Plasmon-assisted facile selective gaseous isopropanol dehydrogenation over Ag nanocubes
Received
12th August 2021
, Accepted 25th October 2021
First published on 17th November 2021
Abstract
In the current research, monodisperse Ag nanocubes are synthesized and characterized by X-ray diffraction (XRD), field-emission scanning electron microscopy (FE-SEM), field-emission transmittance electron microscopy (FE-TEM), X-ray photoelectron spectroscopy (XPS), and UV-vis photospectrometry. It is seen that isopropanol can be dehydrogenated to acetone over Ag nanocubes in high selectivity at near-room temperatures. Light illumination leads to an increase in the isopropanol dehydrogenation efficiency without decreasing the selectivity. The effects of reaction temperatures, light wavelengths, light intensities, and sizes of the Ag nanocubes on the catalytic yields are investigated in detail. The result reveals that plasmonic heating has a low contribution to the light-induced increase of the catalytic activity, which could be ascribed to a plasmonic non-thermal effect. It is seen that the dehydrogenation activity is dependent on α-hydrogen activation. As compared to methanol, ethanol, and N-propanol, isopropanol presents the highest light-induced catalytic activity because of the most active α-hydrogens. It is considered that the hot electron transfer from the Ag nanocubes to molecular O2 accelerates oxygen activation for isopropanol dehydrogenation.
1. Introduction
Efficient use of visible light in photocatalytic transformations has become more and more attractive in recent years.1–3 Plasmon photocatalysis, driven by the localized surface plasmon resonance (LSPR), is a significant pathway. LSPR occurs in a collective oscillation of the surface conductance electrons in metallic nanostructures with high free-electron mobility.4,5 LSPR loses resonance coherence through either non-irradiative or non-irradiative decay. Non-irradiative decay will result in hot electrons and holes that can further react with molecule absorbers.6–8 The direct decay can deposit the energy into the anti-orbitals of adsorbed molecules and cause selective catalysis.9–11 It has been reported that LSPR decay can cause many catalytic effects, such as H2 dissociation,12,13 O2 dissociation,14 selective oxidation,7,15–18 selective hydrogenation,15 water splitting,19–22 CO2 reduction,23–25 NH3 decomposition,26 and organic deep oxidation.27–29
An important advantage of plasmonic photocatalysis is that the absorption light wavelength can be tuned;30 this allows us to deposit specified energies into absorbed molecules for selective transformations.31 The plasmonic absorption of Au, Ag, and Cu nanostructures, which locate in the visible region, can also be shifted to the near-infrared region, so the whole spectrum of solar light can be used.32 Strong field enhancement can also occur at surface vicinities, the corners and along the edges;33 this can result in a non-uniform distribution of the charge carriers and hot spots over metal nanoparticles. Plasmonic photocatalysis is thus dependent on the nanoparticle shape.32 For example, Linic et al. studied the photocatalytic degradation of methylene blue over Ag nanocubes, Ag nanospheres, and Ag nanofibers; they found that Ag nanocubes offered the highest photocatalytic ability,34 and they also saw that Ag nanocubes can be used for ethylene epoxidation near 200 °C under light illumination.7,30–32
Metallic Ag is a catalyst for converting carbonyls from alcohols by dehydrogenation at relatively high temperatures and high pressures. Ag nanoparticles can be used for the hydrogenation of many alcohols under facile conditions;35–39 these studies mainly focused on thermocatalytic conversions. For example, Mourhly et al. studied propanol (IPA) catalysis over Ag nanoparticles/mesoporous SiO2 under gaseous conditions without light illumination and found that IPA could be dehydrogenated to acetone with high efficiency and selectivity.39 Rana et al. found that bioethanol could be dehydrogenated to acetaldehyde in ∼90% selectivity by Ag nanoparticles/CeO2 in the dark.35 Liu et al. observed that benzyl alcohol can be dehydrogenated to benzaldehyde in more than 90% yield by Ag nanoparticles/Mg–Al oxides in the absence of oxidants in the dark.37 Others also studied the effect of light illumination on alcohol hydrogenation over Ag nanoparticles supported on wide-gap semiconductors, such as ZnO and TiO2.40–42 A recent review summarized the selective oxidation of alcohols over Ag-based catalysts.38
However, there were few studies on the role of light illumination in alcohol dehydrogenation over Ag nanocubes. In a recent research, it was shown that phenyl alcohol could be converted to phenyl carbonyl over Ag nanocubes under solution conditions at low temperatures.15 The current research investigates plasmon-assisted IPA dehydrogenation over Ag nanocubes under gaseous conditions. It is seen that the non-thermal effect of the plasmonic absorption causes an obvious increase in the catalytic activity. It is also found that the dehydrogenation could be dependent on α-hydrogen activity. Compared to methanol, ethanol, and N-propanol, Ag nanocubes present the highest catalytic activity for IPA dehydrogenation at near-room temperatures in high selectivity. It is proposed that hot electron transfer is responsible for molecular O2 activation that causes the aerobic isopropanol dehydrogenation.
2. Experimental
2.1. Synthesis of Ag nanocubes
Ag nanocubes were prepared according to a literature method.43 100 ml of ethylene glycol (EG) was firstly loaded in a single-neck flask immersed in an oil bath and was heated to 150 °C. 0.24 mL of NaHS EG solution was then quickly added. 2 min later, 2 mL of chloride acid and 5 mL of 0.36 mM polyvinyl pyrrolidone (PVP) EG solution were added slowly. Another 2 min later, 1.6 ml of CF3COOAg EG solution was also added slowly. The flask was sealed with a glass stopper, and the reaction was continued for 20 and 50 min to obtain small and large sizes of Ag nanocubes, respectively. Finally, the flask was taken out and immersed in an ice water bath quickly. The reacted solutions were mixed with acetone and stirred for several minutes and further centrifuged at 8000 rpm to obtain the Ag nanocube sediments. The sediments were then mixed with deionized water and ultrasonically washed for 10 min to dissolve the PVP polymers over the Ag nanocube surfaces, which were then centrifuged to obtain the Ag nanocube sediment. The above procedure was repeated several times. The final product was dispersed in ethanol for further characterization.
2.2. Characterization
The morphologies of the Ag nanocubes were checked using a field emission scanning electron microscope operating at 5 kV (FE-SEM; type: S-4800, Hitachi, Tokyo, Japan). Transmission electron microscope images of the Ag nanocubes were taken with a field-emission transmission electron microscope (FE-TEM, type: JEM2100F, JEOL, Tokyo, Japan) operating at 200 kV. X-ray diffraction (XRD) patterns were collected with an X-ray diffractometer (XRD; Empyrean, PANalytical, Almelo, Netherlands) at the operating voltage and current of 40 kV and 40 mA, respectively, using CuKα radiation as the X-ray source. The surface chemical compositions of the Ag nanocubes were checked with an X-ray photoelectron spectrometer (XPS; type: VG Multilab 2000, Thermo Scientific, Waltham, USA), using MgKα radiation as the X-ray source. The XPS spectra were calibrated using the binding energy (284.6 eV) of C 1s electrons. The percentage of Ag nanocubes loaded over the α-Al2O3 was determined using inductively coupled plasma optical emission spectroscopy (ICP-OES, Prodigy7, LEEMAN LABS). UV-vis diffusion extinction spectra were measured within the wavelength range from 200 nm to 800 nm using a UV-vis photospectrometer equipped with an optical integration sphere (type: UV-2600, Shimadzu, Tokyo, Japan).
2.3. Photocatalytic experiments
4 mL of the Ag nanocube ethanol solution and 0.5 g commercial α-Al2O3 powder were ultrasonically mixed to form a uniform slurry, which was dispersed over a ϕ 60 mm quartz glass, and dried at 70 °C. The sample was firstly activated through heating at near 200 °C for 1 h in air atmosphere. The catalytic experiments were conducted in a self-designed quartz glass reactor placed upon a heating plate according to our previous studies.44,45 A 500 W xenon lamp, together with optical bandpass filters, was used to illuminate the sample downwards. The light intensities were checked using a Newport Si-based photodetector (843-R-USB). Alcohol–N2 standard gases (concentration: ∼500 ppm) and pure oxygen were flowed through the reaction for ∼30 min under the control of mass flow meters; the flow rate ratio was 5
:
1. Finally, the reactor was connected with a gas chromatograph to form a sealed reaction measurement system. The change of the Ag/Al2O3 surface temperature under light illumination was also checked using IR thermography. IPA, methanol, ethanol, and N-propanol were used for catalytic dehydrogenation. The yields of acetone, formaldehyde, acetaldehyde, and propionaldehyde were used to evaluate the catalytic activities.
2.4. Finite-difference time domain (FDTD) simulation
FDTD simulation was used to obtain the absorption cross-sectional area of the Ag nanocube surface plasmons using the commercial FDTD software (Canadian Lumerical Solutions 7.5). The Ag nanocube was modeled with a side length of 50 nm. The dielectric functions were taken from the Johnson and Christy measurement and corrected for size effect (surface scattering and radiation damping). The refractive index (n) of the surrounding environment was set as the n of air. The whole wavelength scattering field source (TFSF) was used to calculate the absorption cross section with the wavelength range being 300–1000 nm. The grid resolution was set as 1 nm to obtain accurate results.
3. Results and discussion
3.1. Physical characterization
As shown in Fig. 1a and b, the samples obtained after 20 and 50 min reactions are mainly composed of Ag nanocubes, and some Ag nano-triangles and Ag nanospheres are also present. The Ag nanocubes are uniform and the sizes are ∼40 nm and 60 nm for 20 min and 50 min reactions, respectively. As the PVP layer over the Ag nanotubes is not seen, most of it should be removed by washing several times. Fig. 1c shows the TEM image of the small Ag nanocubes, and it can be seen that the Ag nanocubes have spherical corners in addition to edges and faces.46,47 Some nanocubes fuse together at the corners due to the low PVP density and high surface energy. The high-resolution TEM image (Fig. 1d) shows the single crystallinity of the Ag nanocube, and the PVP layer is also not seen in the TEM image. In addition, the percentages of the small and large Ag nanocubes over the α-Al2O3 are determined to be 4.2 wt% and 4.5 wt% by ICP-OES, respectively.
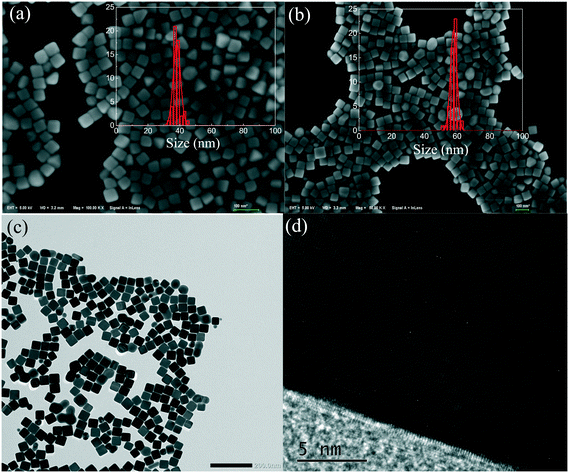 |
| Fig. 1 (a) FE-SEM image of small Ag nanocubes obtained from 20 min of reaction. (b) FE-SEM image of large Ag nanocubes obtained from 50 min of reaction. (c) TEM image of Ag nanocubes obtained from 20 min of reaction. (d) High-resolution TEM image of one Ag nanocube in (c). The size distributions of the Ag nanocubes are shown as insets in (a) and (b). | |
Fig. 2 shows the UV-vis extinction spectra of the Ag nanocubes dispersed in ethanol. The Ag nanocubes show a clear quadrupole plasmon extinction below 400 nm and a strong dipole extinction at ∼450 nm. Charge distribution schematics of dipole and quadrupole oscillations are also shown in Fig. 2; the positive (blue) and negative (red) charges distribute over the corners and the surfaces of Ag nanocubes for the quadrupole and dipole oscillations, respectively. The presence of quadrupole oscillation extinction is therefore a good indication of fine Ag nanocubes. The plasmon extinction shifts to long wavelength as the nanocube size increases from 40 nm to 60 nm, in agreement with other studies.48,49Fig. 2b shows the diffusion extinction spectra of the small Ag nanocubes (40 nm)/α-Al2O3 before heat activation, after heat activation, and after the catalytic reactions. It is seen that the extinction spectrum is changed because of a surface property change caused by the heat activation. The Ag nanocubes should be stable during the catalytic reactions as the extinction spectrum is almost unchanged. As compared to the Ag nanocubes in ethanol, the plasmon absorption spectrum becomes wide and shifts to short wavelength for the Ag nanocubes/α-Al2O3. The transmittance spectra of the 400, 420, and 440 nm optical bandpass filters are shown to see the matching of the lights used for the catalysis with the plasmon absorption band.
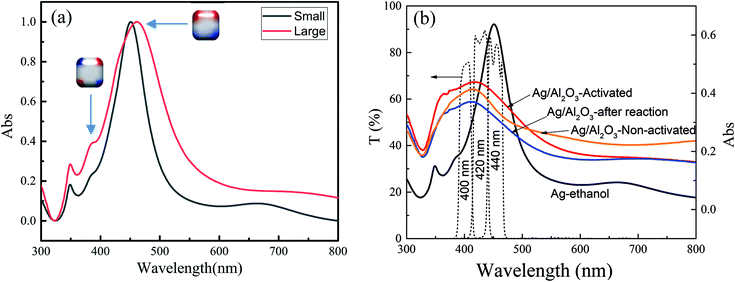 |
| Fig. 2 (a) UV-vis extinction spectra of the Ag nanocubes dispersed in ethanol; (b) UV-vis diffusion extinction spectra of the small Ag nanocubes/α-Al2O3 and the transmittance spectra of the optical bandpass filters. The extinction spectrum of the small Ag nanocube ethanol solution is shown for comparison. | |
Fig. 3a shows the FE-SEM image of the α-Al2O3 loaded with small Ag nanocubes. It is seen that the Ag nanocubes distribute over the α-Al2O3 as bright spots; some nanotubes aggregate together and form polymeric nanocubes. Fig. 3b shows the TEM image of the Ag nanocube/α-Al2O3 after heat activation, and it is seen that the sample is still mainly composed of nanocubes. As shown in Fig. 3c, the nanocube shape is almost kept after the catalytic reaction. The stability of the Ag nanocube morphologies after the heat activation and catalytic reactions agrees with the extinction spectrum (Fig. 2b). In Fig. 4a, the XRD patterns show that the Ag nanocubes are metallic, which are not affected by the heat activation and catalytic reaction. Fig. 4b shows that the Ag 3d XPS peak shifts to high binding energy after the heat activation, which corresponds to metallic Ag.7 Linic et al. also observed that the Ag 3d XPS peak shifts to higher binding energy after heat treatment.7 It is considered that heat activation can destroy the surface capped PVP molecule, which changes the chemical combination between the PVP molecules and the Ag nanocubes, resulting in a change in the Ag chemical states. The binding energy of the XPS Ag 3d peak after heat activation agreed well with the metallic Ag. This indicates that the surfaces of the non-activated Ag nanocubes should be slightly reduced by the PVP ligands because of the lower binding energies.
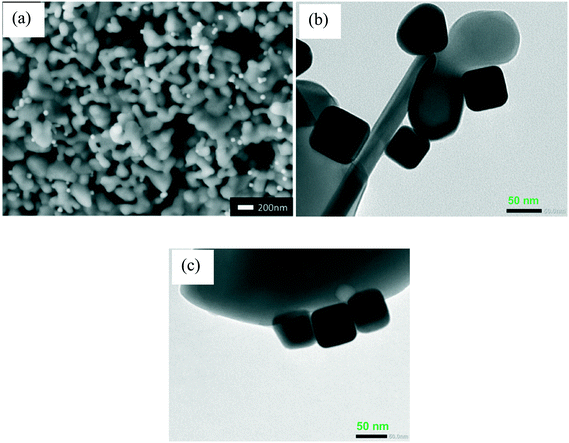 |
| Fig. 3 (a) FE-SEM image of the small Ag nanocubes/α-Al2O3; (b) TEM image of the small Ag nanocubes/α-Al2O3 after heat activation; (c) TEM image of the small Ag nanocubes/α-Al2O3 after the catalytic reactions. | |
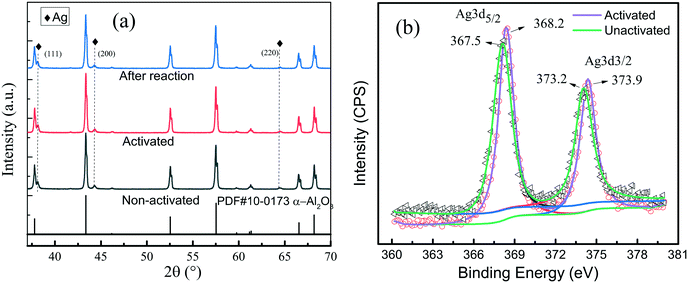 |
| Fig. 4 (a) XRD patterns of small Ag nanocubes/α-Al2O3 before heat activation, after heat activation, and after catalytic reactions; (b) Ag 3d XPS core-level spectra of small Ag nanocubes/α-Al2O3 before and after heat activation. | |
3.2. Catalytic properties
The small Ag nanocubes/α-Al2O3 was used for IPA dehydrogenation. IPA cannot undergo catalytic conversion in the absence of the Ag nanocubes and cannot be oxidized as CO2, but is almost converted to acetone in high selectivity. The Ag nanocubes/α-Al2O3 was firstly activated by heating at 200 °C in air. Fig. 5a shows the acetone yields in the dark and under light illumination for the non-activated and activated samples. The non-activated sample presents very low activity at 110 °C, while the activated sample shows very high activity even at 80 °C, which is further increased by the light illumination. As the XPS result reveals that the metallic Ag state is formed after heat activation, the PVP layer should be destroyed by heating and the metallic surfaces are exposed to reactants. It is shown in Fig. 5b that the catalytic property is stable during the five consecutive reactions conducted at 60 °C under the same light illumination.
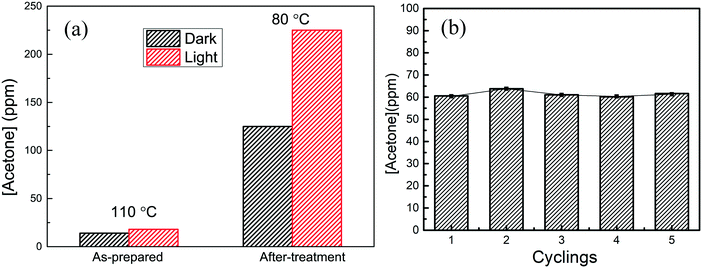 |
| Fig. 5 (a) Yields of the acetone generated from the 84 min isopropanol catalysis over small Ag nanocubes before and after the heat activation in the dark and under 420 nm monochromatic visible light (∼20 mW cm−2) illumination; (b) acetone yields of five consecutive cycles of catalytic reaction at 60 °C under the same light illumination. | |
It has been reported that catalysis over noble materials is dependent on the temperature. For example, Zhu et al. found that the plasmonic photocatalytic oxidation of alcohols over Au/TiO2 increased with the temperature;29 Linic et al. also observed that the photoinduced epoxidation of ethylene over Ag nanocubes increased with the temperature.30 It was also seen that the apparent activation energies (Eapp) of the catalysis under light illumination are lower than that of the dark catalysis. Fig. 6a shows the acetone yields at different temperatures. It is seen that IPA can be converted to acetone at 60 °C in the dark. The light illumination results in a great increase of the activity, almost ∼5 times higher than that of the dark catalysis after taking out the photothermal effect. Light illumination thus provides a facile way for facile selective IPA hydrogenation over Ag nanocubes under gaseous conditions. The temperature dependence means that the IPA dehydrogenation over the Ag nanocubes involves heat activation. The Eapps are estimated to be 114 ± 16 kJ mol−1 and 50 ± 13.3 kJ mol−1 in the dark and under light illumination, respectively, so the light illumination greatly reduces the Eapp; this is also in good agreement with other studies.30 The light enhancement is obtained by subtracting the dark reaction from that under light illumination, as shown in Fig. 6b. It is seen that that light enhancement firstly increases and then decreases as the temperature increases, and the ratios of the acetone yields in the dark and under light illumination decrease; this also accords with other studies.7,50
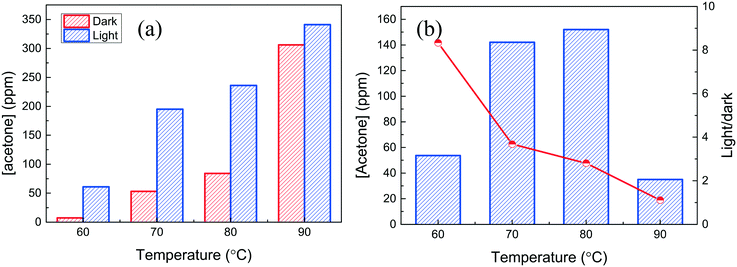 |
| Fig. 6 (a) Temperature dependence of acetone yields in the dark and under 420 nm monochromatic light (∼20 mW cm−2) illumination; (b) temperature dependence of the light-induced acetone yield enhancement and the ratios of the acetone yields in the dark and under light illumination. | |
IPA conversion to acetone was also conducted under the illumination of three monochromatic lights (Fig. 2b). The photon fluxes of the 400 nm and 440 nm lights were normalized to be the same as that of the 420 nm light. Fig. 7a shows that the acetone yields under these light illuminations agree well with the diffusion absorption spectrum of Ag/α-Al2O3. Under 420 nm light illumination, the light-assisted catalytic conversions were also checked at 80 °C by varying light intensities, as shown in Fig. 7b. It has been reported that catalytic activity under light illumination depends on light intensities in super-linear relation at high light intensities due to possible light–heat synergism.7 The light intensities of the present research are low, so the linear relation is also in good agreement with these results; this supports the theory that the light-assisted catalysis is caused by plasmon absorption.
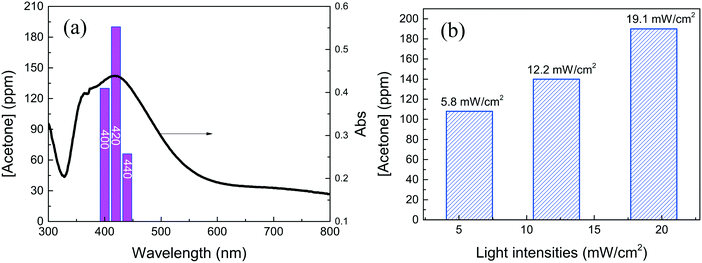 |
| Fig. 7 (a) Acetone yields of the catalysis under 400, 420, and 440 nm monochromatic light illumination at 80 °C; the diffusion absorption spectrum of the Ag nanocubes/α-Al2O3 is also shown. The intensity of 420 nm light is ∼20 mW cm−2, and the photo fluxes of 400 and 440 nm lights are normalized according to the 420 nm light. (b) Dependence of the acetone yields on the intensities of the 420 nm light. | |
Plasmonic non-irradiative decay can transfer energy for photocatalytic effect. It has been shown that the non-irradiative decay decreases as the Ag nanocube size increases.14 We thus compared the catalytic activity between the small and the large Ag nanocubes. As shown in Fig. 8, the higher dark catalytic activity of the small Ag nanocubes should be attributed to the higher surface area. The result clearly shows that the light enhancement of the catalytic activity for the small Ag nanocubes is much higher than that for the large nanocubes; this agrees with the theory that more non-irradiative decay can occur for the small Ag nanocubes. Therefore, the result also indicates that the plasmon absorption contributes to the light-assisted catalysis.
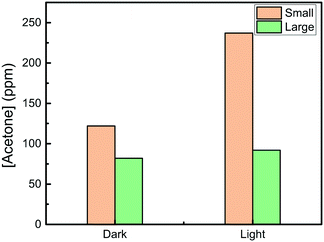 |
| Fig. 8 Acetone yields of the IPA conversion over small and large Ag nanocubes loaded on α-Al2O3 in the dark and under 20 mW cm−2 of 420 nm light illumination. | |
3.3. Contribution of the photothermal effect
Light illumination might cause a photothermal effect that can lead to an overall increase in surface temperature. IR thermography was thus used to check the effect of light illumination on the surface temperature, as shown in Fig. 9. The surface temperatures were increased by 4.3. 2.5, 1.9, and 0.7 °C by the light illumination when the dark temperatures are 60.6, 69.2, 79.8, and 90.0 °C, respectively. According to Fig. 6a, although the light-induced increase of surface temperatures can result in an increase in the dark catalytic activity to some extent, this is still lower than the light enhancement. It is thus thought that the plasmonic non-thermal effect has a dominant contribution.
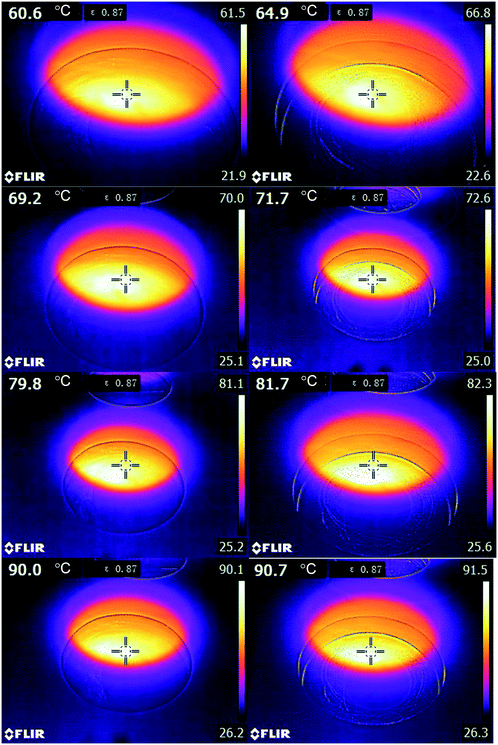 |
| Fig. 9 IR thermal images of small Ag nanocubes/α-Al2O3 in the dark and under 420 nm light illumination (∼20 mW cm−2). | |
In addition, the plasmon heating might lead to a higher local temperature at the Ag nanocubes that cannot be determined by IR thermography; this possibly contributes to the light enhancement in catalysis.51 Plasmon local photothermal heating is usually evaluated from a theoretical prediction. The temperature difference between a Ag nanocube and the environment is calculated according to the following equation.52
| 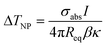 | (1) |
where
σabs is the cross section of plasmon absorption,
I is the incident light intensity,
Req is the radius of equivalent sphere of nanocube,
β is a dimensionless thermal capacity coefficient that relates the particle shape, and
κ is the effective thermal conductivity of α-Al
2O
3 (29 W m
−1 K
−1).
Fig. 10 shows the dependence of the
σabs on the light wavelength. It is seen that the dipole resonance absorption occurs at ∼390 nm. The maximum
σabs is 1.0 × 10
4 nm
2 at 400 nm,
I is set to be 40 mW cm
−2, and
Req was calculated to be 31.02 nm for a nanocube with 50 nm side length.
β is set to 1 for a fine nanocube shape. The Δ
T induced from plasmon heating is estimated to be lower than 3.5 × 10
−7 K. This result is in accordance with other reports that plasmon heating under low-intensity light illumination cannot lead to a temperature difference.
53 The temperatures obtained from the IR thermography should be the real temperatures of the Ag/α-Al
2O
3 under light illumination. It is thus considered that the light enhancement of IPA catalysis arises from the plasmonic non-thermal effect.
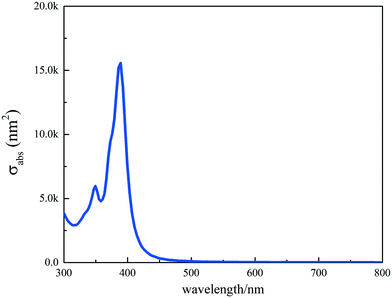 |
| Fig. 10 Absorption cross sections obtained from the FDTD simulations for a Ag nanocube with 40 nm side length. | |
3.4. Mechanism analysis
The above results confirm that the light-induced IPA dehydrogenation should be driven by hot electron transfer. In the presence of molecular O2, the dehydrogenation mainly occurs through α-hydrogen abstraction by oxygen. IPA dehydrogenation thus involves both α-hydrogen and O2 activation. As the α-hydrogen activity is dependent on the chemical coordination of the α-carbons in alcohols, so the dehydrogenation should relate to the alcohol space configuration. The catalytic dehydrogenation reactions of methanol, ethanol, N-propanol, and IPA were compared at 80 °C, as shown in Fig. 11. It is seen that the Ag nanocubes are inactive for methanol dehydrogenation, and ethanol, N-propanol, and IPA can be converted to acetaldehyde, propionaldehyde, and acetone in near 100% selectivity, respectively. The conversion yields increase from ethanol to N-propanol and then to isopropanol independent of light illumination. It is also seen that the light enhancement is the highest for the IPA catalysis. Because of the two methyl groups connecting with the α-carbon, the α-hydrogen in IPA is the most active, which results in the highest activity for the catalysis over the Ag nanocubes.
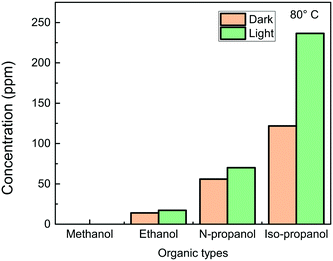 |
| Fig. 11 Conversion of methanol, ethanol, N-propanol, and IPA in the dark and under 420 nm monochromatic light (∼20 mW cm−2) illumination at 80 °C after 84 min reactions. | |
Several pathways for O2 activation were proposed. Plasmons firstly undergo Landau decay to form hot electrons, which can then transfer to O2. The resulting O2− species have a higher potential energy landscape and longer equilibrium O–O distance. When the O2− releases the electron back to the Ag nanocube, extra energy can be deposited in the excited vibration state of O2. Linic et al. considered that this excited vibration state of O2 is more easily activated as atomic oxygens that can take the α-hydrogens.7 This mechanism can well explain the dependence of the above catalysis on temperature, light intensity, and light wavelength.
Because O2 can accept an electron under plasmon excitation to form strongly adsorbed O2− anions, it was also proposed that the anions are transformed to surface Ag oxides or hydroxides that can oxidize surface species. For example, it was seen that p-aminothiophenol can be selectively oxidized as p,p′-dimercaptoazobenzene (DMAB) through Ag oxides/hydroxides.54 For Au/TiO2 materials, it was also proposed by Tsukamoto et al. that the hot electron transfer can lead to Ti–O–O− species over Au/TiO2 interfaces, which can then extract the α-hydrogens of 1-phenylethanol to form acetophenone.55 A similar –O–O− structure might form over Ag/α-Al2O3 interfaces when an O2 captures a hot electron. We thought that that the above proposed O2 activation mechanisms can all possibly contribute to plasmon-assisted enhancement of the catalytic activity. The detailed microscopic pathway of O2 activation for IPA dehydrogenation is not the purpose of this research and will be investigated in our future studies. The O2 activation should arise from the hot electron transfer from the Ag nanocubes, although the detailed activation mechanism might be different.
4. Conclusion
It was shown that IPA underwent fast catalytic dehydrogenation at low temperatures over Ag nanocubes under gaseous conditions in high selectivity. Light illumination could lead to a great increase in the catalytic activity. The results indicated that the light-induced increase of the catalytic effect should mainly arise from the hot electron transfer, and the photothermal effect has a low contribution. The high plasmonic IPA dehydrogenation over the Ag nanocubes depends on the high activity of the α-hydrogens in IPA molecules as compared to methanol, ethanol, and N-propanol. The hot electron transfer to molecular O2 is thought to be the initial step for O2 activation, which might cause IPA dehydrogenation via different pathways.
Conflicts of interest
There are no conflicts to declare.
Acknowledgements
B. L. thanks the National Natural Science Foundation of China (No. 51772230), the National Key Research and Development of China (No. 2017YFE0192600), and the 111 Project (No. B18038). B. Liu also thanks the Open Foundation of Key Laboratory for UV-Emitting Materials and Technology of the Ministry of Education, Northeast Normal University (Grant No. 135130013).
References
- M. Wang, M. Ye, J. Iocozzia, C. Lin and Z. Lin, Plasmon-Mediated Solar Energy Conversion via Photocatalysis in Noble Metal/Semiconductor Composites, Adv. Sci., 2016, 3, 1600024 CrossRef PubMed
.
- M. Valenti, M. P. Jonsson, G. Biskos, A. Schmidt-Otta and W. A. Smith, Plasmonic Nanoparticle-Semiconductor Composites for Efficient Solar Water Splitting, J. Mater. Chem. A, 2016, 4, 17891–17912 RSC
.
- Y. Zhang, S. He, W. Guo, Y. Hu, J. Huang, J. R. Mulcahy and W. D. Wei, Surface-Plasmon-Driven Hot Electron Photochemistry, Chem. Rev., 2018, 118, 2927–2954 CrossRef CAS
.
- C. Zhan, X. J. Chen, Y. F. Huang, D. Y. Wu and Z. Q. Tian, Plasmon-Mediated Chemical Reactions on Nanostructures Unveiled by Surface-Enhanced Raman Spectroscopy, Acc. Chem. Res., 2019, 52, 2784–2792 CrossRef CAS PubMed
.
- K. L. Kelly, E. Coronado, L. L. Zhao and G. C. Schatz, The Optical Properties of Metal Nanoparticles: The Influence of Size, Shape, and Dielectric Environment, J. Phys. Chem. B, 2003, 107, 668–677 CrossRef CAS
.
- J. R. M. Saavedra, A. Asenjo-Garcia and J. G. de Abajo, Hot-Electron Dynamics and Thermalization in Small Metallic Nanoparticles, ACS Photonics, 2016, 3, 1637–1646 CrossRef CAS
.
- O. Christopher, H. Xin, A. Marimuthu and S. Linic, Singular Characteristics and Unique Chemical Bond Activation Mechanisms of Photocatalytic Reactions on Plasmonic Nanostructures, Nat. Mater., 2012, 11, 1044–1050 CrossRef PubMed
.
- K. Watanabe, D. Menzel, N. Nilius and H. J. Freund, Photochemistry on Metal Nanoparticles, Chem. Rev., 2016, 106, 4301–4320 CrossRef
.
- J. Bosbach, C. Hendrich, F. Stietz, T. Vartanyan and F. Träger, Ultrafast Dephasing of Surface Plasmon Excitation in Silver Nanoparticles: Influence of Particle Size, Shape, and Chemical Surrounding, Phys. Rev. Lett., 2002, 89, 257404 CrossRef CAS
.
- S. Y. Lee, P. V. Tsalu, G. W. Kim, M. J. Seo, J. W. Hong and J. W. Ha, Tuning Chemical Interface Damping: Interfacial Electronic Effects of Adsorbate Molecules and Sharp Tips of Single Gold Bipyramids, Nano Lett., 2019, 19, 2568–2574 CrossRef CAS PubMed
.
- B. Foerster, V. A. Spata, E. A. Carter, C. Sönnichsen and S. Link, Plasmon Damping Depends on the Chemical Nature of the Nanoparticle Interface, Sci. Adv., 2019, 5, eaav0704 CrossRef CAS
.
- S. Mukherjee, L. Zhou, A. M. Goodman, N. Large, C. Ayala-Orozco, Y. Zhang, P. Nordlander and N. J. Halas, Hot-Electron-Induced Dissociation of H2 on Gold Nanoparticles Supported on SiO2, J. Am. Chem. Soc., 2014, 136, 64–67 CrossRef CAS PubMed
.
- S. Mukherjee, F. Libisch, N. Large, O. Neumann, L. V. Brown, J. Cheng, J. B. Lassiter, E. A. Carter, P. Nordlander and N. J. Halas, Hot Electrons do the Impossible: Plasmon-Induced Dissociation of H2 on Au, Nano Lett., 2013, 13, 240–247 CrossRef CAS PubMed
.
- S. Chavez, U. Aslam and S. Linic, Design Principles for Directing Energy and Energetic Charge Flow in Multicomponent Plasmonic Nanostructures, ACS Energy Lett., 2018, 3, 1590–1596 CrossRef CAS
.
- M. J. Landry, A. Gellé, B. Y. Meng, C. J. Barrett and A. Moores, Surface-Plasmon-Mediated Hydrogenation of Carbonyls Catalyzed by Silver Nanocubes under Visible Light, ACS Catal., 2017, 7, 6128–6133 CrossRef CAS
.
- Y. Shiraishi, J. Imai, N. Yasumoto, H. Sakamoto, S. Tanaka, S. Ichikawa and T. Hirai, Doping of Nb5+ Species at the Au−TiO2 Interface for Plasmonic Photocatalysis Enhancement, Langmuir, 2019, 35, 5455–5462 CrossRef CAS
.
- H. Sakamoto, T. Ohara, N. Yasumoto, Y. Shiraishi, S. Ichikawa, S. Tanaka and T. Hirai, Hot-Electron-Induced Highly Efficient O2 activation by Pt Nanoparticles Supported on Ta2O5 Driven by Visible light, J. Am. Chem. Soc., 2015, 137, 9324–9332 CrossRef CAS
.
- Y. Shiraishi, N. Yasumoto, J. Imai, H. Sakamoto, S. Tanaka, S. Ichikawa, B. Ohtani and T. Hirai, Quantum Tunneling Injection of Hot Electrons in Au/TiO2 Plasmonic Photocatalysts, Nanoscale, 2017, 9, 8349–8361 RSC
.
- Y. Nishijima, K. Ueno, Y. Kotake, K. Murakoshi, H. Inoue and H. Misawa, Near-Infrared Plasmon-Assisted Water Oxidation, J. Phys. Chem. Lett., 2012, 3, 1248–1252 CrossRef CAS PubMed
.
- B. Wu, D. Liu, S. Mubeen, T. T. Chuong, M. Moskovits and G. D. Stucky, Anisotropic Growth of TiO2 onto Gold Nanorods for Plasmon-Enhanced Hydrogen Production from Water Reduction, J. Am. Chem. Soc., 2016, 138, 1114–1117 CrossRef CAS
.
- Y. Nishijima, K. Ueno, Y. Yokota, K. Murakoshi and H. Misawa, Plasmon-Assisted Photocurrent Generation from Visible to Near-Infrared Wavelength Using an Au-Nanorods/TiO2 Electrode, J. Phys. Chem. Lett., 2010, 1, 2031–2036 CrossRef CAS
.
- J. Lee, S. Mubeen, X. Ji, G. D. Stucky and M. Moskovits, Plasmonic Photoanodes for Solar Water Splitting with Visible Light, Nano Lett., 2012, 12, 5014–5019 CrossRef CAS PubMed
.
- X. Zhang, X. Li, M. E. Reish, D. Zhang, N. Q. Su, Y. Gutiérrez, F. Moreno, W. Yang, H. O. Everitt and J. Liu, Plasmon-Enhanced Catalysis: Distinguishing Thermal and Nonthermal effects, Nano Lett., 2018, 18, 1714–1723 CrossRef CAS PubMed
.
- X. Li, H. O. Everitt and J. Liu, Confirming Nonthermal Plasmonic Effects Enhance CO2 Methanation on Rh/TiO2 Catalysts, Nano Res., 2019, 12, 1906–1911 CrossRef CAS
.
- X. Li, H. O. Everitt and J. Liu, Synergy between Thermal and Nonthermal Effects in Plasmonic Photocatalysis, Nano Res., 2020, 13, 1268–1280 CrossRef
.
- L. Zhou, D. F. Swearer, C. Zhang, H. Robatjazi, H. Zhao, L. Henderson, L. Dong, P. Christopher, E. A. Carter, P. Nordlander and N. J. Halas, Quantifying Hot Carrier and Thermal Contributions in Plasmonic Photocatalysis, Science, 2018, 362, 69–72 CrossRef CAS
.
- E. Kowalska, R. Abea and B. Ohtania, Visible light-induced photocatalytic reaction of gold-modified titanium (IV) oxide particles: action spectrum analysis, Chem. Commun., 2009, 241–243 RSC
.
- E. Kowalska, O. O. P. Mahaney, R. Abea and B. Ohtani, Visible-Light-Induced Photocatalysis through Surface Plasmon Excitation of Gold on Titania Surfaces, Phys. Chem. Chem. Phys., 2010, 12, 2344–2355 RSC
.
- X. S. Li, X. Y. Ma, J. L. Liu, Z. G. Sun, B. Zhu and A. M. Zhu, Plasma-Promoted Au/TiO2 Nanocatalysts for Photocatalytic Formaldehyde Oxidation under Visible-Light Irradiation, Catal. Today, 2019, 337, 132–138 CrossRef CAS
.
- P. Chiristopher, H. Xin and S. Linic, Visible-Light-Enhanced Catalytic Oxidation Reactions on Plasmonic Silver Nanostructures, Nat. Chem., 2011, 3, 467–472 CrossRef
.
- U. Aslam, V. G. Rao, S. Chavez and S. Linic, Catalytic Conversion of Solar to Chemical Energy on Plasmonic Metal Nanostructures, Nat. Catal., 2018, 1, 656–665 CrossRef
.
- S. Linic, P. Christopher, H. Xin and A. Marimuthu, Catalytic and Photocatalytic Transformations on Metal Nanoparticles with Targeted Geometric and Plasmonic Properties, Acc. Chem. Res., 2013, 46, 1890–1899 CrossRef CAS PubMed
.
- N. Hooshmand, J. A. Bordley and M. A. El-Sayed, The Sensitivity of the Distance Dependent Plasmonic Coupling between Two Nanocubes to their Orientation: Edge-to-Edge versus Face-to-Face, J. Phys. Chem. C, 2016, 120, 4564–4570 CrossRef CAS
.
- P. Christopher, D. B. Ingram and S. Linic, Enhancing Photochemical Activity of Semiconductor Nanoparticles with Optically Active Ag Nanostructures: Photochemistry Mediated by Ag Surface Plasmons, J. Phys. Chem. C, 2010, 114, 9173–9177 CrossRef CAS
.
- P. H. Rana and P. A. Parik, Bioethanol Selective Oxidation to Acetaldehyde over Ag–CeO2: Role of Metal–Support Interactions, New J. Chem., 2017, 41, 2636–2641 RSC
.
- J. Zhang, K. Shi, Z. An, Y. Zhu, X. Shu, H. Song, X. Xiang and J. He, Acid−Base Promoted Dehydrogenation Coupling of Ethanol on Supported Ag Particles, Ind. Eng. Chem. Res., 2020, 59, 3342–3350 CrossRef CAS
.
- W. Liu, J. Sun, X. Zhang and M. Wei, Supported Ag Catalysts on Mg−Al Oxides toward Oxidant-Free Dehydrogenation Reaction of Benzyl Alcohol, Ind. Eng. Chem. Res., 2018, 57, 15606–15612 CrossRef CAS
.
- V. V. Torbina, A. A. Vodyankin, S. Ten, G. V. Mamontov, M. A. Salaev, V. I. Sobolev and O. V. Vodyankina, Ag-Based Catalysts in Heterogeneous Selective Oxidation of Alcohols: A Review, Catalysts, 2018, 8, 447 CrossRef
.
- A. Mourhly, A. E. Hamidi, M. Halim and S. Arsalane, Selective Gas Conversion of Isopropyl alcohol over silver nanoparticles (Ag-NPs) supported on new mesoporous silica precipitated from natural resources, Res. Chem. Intermed., 2019, 45, 5091–5109 CrossRef CAS
.
- W. Feng, G. Wu, L. Li and N. Guan, Solvent-free Selective Photocatalytic Oxidation of Benzyl Alcohol over Modified TiO2, Green Chem., 2011, 13, 3265–3272 RSC
.
- P. Zhang, P. Wu, S. Bao, Z. Wang, B. Tian and J. Zhang, Synthesis of Sandwich-structured AgBr@Ag@TiO2 Somposite Photocatalyst and Study of Its Photocatalytic Performance for the Oxidation of Benzyl Alcohols to Benzaldehydes, Chem. Eng. J., 2016, 306, 1151–1161 CrossRef CAS
.
- S. Li, J. Cai, X. Wu and F. Zheng, Sandwich-like TiO2@ZnO-based noble metal (Ag, Au, Pt, or Pd) for betterphoto-oxidation performance: Synergistic effect between noble metal and metal oxide phases, Appl. Surf. Sci., 2018, 443, 603–612 CrossRef CAS
.
- Q. Zhang, W. Li, L. P. Wen, J. Chen and Y. Xia, Facile Synthesis of Ag Nanocubes of 30 to 70 nm in Edge Length with CF3COOAg as a Precursor, Chem. – Eur. J., 2010, 16, 10234–10239 CrossRef CAS PubMed
.
- B. Liu, H. Wu and I. P. Parkin, Gaseous Photocatalytic Oxidation of Formic Acid over TiO2: A Comparison between the Charge Carrier Transfer and Light-Assisted Mars–van Krevelen Pathways, J. Phys. Chem. C, 2019, 123, 22261–22272 CrossRef CAS
.
- B. Liu, H. Wu, X. Zhang, I. P. Parkin and X. Zhao, New Insight into the Role of Electron Transfer to O2 in Photocatalytic Oxidations of Acetone over TiO2 and the Effect of Au Cocatalyst, J. Phys. Chem. C, 2019, 123, 30958–30971 CrossRef CAS
.
- S. W. Hsu and A. R. Tao, Halide-Directed Synthesis of Square Prismatic Ag Nanocrystals by the Polyol Method, Chem. Mater., 2018, 30, 4617–4623 CrossRef CAS
.
- A. Ruditskiy and Y. Xia, Toward the Synthesis of Sub-15 nm Ag Nanocubes with Sharp Corners and Edges: The Roles of Heterogeneous Nucleation and Surface Capping, J. Am. Chem. Soc., 2016, 138, 3161–3167 CrossRef CAS
.
- K. L. Kelly, E. Coronado, L. L. Zhao and G. C. Schatz, The Optical Properties of Metal Nanoparticles: The Influence of Size, Shape, and Dielectric Environment, J. Phys. Chem. B, 2003, 107, 668–677 CrossRef CAS
.
- Q. Zhang, W. Li, C. Moran, J. Zeng, J. Chen, L.-P. Wen and Y. Xia, Seed-Mediated Synthesis of Ag Nanocubes with Controllable Edge Lengths in the Range of 30-200 nm and Comparison of Their Optical Properties, J. Am. Chem. Soc., 2010, 132, 11372–11378 CrossRef CAS
.
- U. Aslam, S. Chavez and S. Linic, Controlling Energy Flow in Multimetallic Nanostructures for Plasmonic Catalysis, Nat. Nanotechnol., 2017, 12, 1000–1007 CrossRef CAS
.
- C. Zhan, B. W. Liu, Y. F. Huang, S. Hu, B. Ren, M. Moskovits and Z. Q. Tian, Disentangling Charge Carrier from Photothermal Effects in Plasmonic Metal Nanostructures, Nat. Commun., 2019, 10, 2671 CrossRef
.
- G. Baffou, R. Quidant and G. D. A. F. Javier, Nanoscale control of optical heating in complex plasmonic systems, ACS Nano, 2010, 4, 709–716 CrossRef CAS PubMed
.
- A. O. Govorov, W. Zhang, T. Skeini, H. Richardson, J. Lee and N. A. Kotov, Gold Nanoparticle Ensembles as Heaters and Actuators: Melting and Collective Plasmon Resonances, Nanoscale Res. Lett., 2006, 1, 84–90 CrossRef
.
- Y. F. Huang, M. Zhang, L. B. Zhao, J. M. Feng, D.-Y. Wu, B. Ren and Z.-Q. Tian, Activation of Oxygen on Gold and Silver Nanoparticles Assisted by Surface Plasmon Resonances, Angew. Chem., Int. Ed., 2014, 53, 2353–2357 CrossRef CAS PubMed
.
- D. Tsukamoto, Y. Shiraishi, Y. Sugano, S. Ichikawa, S. Tanaka and T. Hirai, Gold Nanoparticles Located at the Interface of Anatase/Rutile TiO2 Particles as Active Plasmonic Photocatalysts for Aerobic Oxidation, J. Am. Chem. Soc., 2012, 134, 6309–6315 CrossRef CAS PubMed
.
|
This journal is © The Royal Society of Chemistry 2022 |
Click here to see how this site uses Cookies. View our privacy policy here.