DOI:
10.1039/D1CE00700A
(Paper)
CrystEngComm, 2022,
24, 83-94
Regulation of growth temperature on structure, magnetism of epitaxial FeCo2O4 films
Received
27th May 2021
, Accepted 8th November 2021
First published on 8th November 2021
Abstract
Epitaxial FeCo2O4 films were grown by pulsed laser deposition on Al2O3 (0001) substrates at different growth temperatures in this work. The growth temperature directly affects the occupation of cations and the lattice constant, resulting in different magnetic phases and conductivity. The optimal growth temperature range was 450–550 °C. Double bandgaps were observed in the UV absorption spectra. Electrical measurements showed that the film grown at 450 °C had the lowest activation energy, and the structure of this film was more ordered. The magnetic analysis demonstrated that, in addition to the ferrimagnetic phase formed by the antiparallel arrangement of the tetrahedral and octahedral magnetic moments, there were two kinds of antiferromagnetic phases. One kind of antiferromagnetism was from the antiphase boundaries, and the other was localized antiferromagnetism at the octahedra formed by the Co2+ ions. The three kinds of magnetic phases showed different characteristics with changes in the growth temperature and measurement temperature.
1. Introduction
Spinel ferrites have been extensively investigated in recent decades. In particular, cobalt-substituted magnetite (Fe3−xCoxO4) is very attractive due to the typical anisotropy of the Co ions.1–3 It is an important ferromagnetic material with excellent physical properties, such as high Curie temperature,4 high permeability,5 high resistivity6 and good thermal stability,7 and can be widely used in magnetic storage, semiconductor devices, electrode materials and other fields.8–10 At present, investigations on FeCo2O4 mainly focus on its powder form,7,9 while there are a few reports on FeCo2O4 films.11 The calcination temperature has a great influence on the distribution of the tetrahedral and octahedral cations in the spinel structure.12–14 Some reports show that partial cationic inversion in spinel can induce magnetic hardening.15 Our previous studies have demonstrated that pure phase FeCo2O4 powders can only be formed in the calcination temperature range of 950–1050 °C,13 which affects cation occupation and the magnetic properties. Generally, non-stoichiometric phases are ubiquitous, extensively found in the transition metal oxides, and result in the formation of intrinsic or native defects.16 Compared with the polycrystalline structure, high-quality epitaxial films are more practical in reflecting the intrinsic properties of the material. During the preparation of films, the growth temperature (TG) is an important parameter as it provides activation energy to the atoms and directly affects atomic mobility.8 Our previous research has shown that the TG of epitaxial NiCo2O4 films can change the valence and occupation of the cations. At higher TG, octahedral Ni ions can replace tetrahedral Co ions more easily and change the relative Ni3+/Co3+ concentration.17 Combined with oxygen pressure, TG can tune the metallic behavior and magnetic phases of the NiCo2O4 film.18 In CoFe2O4 films, it was found that with the increase in growth temperature, the cations migrated between the tetrahedral and octahedral sites, and the spinel structure showed a tendency to change from inverse to normal spinel.12 The TG is also known to have significant effects on the crystallinity,19,20 lattice parameters and surface morphology of films.21,22 Therefore, it is necessary to explore the effect of growth temperature on the magnetic and electrical properties of epitaxial FeCo2O4 films.
In this work, FeCo2O4 films were grown by pulsed laser deposition (PLD) on Al2O3 (0001) substrates at different growth temperatures. The effect of TG on the growth mode was investigated. The magnetic properties of the films were analyzed, and it was found that with an increase in TG, the magnetic domains became more and more pronounced. In addition to ferrimagnetism presented by the antiparallel arrangement of the tetrahedral and octahedral magnetic moments, two kinds of antiferromagnetism are thought to coexist in the films. One is due to the presence of the antiphase boundary, and the other local lattice antiferromagnetism in the octahedral sites due to the Co2+ ions, which increase with TG. The electrical transport properties exhibited typical semiconductor properties. The activation energy of the film grown at 450 °C was the lowest, and the structure of the film could be considered as a standard spinel structure.
2. Experimental details
2.1. Film preparation
The Al2O3 (0001) substrate was first ultrasonically cleaned in acetone and ethanol, then with deionized water and finally dried with nitrogen. The substrate was placed in a vacuum chamber. The base pressure was 1.5 × 10−5 mTorr. Prior to film deposition, the substrate was heat-treated at 700 °C for 2 h under the oxygen pressure of 75 mTorr to remove the damages on the crystal surface caused by machine polishing. Then, the epitaxial FeCo2O4 films were grown on Al2O3 (0001) single-crystal substrates at different temperatures between 250–650 °C by using the pulsed laser deposition technique with a KrF excimer laser (λ = 248 nm) of energy 3.94 J cm−2. During deposition, the oxygen pressure was maintained at 75 mTorr. All the films were deposited at a frequency of 8 Hz for 20
000 shots.
2.2. Structural characterization and property measurement
The structures of the films were investigated by X-ray diffraction (X'pert PRO, XRD, Cu-Kα radiation, λ = 1.54 Å) and high-resolution transmission electron microscopy (HR-TEM, FEI Tecnai F20). The cross-sectional TEM samples were prepared by mechanical thinning and ion milling with Ar+ ions at 3.2–4.8 keV for a few hours. The thickness of the film was determined by X-ray reflection (XRR). The surface morphologies of the FeCo2O4 films were scanned using an atomic force microscope (AFM, Nanoscope IV). The magnetic domains of the films were characterized by magnetic force microscopy (MFM, Nanoscope IV). Raman measurements were conducted at room temperature using a Renishaw's InVia Raman microscope with an excitation wavelength of 532 nm. The bond structure and chemical state of the atoms in the films were characterized by Fourier transform infrared spectroscopy (FTIR, Nicolet 380). The bandgap of the films was measured by an ultraviolet-visible spectrophotometer (UV-3600), and the optical bandgap was analyzed from the measured reflection spectrum. The magnetic properties were measured by a superconducting quantum interference device (Quantum Design, SQUID-VSM) magnetometer. The SQUID measurements recorded the hysteresis loop (M–H) of the films in the temperature range of 2–400 K. The zero-field cooled (ZFC) and field cooled (FC) curves were measured at a magnetic field of 500 Oe. The electronic conductivity of the film was measured by the standard four-probe method.
3. Results and discussion
3.1. Structure and morphology
Fig. 1(a) shows the crystal structure of FeCo2O4, which is cubic in the space group Fd
m, while Al2O3 is hexagonal in the space group R
C (Fig. 1(b)). FeCo2O4 can be epitaxially grown on Al2O3 (0001) in principle, and FeCo2O4 (111) films are grown in a triangular lattice. Fig. 1(c) shows the atomic model of the FeCo2O4 (111)/Al2O3 (0001) epitaxial relationship. The solid hexagonal shape with blue atoms represents the FeCo2O4 lattice, and the gray atoms in the dashed hexagon show the Al2O3 lattice. According to the lattice mismatch formula17 | ε = (dfilm − dsub)/dsub | (1) |
where dfilm and dsub represent the atomic spacings of the FeCo2O4 film and Al2O3 (0001) substrate, respectively. The lattice mismatch between FeCo2O4 (111) and Al2O3 (0001) was 21.7%, and the films suffered compressive stress.
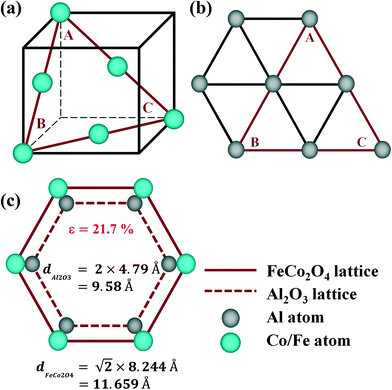 |
| Fig. 1 Schematic diagrams of the (a) FeCo2O4 crystal structure and (b) Al2O3 crystal structure; (c) the FeCo2O4 (111)/Al2O3 (0001) epitaxial relationship atomic model. | |
Fig. 2(a) shows the XRD patterns of the FeCo2O4 epitaxial films grown at different TG. It can be observed that, with the increase in TG, the crystallization quality of the film improved. When the growth temperature was in the range of 450–550 °C, the epitaxial films showed only one orientation with good crystal quality. For clarity, the (222) diffraction peaks of the FeCo2O4 film are enlarged in Fig. 2(b), and it was found that, with increasing TG, the diffraction peak first moved to a lower angle and then to a higher angle, indicating that the out-of-plane lattice constant increased first and then decreased. On increasing the growth temperature further to 650 °C, the diffraction peak returned back to a lower angle, and the out-of-plane lattice constant increased again. Fig. 2(d) gives the thickness of films grown at different TG, as measured by XRR, and the inset is the film thickness curve of the FeCo2O4 film grown at 350 °C. Differences in TG change the atomic mobility and the adhesion of atoms on the substrate. Therefore, the epitaxial films still showed different thicknesses due to the different growth temperatures, even if the same oxygen pressure, shots and power were maintained in the process of film growth.
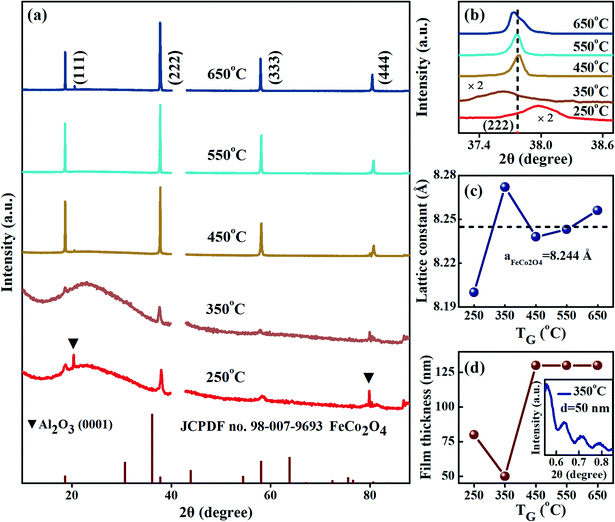 |
| Fig. 2 (a) XRD patterns of the FeCo2O4 films grown at different TG; (b) the enlarged view of the (222) peaks; (c) out-of-plane lattice constants of the FeCo2O4 films grown at different TG; (d) change in film thickness with TG, as measured by XRR. The inset shows the XRR curve of the FeCo2O4 film grown at 350 °C. | |
The out-of-plane lattice constants calculated by the Bragg diffraction equation are shown in Fig. 2(c). When the TG was low (250 °C), a cation deficient spinel with cation vacancies and a small lattice constant was obtained.23 The out-of-plane lattice constant of the FeCo2O4 film grown at 350 °C was larger than that of bulk FeCo2O4 (8.244 Å). We noted that the thickness of the film grown at 350 °C had a large lattice constant and was the thinnest (Fig. 2(d)), making the film more susceptible to compressive stress from the substrate. When the TG was increased to 450–650 °C, the film (∼130 nm) was thick enough, and the stress was released. More octahedral Co2+ had formed in the films, and the lattice constant was mainly affected by cation occupation, which was confirmed by the FTIR results presented below. Considering the fact that the radius of Co2+ is greater than those of Fe3+ and Co3+, and the lattice constants of the FeCo2O4 films grown at 450–650 °C increased with the increase in Co2+ content. In our previous studies on FeCo2O4 powders, it has also been found that different calcination temperatures had a great effect on cation occupancy.13 When the temperature was 250 °C, the film presented low crystal quality, and the full width at half-maximum (FWHM) was wide. For the film grown at 350 °C, the compressive stress was larger, and the FWHM further increased. With increasing growth temperature, the intensity of the diffraction peaks gradually enhanced, the peak width decreased, and the crystallization quality of the film improved. For the film grown at 650 °C, the (222) peak became asymmetric, which indicated that the FeCo2O4 film was partially decomposed at 650 °C. A similar report has been made on the NiCo2O4 epitaxial film.24 From the above analysis, it is evident that low growth temperatures mainly affect the migration of atoms, while high growth temperatures play an important role in regulating the occupation of cations.
In order to observe the surface morphology and domain structure of the films, AFM (left) and MFM (right) measurements were performed. Fig. 3(a–e) shows the morphology and domain images of the FeCo2O4 epitaxial films grown at different TG. We could find that the surface roughness of the films grown at lower and very high TG was elevated. At lower growth temperatures, the adsorbed atoms on the substrates would not have enough energy to cross the energy barrier to reach an equilibrium position.18 Therefore, there was great agglomeration in the film grown at 250 °C. With an increase in TG, the mobility of the atoms on the surface of the films would increase gradually. As a result, the agglomeration phenomenon would decrease gradually, with the atomic deposition mode gradually changing to layered growth.19 Therefore, agglomeration in the film grown at 350 °C was decreased, and the film had become thinner. At 450 °C and 550 °C, the growth temperature was high enough for the surface particles of the films to be uniform, rendering the surface smooth. When the TG was higher than 650 °C, the agglomeration of the particles appeared again due to large grain sizes. Considering the large mismatch between the substrate and the FeCo2O4 lattice, some defects may have formed during the growth of the film, such as lattice defects and stacking faults in the lattice. These defects can lead to antiphase boundaries (APBs). As the TG increased, the APBs in the film decreased. The films grown at 450–550 °C demonstrated good epitaxial quality. Magnetic domains appeared in the films when the TG was above 450 °C. With a further increase in TG, the magnetic domains gradually became obvious, indicating an enhancement in the magnetic moment with increasing TG. To further verify the existence of different domains in the film, we performed micromagnetic simulations based on the idea of finite segmentation.25 The models were set to have a length of 500 nm and a thickness of 130 nm, and the grids were divided into 5 nm × 5 nm × 1 nm. The simulation parameters, namely saturated magnetization, exchange integral constant, and magnetocrystalline anisotropy, were MS = 0.5 × 106 A m−1, A = 1.2 × 10−11 J m−1, and K = 0.7 × 106 J m−3, respectively.26 The magnetic moment distribution of FeCo2O4 was obtained (Fig. 3(f)), and different ferromagnetic regions were observed in the films, and spin inversion could be found at the interface.
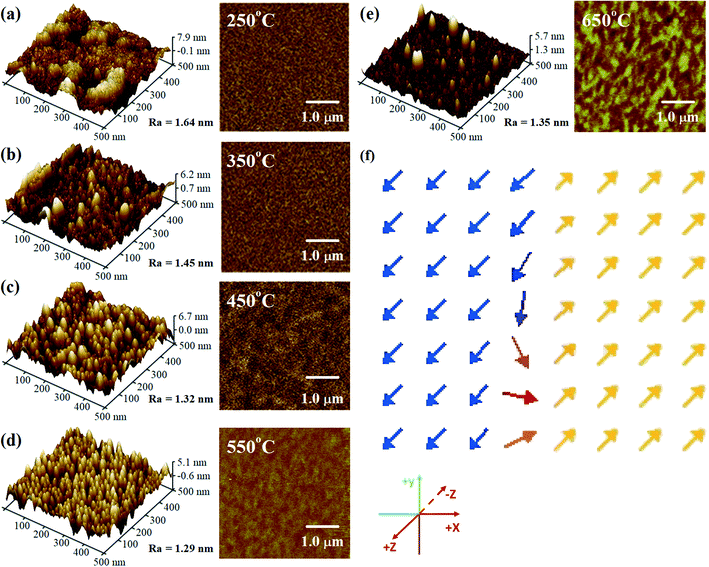 |
| Fig. 3 (a–e) AFM (left) and MFM (right) images and (f) magnetic moment distribution of the FeCo2O4 films obtained by micromagnetic simulation. | |
To determine the crystal structure of the FeCo2O4 thin films, the HR-TEM observations of the FeCo2O4 film on the Al2O3 substrate (TG = 550 °C) were recorded. Fig. 4(a) shows a cross-sectional HR-TEM image of the FeCo2O4 film on Al2O3 along the (111) direction. It was found that there were different crystal plane spacings. The standard FeCo2O4 (111) crystal plane spacing was 4.759 Å. More octahedral Co2+ ions and the partial decomposition of the sample would lead to an increase in crystal plane spacing. Furthermore, it was found from the enlarged image (the inset of Fig. 4(a)) that APBs were formed by the stacking faults of the lattice. Therefore, we believed that there might be different regions in the film. The selected area electron diffraction (SAED) patterns of the film and partial substrate showed spots arranged in hexagons (Fig. 4(b)). The crystal plane corresponding to the diffraction spots was calibrated according to the distance and angle between the diffraction spots. The lattice mismatch between FeCo2O4 (111) and Al2O3 (0001) was observed in the enlarged view. However, the mismatch was not as large as that obtained from the theoretical calculation. It is thought that the observation direction is not exactly perpendicular to the diffraction plane.
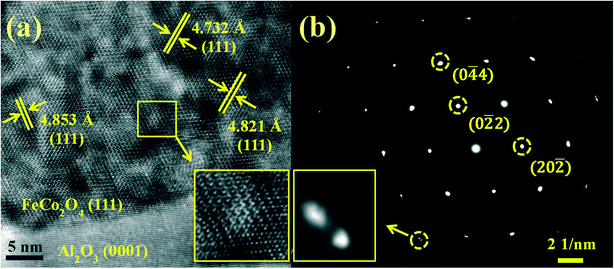 |
| Fig. 4 (a) Cross-sectional HR-TEM image of FeCo2O4 (111)/Al2O3 (0001); (b) selected area electron diffraction patterns of FeCo2O4 (111)/Al2O3 (0001) along the (111) zone axis. | |
Fig. 5(a) shows the Raman spectra of the FeCo2O4 films grown at different TG. The A1 (670 cm−1) mode is related to the stretching of the metal–oxygen bond in the tetrahedral sites, and other low-frequency modes, including Eg (486 cm−1) and F2g (590 cm−1), correspond to the octahedral sites.10,27 It can be noted that with an increase in growth temperature, the peak patterns of the Alg and Eg vibration modes became symmetrical and narrower. We believe that the thin films would have metal cation defects at low temperatures, and the crystalline quality of the films gradually would increase with growth temperature. A new vibration mode F2g appeared when the temperature rose to 450 °C, probably due to the entry of Co2+ ions into the octahedra. Fig. 5(b) shows the infrared spectra of the FeCo2O4 epitaxial films grown at different TG. We found that the vibration modes of the films grown at 250 and 350 °C were similar. However, the larger epitaxial stress on the film grown at 350 °C had a more obvious effect on the octahedron than the tetrahedron. When the TG was increased from 250 °C to 350 °C, it was noted that the ν1 peak shifted to a higher wavenumber, and the intensity of the ν2 vibration increased, while the ν3 peak did not move. With increasing growth temperature, the occupation of ions will change. When the TG was further increased to 450–550 °C, the intensity of the ν3 vibration decreased, and the ν2 vibration broadened. The infrared spectrum of the film grown at 350 °C was deconvoluted into three peaks. The peak at 482 cm−1 (ν1) was from the vibration of octahedral Fe3+–O,28,29 and the vibration of the octahedral Co3+–O bond was located at 529 cm−1 (ν2).30 The signal at 630 cm−1 (ν3) is considered to arise from tetrahedral Co2+–O.31,32 When the growth temperature was increased to 450 °C, two other peaks
and
appeared (Fig. 5(c)). The
peak was caused by the vibrations of octahedral Co2+–O. The octahedral Co2+ ions have two possible sources: one is the tetrahedral Co2+ ions entering the octahedral sites, and the other is from the reduction of some octahedral Co3+ ions to Co2+ due to partially released oxygen. As more Co2+ enter the octahedral sites, more octahedral Fe3+ ions will migrate into the tetrahedral sites. The
vibration represented the vibration of tetrahedral Fe3+–O.28,29 Due to the fact that the films were partially decomposed at 650 °C,33 an increase in the intensity of the octahedral Co2+–O vibrations could be observed. More Co2+ at the octahedral sites would cause local antiferromagnetic interactions.34–36 The magnetic moments of the tetrahedral and octahedral cations were antiparallel, showing long-range ferrimagnetism. The coexistence of ferrimagnetism (FIM) and antiferromagnetism (AF) was confirmed by the following magnetic measurements.
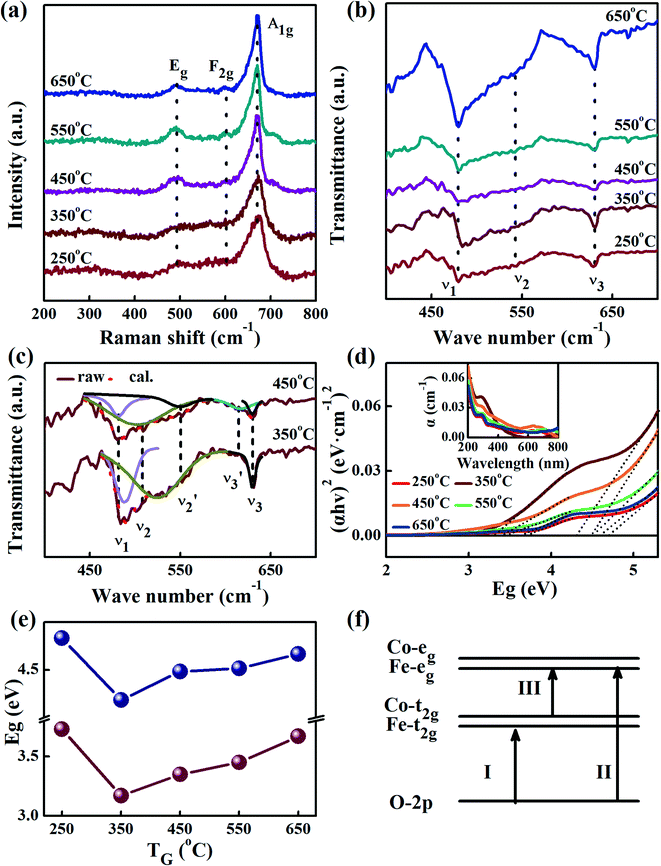 |
| Fig. 5 (a) Raman spectra of the FeCo2O4 films grown at different TG; (b) FTIR spectra of the FeCo2O4 films grown at different TG; (c) FTIR decomposition spectra of the FeCo2O4 films grown at 350–450 °C; (d) (αhν)2–Eg plots from the absorption spectra of the films grown at different TG. The inset shows the UV-vis absorption spectra of the FeCo2O4 films grown at different TG; (e) the two optical bandgaps of the film obtained by extrapolating (αhν)2 = 0; (f) band structure of FeCo2O4. | |
Fig. 5(d) shows the UV absorption spectra of the FeCo2O4 epitaxial films grown at different TG at room temperature. The inset shows the reflection spectra. The absorption bandgap energy, Eg, can be determined from the UV absorption spectra using the following equation:17
where
hν is the photon energy,
α is the absorption coefficient,
A is a constant for a given material, and
n = 1/2 for an indirect transition, while
n = 2 for an allowed directed transition.
37 In our case, the best fit indicated
n = 2. Similar to the report on NiCo
2O
4,
38 we also observed a double bandgap structure in the films at about 3.43 eV and 4.51 eV, as shown in
Fig. 5(e). We found that the film grown at 350 °C had the smallest bandgap.
Fig. 5(f) shows the three possible electronic transitions. I represents the transition from O 2p to Co 3d-t
2g (or Fe 3d-t
2g), II shows the transition from O 2p to Co 3d-e
g (or Fe 3d-e
g) and III represents the transition from Co 3d-t
2g to Co 3d-e
g (or Fe 3d-t
2g to Fe 3d-e
g). The electron transitions I and II correspond to the two direct bandgaps of the FeCo
2O
4 epitaxial films, respectively.
3.2. Magnetic properties
Fig. 6(a–e) shows the FC-ZFC curves of the FeCo2O4 films grown at different TG under a magnetic field of 500 Oe. It was observed that the magnetic moment in the ZFC curves gradually reached the maximum with an increase in the measurement temperature, and the temperature at which the maximum magnetization is reached in the ZFC curve is defined as TP. When we continued to increase the measurement temperature, the FC and ZFC curves coincided. With increasing TG, the magnetic moment gradually increased due to the decrease in APBs,39 and the TP first decreased and then increases (Fig. 6(f)). The TP moved to a lower temperature due to the partial decomposition of the film at 650 °C. The APBs in the film resulted in the local antiferromagnetism of spins,40 and a decrease in the saturation magnetization.41,42 It is worth noting that the ZFC curves of the films grown at 450–650 °C reached the maximum magnetization with two exceptional slopes, as shown by the arrows in Fig. 6(c–e). The temperatures at the inflection points of the two exceptional slopes are defined as T1 (∼100 K) and T2 (∼250 K), respectively. Below T1, the change in the magnetic moment with temperature could be ignored. When the measurement temperature was higher than T1, the magnetic moment increased gradually with increasing measurement temperature. However, when the measurement temperature was above T2, the magnetic moment increased rapidly. In the FeCo2O4 film, the tetrahedral and octahedral magnetic moments are thought to be antiparallel, forming a ferrimagnetic phase. There would also be two kinds of antiferromagnetic phases: one kind of antiferromagnetism is localized in the octahedra formed by the Co2+ ions (defined as α-AF),35,36 and the other is from the APBs (defined as β-AF). The antiferromagnetism between octahedral Co2+ is within the lattice, while the antiferromagnetism from the APBs is caused by the interactions between crystalline grains. In contrast, α-AF is more susceptible to temperature. In a small field of 500 Oe, the magnetic moment of the film would be in a chaotic frozen state at low temperatures. When the measurement temperature rises to T1, due to the thermal disturbance, α-AF becomes weak, and the magnetic moment of the film gradually increases. When the measurement temperature is higher than T2, β-AF decreases with an increase in the measurement temperature, and the magnetic moment increases rapidly. Of the two antiferromagnetisms with different origins in these films, we believe that there is more β-AF in the films grown at lower TG and more α-AF in those grown at higher TG. When the TG is low (250–350 °C), the film has poor crystalline quality, and many APBs exist in the film. The magnetism in the films is mainly dominated by β-AF and FIM. In addition, lattice distortion weakens the effective magnetocrystalline anisotropy, which lowers the TP. In order to confirm the existence of two antiferromagnetisms in the films, we further measured the hysteresis loops of the films.
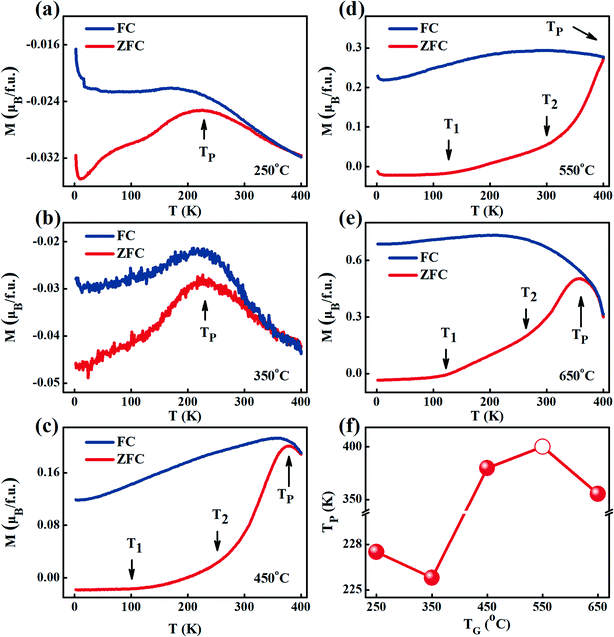 |
| Fig. 6 (a–e) FC-ZFC curves measured at a magnetic field of 500 Oe, and (f) changes in the TP of the FeCo2O4 films grown at different TG. | |
Fig. 7(a–e) shows the M–H curves of the films grown at different TG in the measurement temperature range of 2–400 K. It was found that the saturation magnetization (MS) increased with TG, which indicated that the magnetic moments in the films were enhanced, consistent with the results obtained from the MFM observation and FC-ZFC curves. Similar to our previous results for powder samples, Barkhausen jumps were observed in the hysteresis loops below 100 K,43,44 as shown in the inset of Fig. 7(c). The jump phenomenon disappeared with an increase in the measurement temperature. Barkhausen jumps commonly occur due to the irreversible motion of the domain walls between two regions of opposite magnetic moments.45,46 In our films, the Barkhausen jumps are considered to arise from the pinning of the AF to FIM. To confirm the existence of different magnetic phases in the films,47 we took the film grown at 450 °C as an example. With the increase in TG, the APBs gradually decrease, while β-AF reduces, and the hysteresis loops of the films, therefore, tended to be saturated. The saturation magnetization and coercivity (HC) obtained from Fig. 7(a–e) are given in Fig. 7(f). Two extreme values of MS were observed at about 100 K and 250 K for all the films, which further proved the existence of two kinds of antiferromagnetism in the films. The coercivity decreased gradually with on increasing the measurement temperature, and reduced to 0 Oe at about 250 K. With the measurement temperature, the magnetic phase change in the films is consistent with that in the FC-ZFC test. The coercivity of the film grown at 650 °C was smaller than those of the films grown at 450 and 550 °C, indicating that the film was partially decomposed into soft magnetic materials at high TG.48 Based on the above analysis, we think that there could be one kind of ferrimagnetic phase and two kinds of antiferromagnetic phases in the FeCo2O4 films.
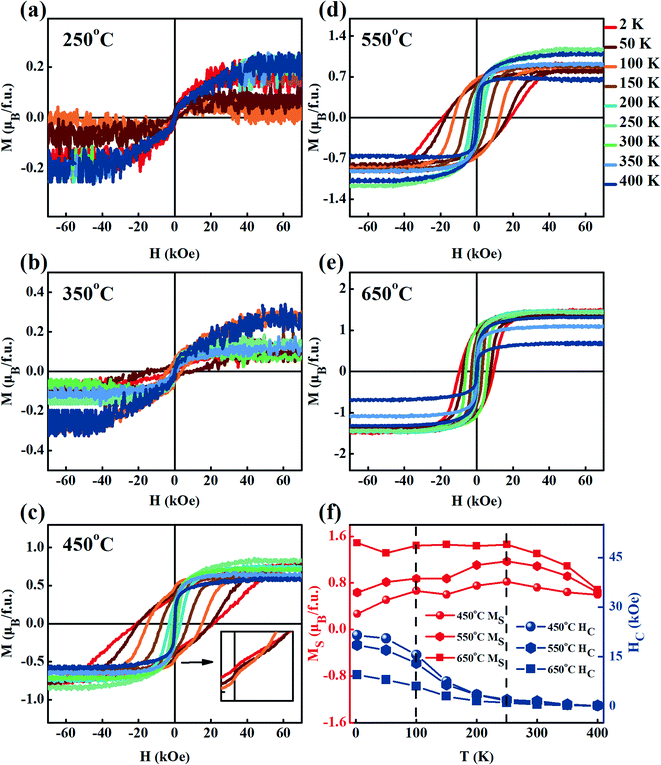 |
| Fig. 7 (a–e) Hysteresis loops in the temperature range of 2–400 K corresponding to the FeCo2O4 films grown at different TG. The inset in (c) is the enlarged view of the low field; (f) MS and HC values of the films grown at 450 °C, 550 °C and 650 °C in a range of measurement temperatures. | |
3.3. Electrical transport properties
Fig. 8(a–e) shows the resistivities and conductivities of the FeCo2O4 films grown at different TG. It was found that the resistivity of the FeCo2O4 film increased with a decrease in the measurement temperature, showing characteristic semiconductor behavior. A significant similarity could also be found in the TG dependency of the room-temperature resistivities (= 300 K) and the out-of-plane lattice constants (Fig. 2(c) and 8(f), respectively). Similar findings were reported by Silwal et al.49 The electron transport process mainly occurs in the plane of the film, and the in-plane interatomic spacing determines the number of times an electron is scattered. If the in-plane interatomic spacing is small, the electron suffers more scatterings and the resistivity increases. Assuming that the out-of-plane is large when the unit cell volume is unchanged, then the in-plane lattice constant is small, resulting in large resistivity.
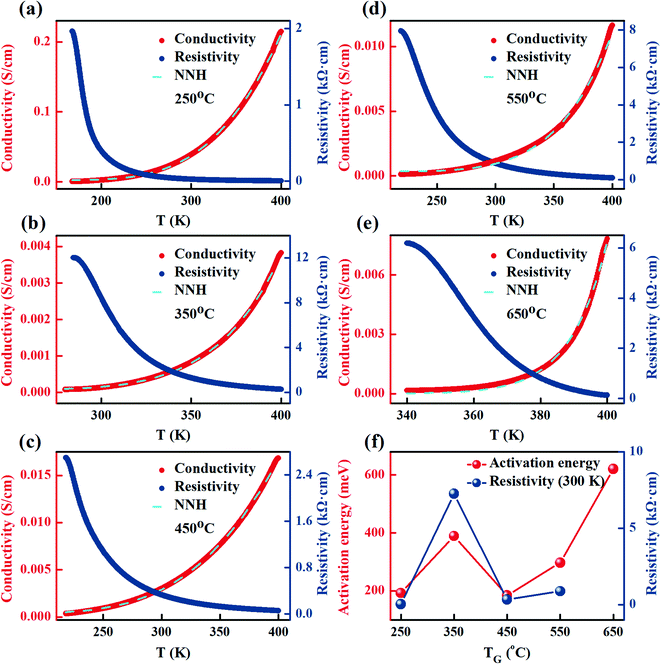 |
| Fig. 8 (a–e) Temperature dependence of the conductivities and resistivities of the FeCo2O4 films grown at different TG and the NNH fitting results; (f) activation energies obtained from the NNH fitting results, and the room-temperature resistivities of the FeCo2O4 films grown at different TG. | |
In order to explore the conductive mechanism of the films, we used the nearest-neighbor hopping mechanism (NNH) to fit the conductivity values:50
where
σ is the measured conductivity,
a is a constant,
k is the Boltzmann constant, and Δ
E is the activation energy. The fitting curves of the films were in good agreement with the raw data (
Fig. 8(a–e)). The changes in activation energy with the measurement temperature were obtained, as shown in
Fig. 8(f). The film grown at 450 °C had the lowest activation energy at 184.5 meV. The structure of the film grown at 450 °C was more ordered. The proposed conduction process is the charge transfer between neighboring octahedral Co
2+ and Co
3+.
Fig. 9(a–e) shows the schematic of the growth mode and microstructures of the FeCo2O4 films grown on the Al2O3 substrate under TG. Four regions have been defined to explain the changes in the microstructure of the FeCo2O4 films with growth temperature. Region-A is the normal FeCo2O4 structure, Region-B shows the FeCo2O4 structure containing more APBs, Region-C represents the FeCo2O4 structure with the octahedra occupied by more Co2+, and “Region-D” is the FeCo2O4 structure with the decomposition phase. Under low TG, particle aggregation is observed in the film, and the film contains more APBs (Region-B). With the increase in TG, the atomic deposition mode gradually changes to layered growth. Due to the effect of substrate stress, the APBs dominate in the structure (Region-B). At 450–550 °C TG, the growth temperature is high enough, and the APBs decrease. More Co2+ ions enter the octahedral sites (Region-C). When the TG is higher than 650 °C, the FeCo2O4 film is partially decomposed (Region-D).
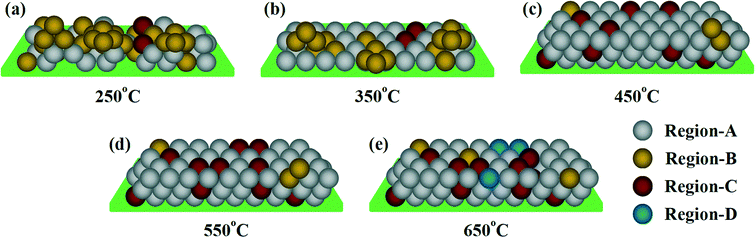 |
| Fig. 9 (a–e) Schematic diagram of the growth mode and microstructures of the FeCo2O4 films on the Al2O3 substrate; the green areas denote the substrate, Region-A is the normal FeCo2O4 structure, Region-B is the FeCo2O4 structure containing more APBs, Region-C is the FeCo2O4 structure with the octahedra occupied by more Co2+, and Region-D is the FeCo2O4 structure with the decomposition phase. | |
4. Conclusions
We have grown a series of FeCo2O4 films at different TG (250–650 °C) on Al2O3 (0001) substrates by PLD. The results show that with increasing TG, due to the difference in ionic mobility, the atomic growth mode changed from the island and layered growth to layered growth. The growth temperature directly affected cation occupation, resulting in different magnetic phases. It was found that with an increase in growth temperature, the magnetic domains of the films became more obvious, and the magnetic moment became stronger. In addition to one ferrimagnetic phase, two kinds of antiferromagnetic phases coexisted in the films: one kind from the antiphase boundary and the localized antiferromagnetism contributed by the octahedral Co2+ ions. With rising growth temperature, the antiferromagnetism from the antiphase boundaries decreased, while the antiferromagnetism formed by octahedral Co2+ gradually increased. With the increase in measurement temperature, the antiferromagnetism formed by the antiphase boundary first decreased, and then the antiferromagnetism formed by Co2+ decreased, and the magnetic moment increased. It was observed by UV absorption that there were double direct bandgaps in the FeCo2O4 epitaxial films. Electrical measurements showed that the film grown at 450 °C had the lowest activation energy, and the structure of this film was more ordered. The room temperature resistivity of the films was closely related to the out-of-plane lattice constants.
Author contributions
Jingtong Xie: conceptualization, methodology, data curation, formal analysis, investigation, visualization, writing-original draft. Congmian Zhen: funding acquisition, project administration, resources, supervision, validation, writing-review & editing. Lei Xu: methodology, formal analysis, investigation, visualization, writing-review & editing. Mengyao Su: methodology, formal analysis, investigation, visualization, writing-review & editing. Chengfu Pan: funding acquisition, investigation. Li Ma: funding acquisition, resources, investigation. Dewei Zhao: funding acquisition, resources, investigation. Denglu Hou: funding acquisition, project administration, resources, supervision, validation, writing-review & editing.
Conflicts of interest
There are no conflicts to declare.
Acknowledgements
This work was supported by the Key Project of Natural Science of Hebei Higher Education (grant numbers ZD2017045 and QN2019154), the National Natural Science Foundation of China (grant numbers 51971087 and 51901067), and the Natural Science Foundation of Hebei Province (grant numbers A2018205144, A2017210070, and E2019205234).
References
- E. Ferreiro-Vila, L. Iglesias, I. L. del Pozo, N. Varela-Dominguez, C. T. Bui, B. Rivas-Murias, J. M. Vila-Fungueirino, P. Jimenez-Cavero, C. Magen, L. Morelion, V. Pardo and F. Rivadulla, APL Mater., 2019, 7, 031109 CrossRef.
- T. A. S. Ferreira, J. C. Waerenborgh, M. H. R. M. Mendonça, M. R. Nunes and F. M. Costa, Solid State Sci., 2003, 5, 383–392 CrossRef CAS.
- P. Lavela, G. F. Ortiz, J. L. Tirado, E. Zhecheva, R. Stoyanova and S. Ivanova, J. Phys. Chem. C, 2007, 111, 14238–14246 CrossRef CAS.
- S. Kawano, N. Achiwa, N. Yamamoto and S.-N. Higashi, Mater. Res. Bull., 1976, 11, 911–916 CrossRef CAS.
- D. Santos-Carballal, A. Roldan, R. Grau-Crespo and N. H. de Leeuw, Phys. Rev. B: Condens. Matter Mater. Phys., 2015, 91, 195106 CrossRef.
- R. N. Bhowmik and I. P. Muthuselvam, J. Alloys Compd., 2014, 589, 247–257 CrossRef CAS.
- R. Zhou, J. Zhao, N. Shen, T. Ma, Y. Su and H. Ren, Chemosphere, 2018, 197, 670–679 CrossRef CAS.
- G. Kamble, A. Kashale, A. Rasal, S. Dengale, S. Kolekar, J. Y. Chang, S. H. Han and A. Ghule, ChemistrySelect, 2021, 6, 1838–1844 CrossRef CAS.
- D. F. Zhang, G. Z. Zhang and L. Zhang, Chem. Eng. J., 2017, 330, 792–803 CrossRef CAS.
- Y. Zhou, Y. Zhang and X. Hu, Colloids Surf., A, 2020, 597, 124568 CrossRef CAS.
- S. G. Mohamed, C. J. Chen, C. K. Chen, S. F. Hu and R. S. Liu, ACS Appl. Mater. Interfaces, 2014, 6, 22701–22708 CrossRef CAS.
- Y. Y. Liao, Y. W. Li, Z. G. Hu and J. H. Chu, Appl. Phys. Lett., 2012, 100, 071905 CrossRef.
- J. T. Xie, C. M. Zhen, L. Liu, L. Ma, D. L. Hou, H. Q. Pang and D. W. Zhao, Ceram. Int., 2021, 47, 11993–12001 CrossRef CAS.
- Y. S. Han, J. B. Li, X. S. Ning and B. Chi, J. Am. Ceram. Soc., 2004, 87, 1347–1349 CrossRef CAS.
- J. M. Li, X. L. Zeng and Z. A. Xu, Appl. Phys. Lett., 2013, 103, 232410 CrossRef.
- J. M. Li, A. Huan, L. Wang, Y. W. Du and D. Feng, Phys. Rev. B: Condens. Matter Mater. Phys., 2000, 61, 6876–6878 CrossRef CAS.
- W. Z. Guo, C. M. Zhen, C. F. Wu, X. C. Wu, G. K. Li, L. Ma and D. L. Hou, Ceram. Int., 2018, 44, 12539–12546 CrossRef CAS.
- K. Q. Zhang, C. M. Zhen, W. G. Wei, W. Z. Guo, G. D. Tang, L. Ma, D. L. Hou and X. C. Wu, RSC Adv., 2017, 7, 36026–36033 RSC.
- V. Sowjanya, K. V. Bangera and G. K. Shivakumar, J. Alloys Compd., 2017, 715, 224–229 CrossRef CAS.
- A. Bhogra, A. Masarrat, D. Hasina, R. Meena, G. R. Umapathy, A. Kumar, T. Som, C. L. Dong, C. L. Chen and A. Kandasami, Surf. Coat. Technol., 2021, 407, 126740 CrossRef CAS.
- P. Mele, S. Saini, H. Honda, K. Matsumoto, K. Miyazaki, H. Hagino and A. Ichinose, Appl. Phys. Lett., 2013, 102, 253903 CrossRef.
- M. Oujja, L. Martin-Garcia, E. Rebollar, A. Quesada, M. A. Garcia, J. F. Fernandez, J. F. Marco, J. de la Figuera and M. Castillejo, Appl. Surf. Sci., 2018, 452, 19–31 CrossRef CAS.
- B. Talic, P. V. Hendriksen, K. Wiik and H. L. Lein, Solid State Ionics, 2018, 326, 90–99 CrossRef CAS.
- M. Wang, X. Sui, Y. Wang, Y. H. Juan, Y. Lyu, H. Peng, T. Huang, S. Shen, C. Guo, J. Zhang, Z. Li, H. B. Li, N. Lu, A. T. N'Diaye, E. Arenholz, S. Zhou, Q. He, Y. H. Chu, W. Duan and P. Yu, Adv. Mater., 2019, 31, e1900458 CrossRef.
-
M. Donahue and D. Porter, OOMMF User's Guide, http://math.nist.gov/oommf/, September 30, 2021.
-
S. Chikazumi, Physics of Ferromagnetism, Oxford University Press, New York, 2009 Search PubMed.
- Z. Wang, R. T. Downs, V. Pischedda, R. Shetty, S. K. Saxena, C. S. Zha, Y. S. Zhao, D. Schiferl and A. Waskowska, Phys. Rev. B: Condens. Matter Mater. Phys., 2003, 68, 094101 CrossRef.
- K. Yang, H. B. Peng, Y. H. Wen and N. Li, Appl. Surf. Sci., 2010, 256, 3093–3097 CrossRef CAS.
- T. Ozkaya, M. S. Toprak, A. Baykal, H. Kavas, Y. Köseoğlu and B. Aktaş, J. Alloys Compd., 2009, 472, 18–23 CrossRef CAS.
- E. Alizadeh-Gheshlaghi, B. Shaabani, A. Khodayari, Y. Azizian-Kalandaragh and R. Rahimi, Powder Technol., 2012, 217, 330–339 CrossRef CAS.
- R. N. Singh, J. P. Pandey, N. K. Singh, B. Lal, P. Chartier and J.-F. Koenig, Electrochim. Acta, 2000, 45, 1911–1919 CrossRef CAS.
- M. Y. Nassar, Mater. Lett., 2013, 94, 112–115 CrossRef CAS.
- D. Byrne, A. Cowley, P. McNally and E. McGlynn, CrystEngComm, 2013, 15, 6144–6150 RSC.
- T. Maurer, F. Zighem, F. Ott, G. Chaboussant, G. Andre, Y. Soumare, J. Y. Piquemal, G. Viau and C. Gatel, Phys. Rev. B: Condens. Matter Mater. Phys., 2009, 80, 064427 CrossRef.
- N. J. O. Silva, A. Millan, F. Palacio, M. Martins, T. Trindade, I. Puente-Orench and J. Campo, Phys. Rev. B: Condens. Matter Mater. Phys., 2010, 82, 094433 CrossRef.
- Q. Zhang, G. X. Cao, F. Ye, H. B. Cao, M. Matsuda, D. A. Tennant, S. X. Chi, S. E. Nagler, W. A. Shelton, R. Y. Jin, E. W. Plummer and J. D. Zhang, Phys. Rev. B, 2019, 99, 094416 CrossRef CAS.
- T. Xie, M. R. Hasan, B. Qiu, E. S. Arinze, N. V. Nguyen, A. Motayed, S. M. Thon and R. Debnath, Appl. Phys. Lett., 2015, 107, 101902 CrossRef.
- Z. C. Liu, C. M. Zhen, D. Y. Xu, X. C. Wu, H. R. Wang, L. Ma, D. W. Zhao, Z. X. Tian and D. L. Hou, Appl. Surf. Sci., 2020, 529, 147155 CrossRef CAS.
- R. Guzman, J. Heuver, S. Matzen, C. Magen and B. Noheda, Phys. Rev. B, 2017, 96, 104105 CrossRef.
- S. Tiwari, D. M. Phase and R. J. Choudhary, Appl. Phys. Lett., 2008, 93, 234108 CrossRef.
- A. Kumar, D. K. Pandya and S. Chaudhary, Appl. Phys. Lett., 2013, 102, 152406 CrossRef.
- X. H. Liu, A. D. Rata, C. F. Chang, A. C. Komarek and L. H. Tjeng, Phys. Rev. B: Condens. Matter Mater. Phys., 2014, 90, 125142 CrossRef.
- G. Huang, X. Li, L. Lou, Y. Hua, G. Zhu, M. Li, H. T. Zhang, J. Xiao, B. Wen, M. Yue and X. Zhang, Small, 2018, 14, 1800619 CrossRef.
- C. Wang, C. Chen, C. H. Chang, H. S. Tsai, P. Pandey, C. Xu, R. Bottger, D. Chen, Y. J. Zeng, X. Gao, M. Helm and S. Zhou, ACS Appl. Mater. Interfaces, 2018, 10, 27472–27476 CrossRef CAS PubMed.
- P. Kaur, K. K. Sharma, R. Pandit, R. J. Choudhary and R. Kumar, Appl. Phys. Lett., 2014, 104, 081608 CrossRef.
- R. Palai, H. Huhtinen, J. F. Scott and R. S. Katiyar, Phys. Rev. B: Condens. Matter Mater. Phys., 2009, 79, 104413 CrossRef.
- V. Trannoy, A. Bordage, J. Dezalay, R. Saint-Martin, E. Riviere, P. Beaunier, C. Baumier, C. La Fontaine, G. Fornasieri and A. Bleuzen, CrystEngComm, 2019, 21, 3634–3643 RSC.
- J. H. Kim, H. Kim, D. Kim, Y. Ihm and W. K. Choo, J. Eur. Ceram. Soc., 2004, 24, 1847–1851 CrossRef CAS.
- P. Silwal, L. Miao, I. Stern, X. L. Zhou, J. Hu and D. H. Kim, Appl. Phys. Lett., 2012, 100, 032102 CrossRef.
- N. F. Mott, Adv. Phys., 1967, 16, 49–144 CrossRef CAS.
|
This journal is © The Royal Society of Chemistry 2022 |
Click here to see how this site uses Cookies. View our privacy policy here.