Composition, concentration, and oxidant reactivity of sesquiterpenes in the southeastern U.S.†
Received
26th May 2022
, Accepted 12th August 2022
First published on 15th August 2022
Abstract
Biogenic volatile organic compounds (BVOCs) contribute the majority of reactive organic carbon to the atmosphere and lead to aerosol formation through reaction with atmospheric oxidants including ozone and hydroxyl radicals. One class of BVOCs, sesquiterpenes, have a high reactivity with ozone but exist at lower concentrations compared to other BVOCs, and there are relatively few measurements of their concentrations in different environments or their importance in the atmospheric oxidant budget. To help close this knowledge gap, we examine concentrations of isomer-resolved sesquiterpene concentrations collected hourly at two sites in Virginia that are representative of different ecosystems in the southeastern US. Sesquiterpene concentrations are presented and discussed in relation to their diurnal patterns and used to estimate their contribution to reactivity with common gas-phase oxidants. Twenty-four sesquiterpenes were identified at the sites, eleven of which were observed at both sites. Total sesquiterpene concentrations were found to range between 0.8 and 2 ppt with no single isomer dominating throughout. Hydroxyl activity is similarly diverse, with no particular isomer dominating activity at either site. Ozone reactivity, however, was found to be dominated (∼3/4 total reactivity) by β-caryophyllene and humulene despite these compounds representing roughly only 10% of total sesquiterpene mass, highlighting their importance as the major driver of sesquiterpene-ozone reactivity. Average reaction rate constants for sesquiterpenes with ozone and hydroxyl radicals were calculated for both sites as a method to simplify future atmospheric modelling concerning sesquiterpenes. This work provides broad insight into the composition and impacts of sesquiterpenes, suggesting that sesquiterpene composition is relatively similar between sites. Furthermore, while the calculated average sesquiterpene-ozone reaction rate constants are at least an order of magnitude higher than that of more prevalent BVOC classes (isoprene and monoterpenes), their low concentrations suggest their impacts on atmospheric reactivity are expected to be limited to periods of high emissions.
Environmental significance
Sesquiterpenes represent a small fraction of emissions from natural sources, but because of their fast reaction rates with ozone and efficient formation of particulate matter, their impacts could be significant compared to other chemical classes of natural emissions. Unfortunately, information in the literature for sesquiterpenes is sparse, leading to uncertainty in the impact of sesquiterpenes on atmospheric oxidants and chemistry. Our work sheds light on the composition and concentration of sesquiterpenes in the southeastern U.S. and examines their likely impacts on the regional atmosphere. In particular, two low-concentration isomers dominate ozone reactivity, but also have large discrepancies between predicted and measured reaction rates, highlighting the need for measurements of ozone reactivity with other sesquiterpene isomers.
|
A Introduction
Emissions of biogenic volatile organic compounds (BVOCs) constitute the largest source of reactive organic carbon to the atmosphere.1,2 These compounds, primarily terpenoids, are comprised of one or more isoprene (C5H8) units in a variety of configurations that result in monoterpenes (C10H16), sesquiterpenes (C15H24), and diterpenes (C20H32). Globally, isoprene represents the majority of these emissions at rates of approximately 500 teragrams of carbon per year (Tg C y−1), while monoterpenes account for somewhat less (∼160 Tg C y−1), sesquiterpenes are even lower (∼30 Tg C y−1), and emissions of diterpenes are largely unconstrained.1,3
Notably, the information on the higher molecular weight BVOCs is more sparse in the literature than for the more dominant classes of lighter compounds, leading to substantial uncertainty in their emissions and concentrations.
Upon emission into the atmosphere, these compounds react with atmospheric oxidants to form a plethora of oxygenated compounds, significantly impacting atmospheric oxidant budgets, radiative balance, and the global carbon cycle.4–10 Emissions of sesquiterpenes from plants is complex, driven by a wide range of biologically and ecologically diverse external stimuli including predation, changing environmental parameters, and oxidant concentrations influencing sesquiterpene emissions in addition to differences in plant species dependent sesquiterpene production.11–18 Laboratory experiments provide insight into the oxidation of BVOCs and their fate but challenges remain.19,20 In particular, there are relatively few real-world, time resolved measurements of higher molecular weight terpenoids (e.g., sesquiterpenes). Sesquiterpenes tend to react faster with atmospheric oxidants and have higher yields of secondary organic aerosol (SOA) compared to lower mass terpenoids.21,22 Furthermore, dozens of isomers are known within each terpenoid class, and their physicochemical properties and reaction rates vary by orders of magnitude, so it is difficult to accurately use an average or proxy reaction rate to describe the compound class.21 Due to the low volatility of higher molecular weight terpenoids, their high ozone reactivity and lower concentrations, and the diversity of their molecular structures, measuring them with sufficient chemical and temporal detail to understand atmospheric impacts requires high sensitivity, careful inlet design to avoid losses, and isomer resolution.23,24 These difficulties have resulted in a limited understanding of these compounds relative to more abundant, higher volatility terpenoids (isoprene, monoterpenes). However, previous work demonstrates that even low concentrations of certain sesquiterpenes can dominate ozone reactivity, so it is critical to further understand this class of reactive carbon.25
Field observations and laboratory experiments show monoterpenes and isoprene can be significant contributors to SOA.20 In contrast, a large amount of uncertainty exists regarding the role of sesquiterpenes in SOA formation.26 In laboratory conditions, higher molecular weight terpenoids have higher SOA yields, but it is not clear that these higher yields are sufficient for the low concentrations observed to contribute substantially to SOA.9,27,28 Modelling suggests that sesquiterpenes contribute to SOA formation as much or more than isoprene, but observations from several environments suggest this contribution may be far lower.29–31 A lack of ambient observations of sesquiterpenes limits understanding of this model-measurement disparity. A wide variety of sesquiterpenes and their respective oxidation products have been measured in the Amazon rainforest and other environments.25,32–35 However, ambient observations are quite limited for many environments, especially in comparison to other BVOC classes. Geographically and environmentally diverse measurements are necessary for a broader understanding of the composition, concentration, and impacts of sesquiterpenes, as anthropogenic and biogenic factors influence emissions and alter their impacts on the atmosphere.35–38 Sesquiterpene emission profiles vary between plant species and changes in the dominant vegetation can results in varied sesquiterpene emission profiles from one locale to another.19,39–41 Furthermore, anthropogenic and biogenic factors may influence emissions and alter their impacts on the atmosphere, so a complete understanding requires measurements across multiple ecosystems and environments.42 The uncertainty is reinforced by the wide range in sesquiterpene's ozone reactivity, where the use of one or a minimal number of reaction rate constants can over or underestimate sesquiterpenes influence on atmospheric chemistry and the atmospheric oxidant budget.
This work seeks to improve the understanding of sesquiterpenes through use of time-resolved measurements of sesquiterpene concentrations to estimate their contributions to atmospheric reactivity at two geographically close but ecologically distinct field sites in Virginia. Both sites have similar sesquiterpene speciated emission profiles, which we hypothesize represents an average description of sesquiterpenes in the southeastern United States and provides generalizable insight into their potential impact on the regional atmosphere. However, we demonstrate that anthropogenic activities can significantly impact their composition. Understanding sesquiterpene concentrations in this environment area helps to resolve uncertainties in oxidant reactivity and potential secondary aerosol formation.29
B Methods
SV-TAG instrumentation
The primary instrumentation used in this work is the semi-volatile thermal desorption aerosol gas chromatography, SV-TAG, for hourly quantification of gas- and particle-phase semi-volatile organic compounds. SV-TAG has been described in detail in previous work and will only briefly be described here.43–45 Air is sampled at 10 L min−1 for 40 minutes through a cyclone with a cutpoint diameter of 2.5 μm. Sample inlet varied by site as described below, but both used 3/8′′ O.D. conductive perfluoroalkoxy tubing (cPFA, Fluorostore), material shown to minimize sampling artifacts for both gases and particles.46 While sesquiterpenes in the gas phase may partition to the cPFA line during sampling, the high sampling flow rate and short inlet length are used to minimize losses from possible absorption and equilibration with the inlet walls. Based on the equations presented by Pagonis et al. and the operating conditions of SV-TAG, equilibration of the inlet is calculated to occur within the first minute of sampling at the tower and 3 minutes of sampling at the farm site, resulting in maximum sample loss of 3–8%.47 Sample flow then passed either through a multi-channel carbon denuder to remove all gas-phase compounds or a cPFA bypass line before sample concentration in a collection and thermal desorption cell (CTD) consisting of a passivated metal fiber filter within a temperature controlled stainless steel housing held at 30 °C. Using a two-step desorption process, the sample was then transferred to a gas chromatograph with a mass spectrometer as a detector (GC-MS). The sample was desorbed from the filter cell under a flow of 80 cm3 helium during which the CTD is ramped from 30 to 330 °C over 13 minutes. Previous work has shown that the SV-TAG cell can collect gas-phase compounds as volatile as tetradecane (similar to sesquiterpenes) with no observed decrease in collection efficiency.48 The sample then passes through a passivated stainless-steel valveless manifold held at 300 °C, and is reconcentrated onto a focusing trap consisting of a short length of chromatography column kept at 30 °C.45 During this desorption process, part of the helium flow volume passed through an N-methyl-N-(trimethylsilyl)trifluoroacetamide (MSTFA) reservoir before passing to the CTD to enable derivatization of hydroxyl containing species. The focusing trap was then heated to 330 °C over 5 minutes while being backflushed with helium through the valveless manifold to the head of a GC column. The GC consists of a non-polar metal capillary column (MXT-1, 17 m × 0.25 mm × 0.1 μm, Restek) wrapped around a temperature-controlled metal hub connected to an electron impact quadrupole MS (5977, Agilent Technologies). GC analysis occurs over 18 minutes, with a ramp of 15 °C min−1 from 60 to 300 °C and then held at 300 °C for 2 minutes. Data is collected over a mass range of m/z 30–550 at a scan rate of 2.8 Hz.
Calibration occurs through regular injections of liquid standards using an automatic injection system.49 A 5 μL sample loop is loaded and injected from one of four chilled reservoirs. Internal standards consisting of 7 deuterated compounds are injected into every sample before cell desorption begins and include: C14, C16, C18, C20, and C24 linear alkanes (CDN Isotope), and palmitic and lauric acids (Sigma Aldrich). External standards for calibrants occur every 6 runs at variable concentrations to create a multi-point calibration curve and include: C14–C28 linear alkanes, levoglucosan, dodecanediol, eugenol, adipic, and azelaic acid (Sigma-Aldrich). Kreisberg et al. have previously shown that liquid injections of calibrants provide accurate signal response compared to thermally desorbed standards, providing a reliable alternative to gas phase standards.50
Field sites overview
Tower site.
Sesquiterpene concentrations were measured at the Virginia Forest Lab (37.9229°N, 78.2739°W), located in Fluvanna County, Virginia. The tower sampling site is located in the Piedmont region on the eastern side of the Blue Ridge Mountains and receives some anthropogenic influence from Charlottesville, VA, which is located 25 km to the west of the site. The forest canopy is predominantly composed of maple, oaks, and pines and is approximately 24 meters tall.51 The site features a 40 m tall tower for atmospheric measurements and a climate-controlled, internet-connected lab with line power available. Measurements occurred between August 8th and August 19th, 2019, and were collected hourly using an SV-TAG mounted directly on the tower at ∼20 m, with an inlet of length ∼ 1 m.
Farm site.
Sesquiterpene concentration were measured at a small agricultural site located at 37.3621°N, 77.5222°W, in Powhatan County, Virginia. The farm sampling site is located at a rural suburban interface that is roughly 55 km west of Richmond, VA and 50 km southeast of the tower site. Measurements occurred between April 17th and May 15th, 2020. Measurements at this site were collected hourly using an SV-TAG mounted near the ceiling of a barn (approximately 3 m) located directly adjacent to agricultural fields used for hay production. The inlet was located on the outside of the barn near the roof, extending 1 m away from the building with a total inlet length of 6 m. The site is situated approximately 300 m from a mixed forest, that is ecologically similar to the tower site.
Quantification of analytes
Though SV-TAG captures a large variety of analytes, the scope of this work is limited to sesquiterpenes. Each sesquiterpene is identified by comparison of background subtracted mass spectra and associated retention index to the 2011 NIST Mass Spectral Library (National Instruments for Standards and Technology 2019). Analytes not included in the library are identified by comparison to previous field observations of sesquiterpenes, which are also used to validate all retention times and retention order.25 Data are analyzed using the freely-available TERN software package within the Igor Pro 8 programming environment (Wavemetrics, Inc.).52
Sesquiterpenes were calibrated based on the response factor of the nearest alkane available in-field multi point calibrations, n-tetradecane. Tetradecane calibration standards were introduced as low as 0.1 ng on column (translating to 0.25 ng m−3) and observed to be near the limit at which chromatographic peaks could no longer be reasonably integrated. Furthermore, calibrants present in blanks exhibit variability of ∼0.009 ng, and no sesquiterpene signal is observed in blanks. Limits of detection are consequently estimated to be roughly 0.25 ng m−3 (30 ppq) and concentrations below this threshold are reported as zero. Uncertainty for concentrations is given as 15%. Previous work has shown that the total ion response factor of tetradecane was determined using the quantification ion m/z 57 adjusted by the contribution of this quantification ion to the average mass spectrum, where the fraction of mass spectrum represented by the tetradecane quantification ion is fquant. Each sesquiterpene is integrated using the quantification ions corresponding to the most abundant unique m/z such as 93, 105, 119 or 161, adjusted by their fquant and quantified based on the total ion response factor of tetradecane. Analytes with fquant lower than 5% were quantified using 5% as a conservative estimate, or if available, assigned the fquant observed in the NIST library mass spectrum.
Data from both measurement sites was quantified using the response factors determined at the farm site. Due to hardware issues with the calibrant system at the tower site, multi-point calibrations were limited and exhibit substantial error. The response factors for sesquiterpene and tetradecane's fquant determined from the farm site reasonably describe the calibration data that is available from the forest site. At the start of each campaign, the MS was re-tuned to its baseline (maximum) sensitivity. Instrument sensitivity determined by MS tune files collected at the start of both measurement periods was similar, supporting similarity in the response factors between sites. At the farm site, long-term decay in instrument sensitivity due to the length of the sampling campaign (∼15% per week) was corrected using a monotonic, smoothed trend line calculated from observed changes in alkane sensitivity throughout the measurement period. This drift has been observed in previous work and arises from changes to the MS detector; a simple linear correction factor accounts for signal changes.50 Due to the short time-period of the forest site measurements and the scarcity of calibration information, no such drift correction was applied to these data, introducing a potential error of roughly 15%.
All reported observed sesquiterpenoid concentrations represent only those species that could be resolved and identified. To quantify the potential presence of unresolved sesquiterpenes and consequent unmeasured mass and reactivity, we also applied an alternative technique for chromatographic data analysis to estimate an upper bound of sesquiterpene concentrations. The mass spectral ion representing the molecular weight of C15H24 (m/z 204) was integrated across the full chromatographic range known to encompass the sesquiterpene compound class and calibrated using an average fquant based on spectra from NIST Mass Spectral Library. This approach has been previously used by both Chan, et al. and Isaacman-VanWertz, et al. and provides a robust estimate of concentrations for hydrocarbon chemical classes, even for those that suffer high degrees of mass spectral fragmentation such as sesquiterpenes.53,54 The method is most effective for samples containing primarily hydrocarbons, with increased uncertainty for compounds dominated by oxidized compounds or other heteroatom-containing functional groups. We consequently apply this approach only to data from the tower site, which is dominated by biogenic hydrocarbons, and do not extend it to data from the farm site, due to the presence of other species with m/z 204 in the sesquiterpene retention time window. Uncertainty in concentrations using the binned integration approach is estimated at roughly 30% based on the previous work.
Calculation of ozone and OH reactivity
Sesquiterpene reactivity with hydroxyl radicals (OHR) and ozone (O3R) is defined as the sum-product of the concentration (molecule per cm3) of each sesquiterpene, i, and oxidant specific reaction rate, k: | OxR (s−1) = ∑kOx+BVOCi[BVOCi] | (1) |
Rate constants (cm3 per molecule per s) are used from previous literature when available and are otherwise calculated from structure–activity relationships developed by Kwok and Atkinson as implemented by the Estimation Program Interface provided by the U.S. Environmental Protection Agency.5,55–57
C Results and discussion
Sesquiterpene composition and concentration
A total of 24 sesquiterpenoid species were observed in the gas phase during the two field campaigns, including 22 sesquiterpenes and two C15H22 sesquiterpenoids. Eleven of these compounds were identified at both sites including both C15H22 species (Fig. 1). Compounds observed at both sites accounted for similar fractions of total sesquiterpene mass at each site (Fig. 2), and less than 35% of total sesquiterpene mass is unique to each site. While the total contribution by shared isomers is nearly identical between sites, there are some differences in the composition, likely due to environmental and location factors discussed below. While compounds with lower hydroxyl reaction rate constants (kOH) appear to account for a larger amount of composition compared to compounds with higher reaction rate constants, the similarity in OH values across the observed sesquiterpene isomers makes such inferences difficult without further study. The similarity in composition between sites suggests a dominant group of compounds commonly emitted throughout the region, though with some differences likely due to external stress, growth cycle, and other factors that warrant further study.
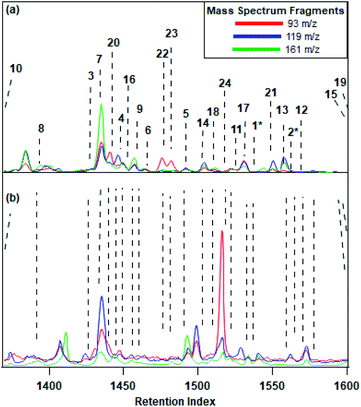 |
| Fig. 1 Sample chromatograms depicting identified sesquiterpenes at the tower (a) and farm (b) site. Compounds are labeled in order of increasing OH reaction rate constant and are as follows: (1) * cuparene, (2) * calamanene, (3) longifolene, (4) β-cedrene, (5) alloaromadendrene, (6) β-gurjunene, (7) α-cedrene, (8) α-copaene, (9) thujopsene, (10) α-cubebene, (11) β-selinene, (12) α-selinene, (13) γ-cadinene, (14) γ-muurolene, (15) γ-cuprenene, (16) α-bergamotene, (17) α-muurolene, (18) α-amorphene, (19) α-cadinene, (20) β-caryophyllene, (21) δ-cadinene, (22) azulene, (23) humulene, (24) farnesene. Sesquiterpenoids (C15H22) are denoted with an ‘*’ next to their number. α-Cubebene, γ-cuprenene, and α-cadinene are outside of the presented retention index. Ions common for sesquiterpenoids are different than those for sesquiterpenes and are not shown in the figure above, only their location relative to other sesquiterpenes. | |
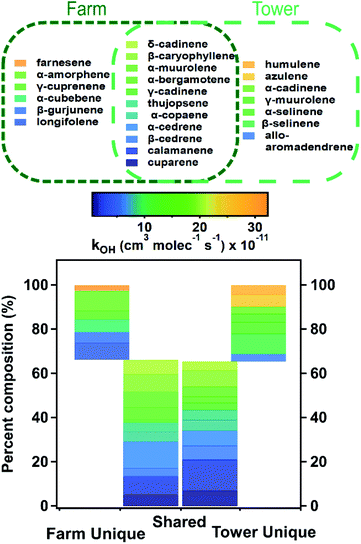 |
| Fig. 2 Percent composition of sesquiterpenes identified at the farm and tower sites with bars representing composition of sesquiterpenes identified at both sites and isomers identified solely at either the farm or the tower. Sesquiterpenes are colored according to increasing their rate constant for reaction with hydroxyl radicals. Specific values for kOH of each isomer are found in the ESI.† | |
At both sites, total sesquiterpene concentrations vary diurnally, with the maximum concentrations observed in the early morning (Fig. 3). Average total sesquiterpene concentration at the tower and farm sites were 22.1 ± 12.4 and 13.7 ± 6.5 ng m−3 (average ± standard deviation), with average nighttime concentrations (12–6 AM) of 31.8 ± 18.1 and 17.2 ± 13.7 ng m−3 at the tower and the farm respectively. Measured concentrations during both field deployments were found to fall within previously observed sesquiterpene concentrations, ranges of 0.1–38.4 ng m−3 (01–4.6 ppt).25,35,54,58 The upper bound estimates for sesquiterpene concentrations at the tower sites, calculated using binned integration of the molecular ion within a specific chromatographic window as described in the methods, is 28.8 ± 12.0 ng m−3 with a nighttime upper bound estimate of 31.4 ± 16.1 ng m−3. The agreement between the resolved and upper bound concentrations for the tower site indicates that while the presence of some unresolved sesquiterpenes cannot be ruled out, there does not appear to be some significant pool of sesquiterpenes not resolved by identified isomers. A similar upper bound analysis was not performed at the farm site due to the presence of several large peaks within the sesquiterpene region that represent oxygenated compounds but share some of the mass spectral fragments (e.g., the peak at 1520 s in the lower panel of Fig. 1); nevertheless we note that nearly all molecular ion signal in the sesquiterpene region of the farm data was qualitatively accounted for by resolved species and the known non-sesquiterpenes, suggesting the presence of little or no unresolved sesquiterpene mass.
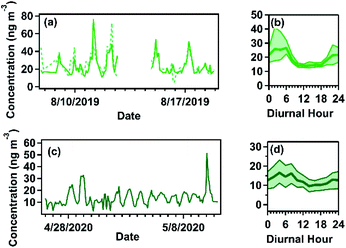 |
| Fig. 3 Concentrations of identified sesquiterpenes and sesquiterpenoids at the tower (a and b) and farm (c and d) sites. Timeseries of total concentrations are shown on the left (a and c) with diurnal behavior on the right (b and d).The dashed line in the tower time series represents the sesquiterpene concentrations estimated by unresolved binned integration as defined in the methods. | |
Differences in total concentration between sites may be attributed to a number of factors including location of sampling at the tower site, which occurred within the forest canopy and was consequently dominated by forest emissions. In contrast, the farm site was located further away from adjacent forest and sampling was influenced by emissions from the fields and isolated trees in addition to the forest. During transport from the forest, ambient ozone may react with sesquiterpenes before they reach the sample inlet.59 However, travel from the forest to the farm site is expected to occur on the timescale of minutes while atmospheric lifetime of sesquiterpenes is on the order of 10's–1000's of minutes. Consequently, while some sesquiterpenes may be lost due to reactivity, the losses will be relegated to small portions of the most reactive isomers. Sesquiterpene concentrations observed at the tower site can be surmised to be represent ambient forest concentrations due to the sample inlet location in the canopy while concentrations observed at the farm may be somewhat lower than those in the nearby forest due to reactive losses before reaching the sample inlet. Ozone scrubbers have been shown to trap other SVOC's and as such no scrubber was installed at either site, leading to the loss of a of sesquiterpenes trapped on the sample cell through surface assisted ozonolysis.54 Additionally, higher volatility sesquiterpenes (longifolene, α-cubebene) may not be adequately captured by the focusing trap, and consequently may be underestimated at both sites. Therefore, sesquiterpene measurements in this study should be considered as the lower limit measurements of ambient sesquiterpene concentrations.
Seasonal changes also play a role, as the farm site experienced cooler temperatures during sampling which occurred in late spring compared to late summer for the tower site. Temperature, light, and seasonal patterns have been shown to have a strong impact on BVOC emissions including sesquiterpenes.37,60–62 Generally, sesquiterpene emissions have been found to increase with temperature and emissions peak during the summer but dependance on these factors can vary. Observations of sesquiterpenes in the Amazon have shown a daily variation driven largely by temperature rather than temperature and light.60 The overall relation between emissions and temperature may arise due to lower sesquiterpene production observed from mid-fall to mid-spring and correlates with typically lower temperatures but the complexity of emissions appears to be greatest during spring.37,63 The degree to which sesquiterpene emissions correlate to seasonality and temperature dependance vary between species and even a small change in ecosystem composition could lead to changes in sesquiterpene composition; highlighting the need for greater analysis of the different species' emissions and influence of the variables described above.61 In fact, given the differences in season and proximity to the source, it is notable that the total concentration of shared isomers has such a high degree of similarity between the two experiments.
Sesquiterpene variation due to human agricultural activity
Agricultural activity took place at the farm site in the form of cutting several acres of hay field adjacent to the sampling location. This cutting occurred May 11th and 12th. Following this event, the sesquiterpene emissions profile changed drastically, and several other compounds were observed. Fig. 4, an early morning sesquiterpene profile shows the changes to the sesquiterpene emissions, in contrast to the representative sample shown in Fig. 1. Longifolene, observed at low intensities during the campaign, increased to the same relative intensity as α-cedrene, the usually dominant species. Many other sesquiterpene species identified at the farm site prior to the cutting were found to be either non-existent or below limits of detection after the cutting in addition to several non-sesquiterpenes not observed during the rest of the study. For example, several oxygenate species including methyl salicylate and trans-coumaric acid were observed in the early morning period for three days following the cutting. These compounds have been observed to be used as a defense mechanism against herbivore predation and indicate that the farm activity results in large changes to plant behavior. This change in sesquiterpene profile due to the influence of human agricultural activity illustrates the large impact anthropogenic or natural disruptions may have on emission profiles and supports the conclusion that transient events can significantly modulate the impact of sesquiterpenes, in addition to other VOCs, on local atmospheres.64–66 However, the data here provides only a small section of information, and calls to the need for an in-depth study of human agricultural activity and impact on BVOC emissions, particularly with regards to sesquiterpenes.
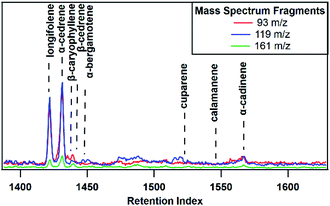 |
| Fig. 4 Sesquiterpene profile for the morning following hay cutting. Only species observed after hay cutting are identified. | |
Hydroxyl reactivity
The average calculated OH reactivity for the tower and farm sites were (7.17 ± 3.6) × 10−3 and (4.65 ± 2.0) × 10−3 s−1, respectively, with calculated nighttime OH reactivity of (11.3 ± 6.1) × 10−3 and (5.2 ± 4.0) × 10−3 s−1 for the tower and farm sites respectively. The difference in OH reactivity between the two sites (Fig. 5 and 6) is attributed to the increased sesquiterpene concentration at the tower site rather than differences in site profiles. The contribution of each species to reactivity is relatively evenly distributed between detected compounds (Fig. 5), which is expected due to the narrow range of sesquiterpene reaction rates, from 0.9–32 × 10−11 cm3 per molecule per s for species identified in this study (reaction rates provided in ESI†); sesquiterpenoids have somewhat lower reaction rates due to having fewer double bonds, but this effect has relatively little overall impact on the distribution of reactivity. A few highly reactive sesquiterpenes (humulene, farnesene, and δ-cadinene) do contribute an outsize amount of total reactivity despite their small concentrations, but the diversity of sesquiterpenes is nevertheless represented in their OH reactivity.
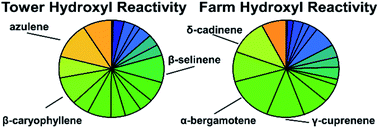 |
| Fig. 5 Composition weighted hydroxyl reactivity of sesquiterpene species identified and quantified at the tower and farm sites. The top three sesquiterpene contributors to hydroxyl reactivity for each site are listed next to their corresponding slice. | |
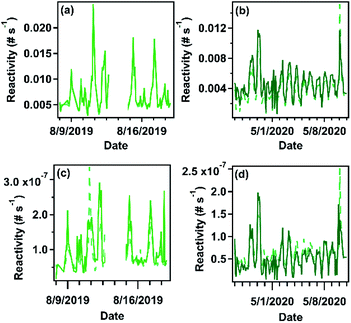 |
| Fig. 6 Time series of hydroxyl reactivity for the tower (a) and farm (b) sites as well as ozone reactivity time series for the tower (c) and farm (d) sites. Solid lines represent calculated reactivity using speciated sesquiterpenes while dashed lines represent reactivity based on average sesquiterpene reaction rates. | |
The observed hydroxyl-sesquiterpene reactivity is several orders of magnitude smaller than monoterpene and isoprene reactivity measured around the same period at the tower site. Literature values of isoprene and monoterpenes range from 1–21 s−1 and OH reactivity is shown to be as high as 32 s−1 at the tower site, largely driven by isoprene in warmer seasons.67,68 Most observed sesquiterpenes have similar reaction rate constants as monoterpenes compounds but are present at much lower concentrations, rendering sesquiterpene impact on OH reactivity negligible in comparison to other biogenic emissions. This agrees with a recent comparison between measured and calculated OH reactivity in the southeastern US that did not include sesquiterpenes, which found little to no “missing” reactivity, suggesting these higher molecular weight terpenoids do not contribute substantially to OH reactivity.58 This is likely to be generalizable across most ecosystems, as global emissions of sesquiterpenes are thought to be substantially lower than that of monoterpenes, and reaction rate constants with OH are not substantially faster.1
Ozone reactivity
In contrast to OH, reaction rate constants of sesquiterpenes with ozone are much faster than that of monoterpenes and can vary by several orders of magnitude (10−17 to 10−14 cm3 per molecule per s, reaction rates constants provided in ESI†). Consequently, both magnitude and composition of sesquiterpenes are expected to be important in considering total calculated ozone reactivity. Calculated average ozone-sesquiterpene reactivity for the tower and farm sites averaged 10.1 (±6.2) × 10−8 and 2.3 (±3.6) × 10−8 s−1. Nighttime (12–6 AM) reactivity for the tower and farm sites were 18.7 (±8.9) × 10−8 and 3.8 (±1.8) × 10−8 s−1. At both sites, the calculated sesquiterpene-ozone reactivity is dominated by β-caryophyllene and humulene, accounting for roughly three – quarters of the reactivity (Fig. 7, 83% and 69% for the tower and farm sites, respectively). This is due to β-caryophyllene and humulene's ozone reaction rate constant that are two orders of magnitude faster than most other sesquiterpenes. Even at their low abundance relative to other compounds (roughly 9% for β-caryophyllene at both sites), β-caryophyllene and humulene (roughly 5% at the tower site) represent the majority of sesquiterpene related ozone reactivity at the sites and other locales of the same representative ecosystem. The importance of such minor constituents of the compound class on the relative atmospheric impacts highlights the need for isomer-resolved measurements of these compounds and an understanding of their impacts.
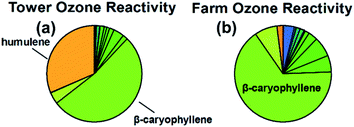 |
| Fig. 7 Composition weighted ozone reactivity of sesquiterpene species identified and quantified at the tower (a) and farm (b) sites. | |
Although sesquiterpenes are more reactive than other BVOC, their small concentrations result in uncertainty about how large a role they play in atmospheric chemistry. At the tower site, summertime BVOC ozone reactivity is on average 5 × 10−6 s−1, with concentrations of isoprene and monoterpenes in the ppb range, in contrast to ppt-level sesquiterpene (and ppq-level β-caryophyllene). This suggests that sesquiterpenes contribution to ozone reactivity is typically low (roughly a few percent at most), but it could become non-negligible during peak periods or during certain emission events. In particular, environmental factors resulting in enhanced emission of β-caryophyllene or other highly-reactive sesquiterpenes (e.g., herbivory) could significantly alter the ozone chemical loss in the local atmosphere.11,62,69 We note that the calculated ozone reaction rate constant for some sesquiterpenes is several orders of magnitude lower than experimentally determined values (e.g., β-caryophyllene, 4.4 × 10−16 compared to 110 × 10−16), so it is possible that the contribution to ozone reactivity of less-studied isomers (i.e., those without experimentally determined rate constants) could be significantly underestimated.
Average sesquiterpene reaction rates
Because of the scarcity and difficulty of ambient sesquiterpene measurements, there is rarely sufficient information regarding the composition of sesquiterpenes in an environment to properly model their atmospheric impacts. Here, we provide a calculated average reaction rate of the sesquiterpene mixture (i.e., kOx+SQTmix) that improves and simplifies modelling of this complex compound class. Oxidant rate constants for each site were calculated through linear regression of the calculated hourly total reactivity and the concentration of identified sesquiterpenes, yielding an average rate constant that bests converts concentration to total reactivity.51 The calculated hydroxyl-sesquiterpene rate constants were observed to be 11.4 × 10−11 and 11.5 × 10−11 cm3 per molecule per s at the tower and farm sites respectively; uncertainty in these values is similar to uncertainty in any speciated calculated reactivity and driven by uncertainty in concentrations measurements (∼15%), in addition to uncertainty in the calculated rate constants. Differences between calculated hydroxyl reactivity using speciated data versus the average rate constant with total concentration values are minimal, indicative of the relatively low variability of OH reaction rate constants between isomers. These average rate constants lie in the middle of the range of sesquiterpene-hydroxyl reaction rates are similar to the reaction rates of common monoterpenes, and are not substantially faster than the average reaction rate of monoterpenes with OH.51 Consequently, only high concentrations of sesquiterpenes comparable to other terpenoid classes would be able to significantly contribute to OH reactivity, and the contribution is likely low or negligible under most conditions. This is supported by previous work in the southeastern U.S. that leaves little room for a large contribution to OH reactivity by sesquiterpenes.58
The average ozone-sesquiterpene rate constants for the tower and farm sites were 17.5 × 10−16 and 18.5 × 10−16 cm3 per molecule per s, respectively. These average reaction rate are significantly faster than that of the typical monoterpenes observed at the site and an order of magnitude faster than the average reaction rate of monoterpenes with ozone (1.16 × 10−16),51 suggesting sesquiterpenes could contribute significantly to ozone reactivity compared to other compound classes under some conditions. These rate constants are highly influenced by β-caryophyllene's reactivity (in addition to humulene at the tower) despite β-caryophyllene representing less than 10% of total observed sesquiterpenes and humulene representing less than 5% of observed sesquiterpenes at the tower. It is worth noting that, although β-caryophyllene is, perhaps, the best studied sesquiterpene, using its ozone reaction rate as a proxy for all sesquiterpenes results in overestimating sesquiterpene ozone reactivity by an order of magnitude. As demonstrated by β-caryophyllene and humulene, the wide range of sesquiterpene reaction rates with ozone suggests that low-concentration sesquiterpenes, below resolvable levels of detection, could contribute non-negligibly to total ozone reactivity.51 Nevertheless, these results suggest that contributions by sesquiterpenes to total ozone reactivity in this ecosystem is small on average. In contrast to OH reactivity, the average ozone reaction rate is variable due to changes in composition. Calculated speciated reactivity can diverge from reactivity calculated using the average rate (Fig. 7), though deviations tend to be on orders of tens of percent and the calculated average rate constants serve well for approximating overall sesquiterpene-ozone reactivity. It should again be noted that, as illustrated by the change in sesquiterpene composition at the farm site during an anthropogenic influence event, particular events may have transient impacts on this mixture that could significantly change the average reaction rates and contributions to overall ozone reactivity could be non-negligible when concentrations are relatively high.
Owing to the high SOA yields of sesquiterpenes, this compound class is also often considered as a potential major contributor to organic aerosol. However, the low concentrations observed at this site indicate that sesquiterpenes will not contribute strongly to SOA mass downwind of this ecosystem; even complete conversion of sesquiterpenes to SOA (i.e., a yield of 100%) would generate only tens of nanograms per cubic meter of additional aerosol, on top of a typical aerosol concentration of micrograms per cubic meter. Consequently, the downwind SOA formation potential is consequently very low. While this complete conversion of sesquiterpenes to SOA is low, but this does not necessarily preclude sesquiterpenes playing a role in the aerosol processes or contributing significantly in other ecosystems. For example, previous studies have shown that sesquiterpenes represent large portions of the condensed phase of particles during specific events in some ecosystems, and can influence the local aerosol properties despite their trace concentrations.70 Sesquiterpenes could potentially influence the local aerosol properties despite their trace concentrations.71 Additionally, it is possible that highly reactive sesquiterpenes, such as farnesene or β-caryophyllene may be emitted at high rates and chemically depleted prior to the measurements made here, contributing substantially to SOA formation through rapid aerosol formation within the forest canopy and low measured concentrations, which has been observed for other environments.71 However, such a conclusion would not be supported by previous works where SOA composition has been more comprehensively measured in the southeastern US, in which organic aerosol could be explained almost completely by monoterpenes, isoprene, and other sources.29,35 No such comprehensive characterization of organic aerosol was available at this site to assess the prevalence of in-canopy aerosol formation from sesquiterpenes and future studies are needed to better assess the impact of sesquiterpenes on SOA formation and characteristics in this region of the southeastern US. The location of the measurements within the canopy would require such formation to occur very rapidly, so would depend on only the most reactive isomers, which may be probed by future analyses of oxidation products of these compounds. SV-TAG measured concentrations of potential oxidation products in the aerosol, but identification of individual components as sesquiterpene products is ongoing and non-trivial due to molecular structural features and fragments shared with monoterpenes.
Implications for the broader atmosphere
Better understanding of the composition and concentrations of sesquiterpenes is necessary to bridge the discrepancies between modeled and observed impacts of this compound class on atmospheric chemistry. Using a field deployable GC-MS (SV-TAG), concentrations of sesquiterpenes at two field sites in the southeastern U.S. were measured on the order of tens of ng m−3, and these data can provide several broader insights into the role of sesquiterpenes in the atmosphere. The similarity in composition observed at the two sites suggests the observed sesquiterpene mixture serve as a reasonable reaction rate proxy for the studied ecosystem in the southeastern U.S.
Due to their low relative concentrations and reaction rates compared to other major BVOC chemical classes, we conclude that sesquiterpenes under normal circumstances do not contribute significantly to total OH reactivity. This conclusion is expected to generally apply across environments, as the range of OH reaction rates for sesquiterpenes is not significantly different than that of monoterpenes and other more abundant biogenic compound classes. However, sesquiterpenes, in particular β-caryophyllene, may contribute non-negligibly to ozone reactivity due to their fast ozone reaction rates and may play a larger role under different environmental conditions. Notably, β-caryophyllene and humulene have experimental reaction rate constants that are approximately 150 times greater than that estimated by structure–activity relationships; if such a discrepancy were to exist for other sesquiterpenes, the contribution of the compound class to ozone reactivity would be significantly more important. We highlight a critical need for improved experimental data on the reactions of dominant sesquiterpenes to better constrain the impacts of the compound class.
We further present average reaction rates for the reaction of the total sesquiterpene class with common atmospheric oxidants, in combination with those estimated for monoterpenes by McGlynn et al., present an alternative approach to determine the potential impacts of terpene compounds to the local atmosphere.51 These reaction rate constants present a novel approach to accounting for sesquiterpene influence on tropospheric oxidant budget in addition to other situations that utilize modelling. Furthermore, they represent an effective approach to better understand and model the impacts of these compound classes when little or no speciated information is available. For example, this can be used in the case of emissions models with few sesquiterpene emission categories or measurements by direct-sampling mass spectrometers that cannot resolve compound classes by structure or isomer.1,72–74 This can provide a method for improving our understanding of sesquiterpene chemistry and better bridge the observations of sesquiterpenes in ambient conditions and those predicted by modelling. It is unlikely, however, that sesquiterpenes at present represent substantial SOA formation potential downwind of the emitting ecosystem (i.e., mixed forest) within the southeastern US, as even complete conversion would not generate significant aerosol. We cannot determine in this work whether or not rapid aerosol formation within the canopy contributes significantly to aerosol composition within the ecosystem as has been observed for other ecosystems, but the most likely interpretation of the present and previously published data is that sesquiterpenes simply do not contribute significantly to SOA concentrations in the southeastern US.71 in other environments, sesquiterpenes have been shown to contribute significantly to SOA particularly during certain episodes, suggesting a need for both expanded measurements of this compound class and associated oxidation products in addition to a better understanding of the drivers of the sesquiterpene emissions that may lead to intermittent or episodic periods of high sesquiterpene concentrations.71
Conflicts of interest
There are no conflicts to declare.
Acknowledgements
This research was funded by the National Science Foundation (AGS 1837882 and AGS 1837891). Tower maintenance and operation were supported in part by the Pace Endowment. Deborah F. McGlynn and Laura E. R. Barry were supported in part by Virginia Space Grant Consortium Graduate Research Fellowships. Graham Frazier was supported in part by the Edna Bailey Sussman Foundation Internship. We would like to thank the reviewers for help in strengthening the presentation of this paper. In particular, we would like to thank reviewer 3 for their advice on formatting of Fig. 2 to better display the data.
References
- A. B. Guenther, X. Jiang, C. L. Heald, T. Sakulyanontvittaya, T. Duhl, L. K. Emmons and X. Wang, The Model of Emissions of Gases and Aerosols from Nature Version 2.1 (MEGAN2.1): An Extended and Updated Framework for Modeling Biogenic Emissions, Geosci. Model Dev., 2012, 5(6), 1471–1492, DOI:10.5194/gmd-5-1471-2012.
- F. Fehsenfeld, J. Calvert, R. Fall, P. Goldan, A. B. Guenther, C. N. Hewitt, B. Lamb, S. Liu, M. Trainer, H. Westberg and P. Zimmerman, Emissions of Volatile Organic Compounds from Vegetation and the Implications for Atmospheric Chemistry, Global Biogeochem. Cycles, 1992, 6(4), 389–430, DOI:10.1029/92GB02125.
- A. Guenther, T. Karl, P. Harley, C. Wiedenmyer, P. I. Palmer and C. Geron, Estimates of Global Terrestrial Isoprene Emissions Using MEGAN (Model of Emissions of Gases and Aerosols from Nature), Atmos. Chem. Phys., 2007, 7(16), 4327, DOI:10.5194/acp-7-4327-2007.
- R. Atkinson and J. Arey, Gas-Phase Tropospheric Chemistry of Biogenic Volatile Organic Compounds: A Review, Atmos. Environ., 2003, 37, 197–219, DOI:10.1016/S1352-2310(03)00391-1.
- R. Atkinson, Gas-Phase Tropospheric Chemistry of Volatile Organic Compounds: 1. Alkanes and Alkenes, J. Phys. Chem. Ref. Data, 1997, 26(139), 215–1329, DOI:10.1063/1.4821165.
- J. H. Kroll and J. H. Seinfeld, Chemistry of Secondary Organic Aerosol: Formation and Evolution of Low-Volatility Organics in the Atmosphere, Atmos. Environ., 2008, 42(16), 3593–3624, DOI:10.1016/j.atmosenv.2008.01.003.
- K. C. Barsanti, J. H. Kroll and J. A. Thornton, Formation of Low-Volatility Organic Compounds in the Atmosphere: Recent Advancements and Insights, J. Phys. Chem. Lett., 2017, 8(7), 1503–1511, DOI:10.1021/acs.jpclett.6b02969.
- S. J. Oltmans, A. S. Lefohn, J. M. Harris, I. Galbally, H. E. Scheel, G. Bodeker, E. Brunke, H. Claude, D. Tarasick, B. J. Johnson, P. Simmonds, D. Shadwick, K. Anlauf, K. Hayden, F. Schmidlin, T. Fujimoto, K. Akagi, C. Meyer, S. Nichol, J. Davies, A. Redondas and E. Cuevas, Long-Term Changes in Tropospheric Ozone, Atmos. Environ., 2006, 40(17), 3156–3173, DOI:10.1016/j.atmosenv.2006.01.029.
- B. Bonn and G. K. Moortgat, Sesquiterpene Ozonolysis: Origin of Atmospheric New Particle Formation from Biogenic Hydrocarbons, Geophys. Res. Lett., 2003, 30(11), 1585, DOI:10.1029/2003GL017000.
- G. M. Wolfe, J. A. Thornton, M. McKay and A. H. Goldstein, Forest-Atmosphere Exchange of Ozone: Sensitivity to Very Reactive Biogenic VOC Emissions and Implications for in-Canopy Photochemistry, Atmos. Chem. Phys., 2011, 11(15), 7875–7891, DOI:10.5194/acp-11-7875-2011.
- C. L. Faiola, I. Pullinen, A. Buchholz, F. Khalaj, A. Ylisirniö, E. Kari, P. Miettinen, J. K. Holopainen, M. Kivimäenpää, S. Schobesberger, T. Yli-Juuti and A. Virtanen, Secondary Organic Aerosol Formation from Healthy and Aphid-Stressed Scots Pine Emissions, ACS Earth Space Chem., 2019, 3(9), 1756–1772, DOI:10.1021/acsearthspacechem.9b00118.
- E. Ormeño, D. R. Gentner, S. Fares, J. Karlik, J. H. Park and A. H. Goldstein, Sesquiterpenoid Emissions from Agricultural Crops: Correlations to Monoterpenoid Emissions and Leaf Terpene Content, Environ. Sci. Technol., 2010, 44(10), 3758–3764, DOI:10.1021/es903674m.
- E. Ormeño, J. P. Mévy, B. Vila, A. Bousquet-Mélou, S. Greff, G. Bonin and C. Fernandez, Water Deficit Stress Induces Different Monoterpene and Sesquiterpene Emission Changes in Mediterranean Species. Relationship
between Terpene Emissions and Plant Water Potential, Chemosphere, 2007, 67(2), 276–284, DOI:10.1016/j.chemosphere.2006.10.029.
- Ü. Niinemets, A. Kännaste and L. Copolovici, Quantitative Patterns between Plant Volatile Emissions Induced by Biotic Stresses and the Degree of Damage, Front. Plant Sci., 2013, 4(262), 1–15, DOI:10.3389/fpls.2013.00262.
- E. Bourtsoukidis, B. Bonn, A. Dittmann, H. Hakola, H. Hellén and S. Jacobi, Ozone Stress as a Driving Force of Sesquiterpene Emissions: A Suggested Parameterisation, Biogeosciences, 2012, 9(11), 4337–4352, DOI:10.5194/bg-9-4337-2012.
- A. Tani and T. Mochizuki, Review: Exchanges of Volatile Organic Compounds between Terrestrial Ecosystems and the Atmosphere, J. Agric. Meteorol., 2021, 77(1), 66–80, DOI:10.2480/agrmet.D-20-00025.
- M. Lerdau and L. Slobodkin, Trace Gas Emissions and Species-Dependent Ecosystem Services, Trends Ecol. Evol., 2002, 17(7), 309–312, DOI:10.1016/S0169-5347(02)02535-1.
- M. Lerdau, A. Guenther and R. Monson, Plant Production and Emission of Volatile Organic Compounds: Plant-Produced Hydrocarbons Influence Not Only the Plant Itself but the Atmosphere a Well, BioScience, 1997, 47(6), 373–383, DOI:10.2307/1313152.
- D. Helmig, J. Ortega, T. Duhl, D. Tanner, A. Guenther, P. Harley, C. Wiedinmyer, J. Milford and T. Sakulyanontvittaya, Sesquiterpene Emissions from Pine Trees – Identifications, Emission Rates and Flux Estimates for the Contiguous United States, Environ. Sci. Technol., 2007, 41(5), 1545–1553, DOI:10.1021/es0618907.
- M. Jaoui, T. E. Kleindienst, K. S. Docherty, M. Lewandowski and J. H. Offenberg, Secondary Organic Aerosol Formation from the Oxidation of a Series of Sesquiterpenes: α-Cedrene, β-Caryophyllene, α-Humulene and α-Farnesene with O3, OH and NO3 Radicals, Environ. Chem., 2013, 10(3), 178–193, DOI:10.1071/EN13025.
- A. Lee, A. H. Goldstein, J. H. Kroll, N. L. Ng, V. Varutbangkul, R. C. Flagan and J. H. Seinfeld, Gas-Phase Products and Secondary Aerosol Yields from the Photooxidation of 16 Different Terpenes, J. Geophys. Res.: Atmos., 2006, 111(17), 17305, DOI:10.1029/2006JD007050.
- S. Richters, H. Herrmann and T. Berndt, Gas-Phase Rate Coefficients of the Reaction of Ozone with Four Sesquiterpenes at 295+- 2 K, Phys. Chem. Chem. Phys., 2015, 17, 11658, 10.1039/c4cp05542j.
- J. Pollmann, J. Ortega and D. Helmig, Analysis of Atmospheric Sesquiterpenes: Sampling Losses and Mitigation of Ozone Interferences, Environ. Sci. Technol., 2005, 39(24), 9620–9629, DOI:10.1021/es050440w.
- K. Mermet, S. Sauvage, S. Dusanter, T. Salameh, T. Léonardis, P. M. Flaud, E. Perraudin, E. Villenave and N. Locoge, Optimization of a Gas Chromatographic Unit for Measuring Biogenic Volatile Organic Compounds in Ambient Air, Atmos. Meas. Tech., 2019, 12(11), 6153–6171, DOI:10.5194/amt-12-6153-2019.
- L. D. Yee, G. Isaacman-VanWertz, R. A. Wernis, M. Meng, V. Rivera, N. M. Kreisberg, S. V. Hering, M. S. Bering, M. Glasius, M. A. Upshur, A. Gray Bé, R. J. Thomson, F. M. Geiger, J. H. Offenberg, M. Lewandowski, I. Kourtchev, M. Kalberer, S. De Sá, S. T. Martin, M. L. Alexander, B. B. Palm, W. Hu, P. Campuzano-Jost, D. A. Day, J. L. Jimenez, Y. Liu, K. A. McKinney, P. Artaxo, J. Viegas, A. Manzi, M. B. Oliveira, R. De Souza, L. A. T. Machado, K. Longo and A. H. Goldstein, Observations of Sesquiterpenes and Their
Oxidation Products in Central Amazonia during the Wet and Dry Seasons, Atmos. Chem. Phys., 2018, 18(14), 10433–10457, DOI:10.5194/acp-18-10433-2018.
- C. L. Faiola, A. Buchholz, E. Kari, P. Yli-Pirilä, J. K. Holopainen, M. Kivimäenpää, P. Miettinen, D. R. Worsnop, K. E. J. Lehtinen, A. B. Guenther and A. Virtanen, Terpene Composition Complexity Controls Secondary Organic Aerosol Yields from Scots Pine Volatile Emissions, Sci. Rep., 2018, 8(1), 3053, DOI:10.1038/s41598-018-21045-1.
- L. Xu, H. O. T. Pye, J. He, Y. Chen, B. N. Murphy and N. L. Ng, Experimental and Model Estimates of the Contributions from Biogenic Monoterpenes and Sesquiterpenes to Secondary Organic Aerosol in the Southeastern United States, Atmos. Chem. Phys., 2018, 18(17), 12613–12637, DOI:10.5194/acp-18-12613-2018.
- T. Sakulyanontvittaya, A. Guenther, D. Helmig, J. Milford and C. Wiedinmyer, Secondary Organic Aerosol from Sesquiterpene and Monoterpene Emissions in the United States, Environ. Sci. Technol., 2008, 42(23), 8784–8790, DOI:10.1021/es800817r.
- B. H. Lee, E. L. D'Ambro, F. D. Lopez-Hilfiker, S. Schobesberger, C. Mohr, M. A. Zawadowicz, J. Liu, J. E. Shilling, W. Hu, B. B. Palm, J. L. Jimenez, L. Hao, A. Virtanen, A. H. Goldstein, H. O. T. Pye and J. A. Thornton, Resolving Ambient Organic Aerosol Formation and Aging Pathways with Simultaneous Molecular Composition and Volatility Observations, ACS Earth Space Chem., 2020, 4(3), 391–402, DOI:10.1021/acsearthspacechem.9b00302.
- T. Sakulyanontvittaya, A. Guenther, D. Helmig, J. Milford and C. Wiedinmyer, Secondary Organic Aerosol from Sesquiterpene and Monoterpene Emissions in the United States, Environ. Sci. Technol., 2008, 42(23), 8784–8790, DOI:10.1021/es800817r.
- W. Vizuete, V. Junquera and D. T. Allen, Sesquiterpene Emissions and Secondary Organic Aerosol Formation Potentials for Southeast Texas, Aerosol Sci. Technol., 2004, 38(sup1), 167–181, DOI:10.1080/02786820390229480.
- K. Jardine, A. Yañez Serrano, A. Arneth, L. Abrell, A. Jardine, J. Van Haren, P. Artaxo, L. V. Rizzo, F. Y. Ishida, T. Karl, J. Kesselmeier, S. Saleska and T. Huxman, Within-Canopy Sesquiterpene Ozonolysis in Amazonia, J. Geophys. Res.: Atmos., 2011, 116, D19301, DOI:10.1029/2011JD016243.
- A. M. Winer, J. Arey, R. Atkinson, S. M. Aschmann, W. D. Long, C. L. Morrison and D. M. Olszyk, Emission Rates of Organics from Vegetation in California's Central Valley, Atmos. Environ., Part A, 1992, 26(14), 2647–2659, DOI:10.1016/0960-1686(92)90116-3.
- H. Hellén, S. Schallhart, A. P. Praplan, T. Tykkä, M. Aurela, A. Lohila, A. Lohila and H. Hakola, Sesquiterpenes Dominate Monoterpenes in Northern Wetland Emissions, Atmos. Chem. Phys., 2020, 20(11), 7021–7034, DOI:10.5194/acp-20-7021-2020.
- N. C. Bouvier-Brown, A. H. Goldstein, J. B. Gilman, W. C. Kuster and J. A. De Gouw, In-Situ Ambient Quantification of Monoterpenes, Sesquiterpenes and Related Oxygenated Compounds during BEARPEX 2007: Implications for Gas- And Particle-Phase Chemistry, Atmos. Chem. Phys., 2009, 9(15), 5505–5518, DOI:10.5194/acp-9-5505-2009.
- D. Kim, P. S. Stevens and R. A. Hites, Rate Constants for the Gas-Phase Reactions of OH and O 3 with β-Ocimene, β-Myrcene, and α-and β-Farnesene as a Function of Temperature, J. Phys. Chem. A, 2011, 115(4), 500–506, DOI:10.1021/jp111173s.
- C. Holzke, T. Hoffmann, L. Jaeger, R. Koppmann and W. Zimmer, Diurnal and Seasonal Variation of Monoterpene and Sesquiterpene Emissions from Scots Pine (Pinus Sylvestris L.), Atmos. Environ., 2006, 40(17), 3174–3185, DOI:10.1016/j.atmosenv.2006.01.039.
- F. Weikl, A. Ghirardo, J. P. Schnitzler and K. Pritsch, Sesquiterpene Emissions from Alternaria Alternata and Fusarium Oxysporum: Effects of Age, Nutrient Availability, and Co-Cultivation, Sci. Rep., 2016, 6(1), 1–12, DOI:10.1038/srep22152.
- S. Fares, D. R. Gentner, J. H. Park, E. Ormeno, J. Karlik and A. H. Goldstein, Biogenic Emissions from Citrus Species in California, Atmos. Environ., 2011, 45(27), 4557–4568, DOI:10.1016/J.ATMOSENV.2011.05.066.
- F. Chen, H. Al-Ahmad, B. Joyce, N. Zhao, T. G. Köllner, J. Degenhardt and C. N. Stewart, Within-Plant Distribution and Emission of Sesquiterpenes from Copaifera Officinalis, Plant Physiol. Biochem., 2009, 47(11–12), 1017–1023, DOI:10.1016/j.plaphy.2009.07.005.
- J. Arey, A. M. Winer, R. Atkinson, S. M. Aschmann, W. D. Long, C. L. Morrison and D. M. Olszyk, Terpenes Emitted from Agricultural Species Found in California's Central Valley, J. Geophys. Res., 1991, 96(D5), 9329, DOI:10.1029/91JD00447.
- C. Hogrefe, S. S. Isukapalli, X. Tang, P. G. Georgopoulos, S. He, E. E. Zalewsky, W. Hao, J. Y. Ku, T. Key and G. Sistla, Impact of Biogenic Emission Uncertainties on the Simulated Response of Ozone and Fine Particulate Matter to Anthropogenic Emission Reductions, J. Air Waste Manage. Assoc., 2011, 61(1), 92–108, DOI:10.3155/1047-3289.61.1.92.
- Y. Zhao, N. M. Kreisberg, D. R. Worton, A. P. Teng, S. V. Hering and A. H. Goldstein, Development of an In Situ Thermal Desorption Gas Chromatography Instrument for Quantifying Atmospheric Semi-Volatile Organic Compounds, Aerosol Sci. Technol., 2013, 47(3), 258–266, DOI:10.1080/02786826.2012.747673.
- N. M. Kreisberg, D. R. Worton, Y. Zhao, G. Isaacman, A. H. Goldstein and S. V. Hering, Development of an Automated High Temperature Valveless Injection System for On-Line Gas Chromatography, Atmos. Meas. Tech. Discuss., 2014, 7, 7531–7567, DOI:10.5194/amtd-7-7531-2014.
- G. Isaacman, N. M. Kreisberg, L. D. Yee, D. R. Worton, A. W. H. Chan, J. A. Moss, S. V. Hering and A. H. Goldstein, Online Derivatization for Hourly Measurements of Gas- and Particle-Phase Semi-Volatile Oxygenated Organic Compounds by Thermal Desorption Aerosol Gas Chromatography (SV-TAG), Atmos. Meas. Tech., 2014, 7(12), 4417–4429, DOI:10.5194/amt-7-4417-2014.
- B. L. Deming, D. Pagonis, X. Liu, D. A. Day, R. Talukdar, J. E. Krechmer, J. A. De Gouw, J. L. Jimenez and P. J. Ziemann, Measurements of Delays of Gas-Phase Compounds in a Wide Variety of Tubing Materials Due to Gas-Wall Interactions, Atmos. Meas. Tech., 2019, 12(6), 3453–3461, DOI:10.5194/amt-12-3453-2019.
- D. Pagonis, J. E. Krechmer, J. De Gouw, J. L. Jimenez and P. J. Ziemann, Effects of Gas-Wall Partitioning in Teflon Tubing and Instrumentation on Time-Resolved Measurements of Gas-Phase Organic Compounds, Atmos. Meas. Tech., 2017, 10, 4687–4696, DOI:10.5194/amt-10-4687-2017.
- Y. Zhao, N. M. Kreisberg, D. R. Worton, G. Isaacman, R. J. Weber, S. Liu, D. A. Day, L. M. Russell, M. Z. Markovic, T. C. Vandenboer, J. G. Murphy, S. V. Hering and A. H. Goldstein, Insights into Secondary Organic Aerosol Formation Mechanisms from Measured Gas/Particle Partitioning of Specific Organic Tracer Compounds, Environ. Sci. Technol., 2013, 47(8), 3781–3787, DOI:10.1021/es304587x.
- G. Isaacman, N. M. Kreisberg, D. R. Worton, S. V. Hering and A. H. Goldstein, A Versatile and Reproducible Automatic Injection System for Liquid Standard Introduction: Application to in-Situ Calibration, Atmos. Meas. Tech., 2011, 4(9), 1937–1942, DOI:10.5194/AMT-4-1937-2011.
- N. M. Kreisberg, S. V. Hering, B. J. Williams, D. R. Worton and A. H. Goldstein, Quantification of Hourly Speciated Organic Compounds in Atmospheric Aerosols, Measured by an In-Situ Thermal Desorption Aerosol Gas Chromatograph (TAG), Aerosol Sci. Technol., 2009, 43(1), 38–52, DOI:10.1080/02786820802459583.
- D. McGlynn, L. Barry, M. Lerdau, S. Pusede and G. Isaacman-VanWertz, Measurement Report: Variability in the Composition of Biogenic Volatile Organic Compounds in a Southeastern US Forest and Their Role in Atmospheric Reactivity, Atmos. Chem. Phys. Discuss., 2021, 1–20, DOI:10.5194/acp-2021-416.
- G. Isaacman-VanWertz, D. T. Sueper, K. C. Aikin, B. M. Lerner, J. B. Gilman, J. A. de Gouw, D. R. Worsnop and A. H. Goldstein, Automated Single-Ion Peak Fitting as an Efficient Approach for Analyzing Complex Chromatographic Data, J. Chromatogr. A, 2017, 1529, 81–92, DOI:10.1016/j.chroma.2017.11.005.
- G. Isaacman-Vanwertz, X. Lu, E. Weiner, E. Smiley and M. Widdowson, Characterization of Hydrocarbon Groups in Complex Mixtures Using Gas Chromatography with Unit-Mass Resolution Electron Ionization Mass Spectrometry, Anal. Chem., 2020, 92(18), 12481–12488, DOI:10.1021/ACS.ANALCHEM.0C02308.
- A. W. H. Chan, N. M. Kreisberg, T. Hohaus, P. Campuzano-Jost, Y. Zhao, D. A. Day, L. Kaser, T. Karl, A. Hansel, A. P. Teng, C. R. Ruehl, D. T. Sueper, J. T. Jayne, D. R. Worsnop, J. L. Jimenez, S. V. Hering and A. H. Goldstein, Speciated Measurements of Semivolatile and Intermediate Volatility Organic Compounds (S/IVOCs) in a Pine Forest during BEACHON-RoMBAS 2011, Atmos. Chem. Phys. Discuss., 2015, 15(16), 22331–22377, DOI:10.5194/acpd-15-22331-2015.
- R. Atkinson, D. L. Baulch, R. A. Cox, R. F. Hampson, J. A. Kerr, M. J. Rossi and J. Troe, Evaluated Kinetic and Photochemical Data for Atmospheric Chemistry, Organic Species: Supplement VII, J. Phys. Chem. Ref. Data, 1999, 28(2), 191–393, DOI:10.1063/1.556048.
- E. S. C. Kwok and R. Atkinson, Estimation of Hydroxyl Radical Reaction Rate Constants for Gas-Phase Organic Compounds Using a Structure-Reactivity Relationship: An Update, Atmos. Environ., 1995, 29(14), 1685–1695, DOI:10.1016/1352-2310(95)00069-B.
- M. D. King, C. E. Canosa-Mas and R. P. Wayne, A Structure-Activity Relationship (SAR) for Predicting Rate Constants for the Reaction of NO3, OH and O3 with Monoalkenes and Conjugated Dienes, Phys. Chem. Chem. Phys., 1999, 1(9), 2239–2246, 10.1039/a901193e.
- C. L. Heald, J. De Gouw, A. H. Goldstein, A. B. Guenther, P. L. Hayes, W. Hu, G. Isaacman-Vanwertz, J. L. Jimenez, F. N. Keutsch, A. R. Koss, P. K. Misztal, B. Rappenglück, J. M. Roberts, P. S. Stevens, R. A. Washenfelder, C. Warneke and C. J. Young, Contrasting Reactive Organic Carbon Observations in the Southeast United States (SOAS) and Southern California (CalNex), Environ. Sci. Technol., 2020, 54(23), 14923–14935, DOI:10.1021/acs.est.0c05027.
- K. Jardine, A. Yañez Serrano, A. Arneth, L. Abrell, A. Jardine, J. Van Haren, P. Artaxo, L. V. Rizzo, F. Y. Ishida, T. Karl, J. Kesselmeier, S. Saleska and T. Huxman, Within-Canopy Sesquiterpene Ozonolysis in Amazonia, J. Geophys. Res.: Atmos., 2011, 116(D19), 19301, DOI:10.1029/2011JD016243.
- E. G. Alves, K. Jardine, J. Tota, A. Jardine, A. Maria Yãnez-Serrano, T. Karl, J. Tavares, B. Nelson, D. Gu, T. Stavrakou, S. Martin, P. Artaxo, A. Manzi and A. Guenther, Seasonality of Isoprenoid Emissions from a Primary Rainforest in Central Amazonia, Atmos. Chem. Phys., 2016, 16(6), 3903–3925, DOI:10.5194/acp-16-3903-2016.
- T. R. Duhl, D. Helmig and A. Guenther, Sesquiterpene Emissions from Vegetation: A Review, Biogeosciences, 2008, 5(3), 761–777, DOI:10.5194/bg-5-761-2008.
- U. Hansen and G. Seufert, Temperature and Light Dependence of β-Caryophyllene Emission Rates, J. Geophys. Res.: Atmos., 2003, 108, 4801, DOI:10.1029/2003JD003853.
- C. D. Geron and R. R. Arnts, Seasonal Monoterpene and Sesquiterpene Emissions from Pinus Taeda and Pinus Virginiana, Atmos. Environ., 2010, 44(34), 4240–4251, DOI:10.1016/j.atmosenv.2010.06.054.
- C. Faiola and D. Taipale, Impact of Insect Herbivory on Plant Stress Volatile Emissions from Trees: A Synthesis of Quantitative Measurements and Recommendations for Future Research, Atmos. Environ.: X, 2020, 5, 100060, DOI:10.1016/j.aeaoa.2019.100060.
- J. A. De Gouw, C. J. Howard, T. G. Custer and R. Fall, Emissions of Volatile Organic Compounds from Cut Grass and Clover Are Enhanced during the Drying Process, Geophys. Res. Lett., 1999, 26(7), 811–814, DOI:10.1029/1999GL900076.
- J. de Grouw and C. J. Howard, Proton-Transfer Chemical-Ionization Mass Spectrometry Allows Real-Time Analysis of Volatile Organic Compounds Released from Cutting and Drying of Crops, Environ. Sci. Technol., 2000, 34, 2640–2648, DOI:10.1021/es991219k.
- Y. Nakashima, S. Kato, J. Greenberg, P. Harley, T. Karl, A. Turnipseed, E. Apel, A. Guenther, J. Smith and Y. Kajii, Total OH Reactivity Measurements in Ambient Air in a Southern Rocky Mountain Ponderosa Pine Forest during BEACHON-SRM08 Summer Campaign, Atmos. Environ., 2014, 85, 1–8, DOI:10.1016/j.atmosenv.2013.11.042.
- S. Ramasamy, A. Ida, C. Jones, S. Kato, H. Tsurumaru, I. Kishimoto, S. Kawasaki, Y. Sadanaga, Y. Nakashima, T. Nakayama, Y. Matsumi, M. Mochida, S. Kagami, Y. Deng, S. Ogawa, K. Kawana and Y. Kajii, Total OH Reactivity Measurement in a BVOC Dominated Temperate Forest during a Summer Campaign, 2014, Atmos. Environ., 2016, 131, 41–54, DOI:10.1016/j.atmosenv.2016.01.039.
- R. N. Kigathi, S. B. Unsicker, M. Reichelt, J. Kesselmeier, J. Gershenzon and W. W. Weisser, Emission of Volatile Organic Compounds after Herbivory from Trifolium Pratense (L.) under Laboratory and Field Conditions, J. Chem. Ecol., 2009, 35(11), 1335–1348, DOI:10.1007/s10886-009-9716-3.
- D. F. Zhao, A. Buchholz, R. Tillmann, E. Kleist, C. Wu, F. Rubach, A. Kiendler-Scharr, Y. Rudich, J. Wildt and T. F. Mentel, Environmental Conditions Regulate the Impact of Plants on Cloud Formation, Nat. Commun., 2017, 8(1), 1–8, DOI:10.1038/ncomms14067.
- L. M. F. Barreira, A. Ylisirniö, I. Pullinen, A. Buchholz, Z. Li, H. Lipp, H. Junninen, U. Hõrrak, S. M. Noe, A. Krasnova, D. Krasnov, K. Kask, E. Talts, Ü. Niinemets, J. Ruiz-Jimenez and S. Schobesberger, The Importance of Sesquiterpene Oxidation Products for Secondary Organic Aerosol Formation in a Springtime Hemiboreal Forest, Atmos. Chem. Phys., 2021, 21(15), 11781–11800, DOI:10.5194/ACP-21-11781-2021.
- S. Kim, T. Karl, D. Helmig, R. Daly, R. Rasmussen and A. Guenther, Measurement of Atmospheric Sesquiterpenes by Proton Transfer Reaction-Mass Spectrometry (PTR-MS), Atmos. Meas. Tech., 2009, 2(1), 99–112, DOI:10.5194/amt-2-99-2009.
- S. Kim, T. Karl, A. Guenther, G. Tyndall, J. Orlando, P. Harley, R. Rasmussen and E. Apel, Emissions and Ambient Distributions of Biogenic Volatile Organic Compounds (BVOC) in a Ponderosa Pine Ecosystem: Interpretation of PTR-MS Mass Spectra, Atmos. Chem. Phys., 2010, 10(4), 1759–1771, DOI:10.5194/acp-10-1759-2010.
- H. Li, M. Riva, P. Rantala, L. Heikkinen, K. Daellenbach, J. E. Krechmer, P. M. Flaud, D. Worsnop, M. Kulmala, E. Villenave, E. Perraudin, M. Ehn and F. Bianchi, Terpenes and Their Oxidation Products in the French Landes Forest: Insights from Vocus PTR-TOF Measurements, Atmos. Chem. Phys., 2020, 20(4), 1941–1959, DOI:10.5194/acp-20-1941-2020.
|
This journal is © The Royal Society of Chemistry 2022 |