recent Pat. Anticancer Drug Discovery, 2011, 6, 116–130 CrossRef CAS PubMed.
- B. Stefanska, H. Karlic, F. Varga, K. Fabianowska-Majewska and A. Haslberger, Epigenetic Mechanisms in Anti-Cancer Actions of Bioactive Food Components–the Implications in Cancer Prevention, Br. J. Pharmacol., 2012, 167, 279–297 CrossRef CAS PubMed.
Footnotes |
† Electronic supplementary information (ESI) available. See DOI: 10.1039/d1fo01484f |
‡ Equal first authors. |
§ These authors are equal senior authors. |
|
This journal is © The Royal Society of Chemistry 2022 |
Click here to see how this site uses Cookies. View our privacy policy here.