DOI:
10.1039/C5EE03149D
(Communication)
Energy Environ. Sci., 2016,
9, 102-106
Nitrogen-doped activated carbon for a high energy hybrid supercapacitor†
Received
14th October 2015
, Accepted 11th November 2015
First published on 18th November 2015
Abstract
Nitrogen-doped activated carbons (NACs) were prepared through a one-step process. The obtained NACs show high surface areas of up to 2900 m2 g−1 with a moderate N content of up to 4 wt%. Electrochemical evaluation of the NACs shows a high specific capacity of 129 mA h g−1 (185 F g−1) in an organic electrolyte at a current density of 0.4 A g−1, as well as excellent rate capability and cycling stability. The hybrid-type supercapacitor assembled using the NACs and a Si/C electrode exhibits a high material level energy density of 230 W h kg−1 at 1747 W kg−1. The hybrid device achieved 76.3% capacity retention after 8000 cycles tested at 1.6 A g−1.
Broader context
The present work provides a novel one-step synthesis for nitrogen-doped activated carbon. The excellent performance of the N-doped AC allows its further application in a hybrid-type supercapacitor, which utilizes a combination of the capacitor electrode and a Li-ion battery anode. The special configuration can much improve the energy density, which could be several times that of a traditional electric double-layer supercapacitor, without sacrificing the power density. The N-doped AC and corresponding hybrid supercapacitor presented in this work can provide a good example for novel material and system design for advanced energy storage systems.
|
In recent years, the rapidly growing commercial electrical device market evokes a high demand of novel energy storage systems that can provide higher energy and power than traditional systems.1,2 Besides lithium-ion batteries, supercapacitors have been recognized as promising systems for power-based applications. Compared with traditional dielectric capacitors, supercapacitors can provide higher energy density while maintaining a high power output.3 However, although they provide an extremely long cycling life, their relatively low energy-to-power ratio hinders their further application in some areas where both energy and power are required, such as hybrid electrical vehicles (HEV). In order to further improve the performance, novel capacitor systems utilizing different surface chemistry and device configuration have been proposed and investigated. Recently, hybrid-type supercapacitors (HSs), or so-called supercapacitor–battery hybrid systems, have attracted attention due to their high energy/power ratio, compared with traditional electron double-layer capacitors (EDLC). Such systems utilize a traditional capacitor electrode as one electrode via the sorption of ions, and a lithium-ion battery (LIB) electrode as the counter electrode via Li ion insertion/intercalation.4,5 The application of promising high energy LIB electrode materials helps to improve the performance of the hybrid system significantly. Several examples have been reported with exciting results.6–10 Among these, the configuration using high energy LIB anode materials, such as silicon, could provide higher energy densities and power densities.8 However, the energy and power densities of a device are determined by the cathode part, due to the asymmetrical configuration and the huge difference in specific capacity of the cathode and anode. Therefore, further improvement of the system level performance is still prohibited by cathode materials, which always have a much lower specific capacity (or capacitance) than that of anode materials.1 The same issue has been emphasized in LIB studies.11,12 Thus, the development of cathode materials for HSs with improved electrochemical performance is highly necessary.
Activated carbons (ACs) have been widely used as the active material in many types of supercapacitors, due to their high surface area and porous structure, which are important to the final power and energy storage capacity.4,13,14 Other advantages such as low cost and industrial large-scale production, which are also superior to other types of porous materials, are critical for the application of ACs in commercial capacitor devices too.14 Unfortunately, common physical or chemical activation methods always produce a wide range of pore size distribution, ranging from micro-size to macro-size.15 The specific capacitance of such kinds of ACs is thus limited, despite having high surface areas of up to 3000 m2 g−1. Therefore, novel activation or preparation methods providing a narrow pore size distribution and controllable pore structure are necessary for the further development of AC materials.
Besides structure tuning, proper surface functionality also helps to improve the performance of AC materials. Recently, nitrogen-doping was found to help increase the specific capacitance of AC materials effectively.16–20 Possible explanations for the capacitance increase are the faradaic reaction of the nitrogen-containing functional groups and improving the wettability of the pore walls. Some recent research also suggests that the heteroatom doping can increase the conductivity of porous carbon materials.21–24
Herein we report a novel one-step process for the preparation of nitrogen-doped ACs (NACs), utilizing agricultural waste (corncob) as a precursor. The obtained NACs had a narrow micro- to meso-pore distribution and showed a high specific surface area of up to 2859 m2 g−1 with a moderate N content of up to 4 wt%. The NACs delivered a high specific capacity of up to 129 mA h g−1 (185 F g−1) in an organic electrolyte at a current density of 0.4 A g−1, as well as an excellent rate capability and much improved cycle stability. The HS was assembled using the NAC and Si/C electrode materials and showed an energy density of 230 W h kg−1 at a power density of 1747 W kg−1. Even at an elevated working power density of 30
127 W kg−1, such a supercapacitor could still provide an energy density of 141 W h kg−1. To our knowledge, this is the highest value among previously reported literature results. This HS device also showed a good cycling stability with 76.3% capacity retention after 8000 cycles at 1.6 A g−1.
Results and discussion
Synthesis
In this work, corncobs were utilized as the precursor for NAC preparation. The corncobs were pre-dried at 120 °C for 12 hours before being ground and sieved. The powders were mixed with KOH followed by heat treatment at different temperatures under N2 flow with NH3 as a nitrogen source. Three NAC materials, namely NAC-x where x is the heat treatment temperature, were prepared and used for further characterization and electrochemical performance evaluation.
Characterizations
The bulk of the NAC particles are micron-sized ranging from 5 to 30 μm, as shown in Fig. 1A and Fig. S1 (ESI†). There are no observable macro-sized pore or large meso-sized pore based on the TEM images of all NACs shown in Fig. 1B–D. The nitrogen content of all samples was determined by elemental analysis. As the heat treatment temperature increases, the nitrogen content increases from 2.97 wt% for NAC-400 to 3.98 wt% for NAC-600. Oxygen content was also determined along with nitrogen content. NAC-400 shows an oxygen content of 9.8 wt%, while NAC-600 has an oxygen content of 9.05 wt%. All NACs show similar C and O signals (C1s, O1s) in the XPS spectra (Fig. 2A and Fig. S2, ESI†). Typical C1s, O1s and N1s of the synthesized carbon (NAC-400) are shown in Fig. 2A. The C1s peak is dominated by a C–C bond (diamond and graphite type) at 248.8 eV. A small amount of ethereal carbon (C–OH, 286 eV) and carbonyl carbon (C
O, 290 eV) can also be detected from the XPS data for both the C1s and O1s (531.5 eV and 532.5 eV).25,26 These types of surface functional groups are well known to increase the capacity of the engineered carbon for their fast reaction with lithium.27 The N1s peak is mainly composed of pyridinic N (399.7 eV), together with a small amount of N-oxides (402 eV).14,28,29 In contrast, NAC-600 has two peaks in the same range instead of one, as shown in Fig. S2 (ESI†). The contribution from the N-oxides is more than that for NAC-400. Raman spectra of the NACs show intensive G bands, suggesting the partial graphitisation of all activated carbon materials (Fig. 2B).30 With higher heat treatment temperatures, much more graphitisation is obtained for the NAC materials, as shown in Fig. 2B.
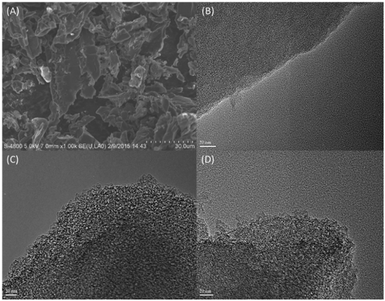 |
| Fig. 1 (A) SEM image of NAC-400, TEM image of (B) NAC-0, (C) NAC-400, and (D) NAC-600. | |
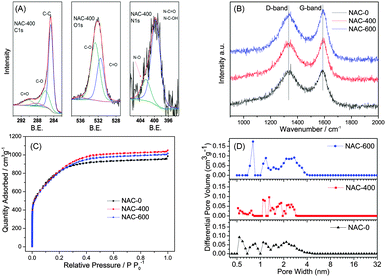 |
| Fig. 2 (A) XPS spectra of NAC-400, (B) Raman spectra of all NACs, (C) nitrogen adsorption isotherms at 77 K, and (D) pore-size distribution of NACs. | |
The pore structure of the NACs was analyzed by N2-sorption at 77 K. The isotherm plots in Fig. 2C clearly show type I isotherm curves with well-defined plateaus, suggesting a microporous nature of the NACs. The pore size distributions were calculated using Density Functional Theory (DFT) and are shown in Fig. 2D. All samples have pore distribution peaks between 0.5 and 5 nm, which suggests the formation of both micropores and small mesopores. As the heat treatment temperature increases, the pore size distribution peaks in the mesopore range become broader, suggesting the formation of slightly larger size mesopores. All NACs show similar high BET specific surface areas of 2759, 2859 and 2787 m2 g−1 for NAC-0, NAC-400 and NAC-600, respectively.
Electrochemical performance
The electrochemical performance of the NACs was evaluated using a coin format cell. The capacities were read from the instrument by the galvanostatic charge/discharge method. The corresponding specific capacitance was calculated based on the capacity (see ESI†). Fig. 3A shows the cycling performance of all the NAC materials at a current density of 0.4 A g−1. Both NAC-0 and NAC-400 show high initial specific capacities of 129 mA h g−1 (185 F g−1) and 127 mA h g−1 (182 F g−1), respectively. In contrast, the NAC-600 has a lower initial capacity of 110 mA h g−1. All NAC materials show good cycling stabilities and Coulombic efficiencies (CEs). NAC-400 shows the best capacity retention of about 86% (110 mA h g−1) after 500 cycles among all samples. The cycling CE is above 99.2% after the initial cycles. The cyclic voltammetry (CV) profiles of the NACs maintain a quasi-rectangular shape at a scan rate of 5 mV s−1, indicating the capacitive behavior of all samples (Fig. 3B). Fig. 3C shows the performance of the NAC materials under various current densities. The difference in the specific capacities of all samples becomes more and more distinct with increasing current density. At a low current density of 0.4 A g−1, all three NAC materials show similar specific capacities above 110 mA h g−1. However, at elevated current densities of 0.8 A g−1 and 1.6 A g−1, the specific capacity of NAC-600 drops to significantly lower than the other two materials. At a high current density of 12.8 A g−1 (128 C), the NAC-400 provides a capacity of 73.7 mA h g−1, which is 15% and 33% higher than those of NAC-0 (63.8 mA h g−1) and NAC-600 (55.3 mA h g−1) respectively.
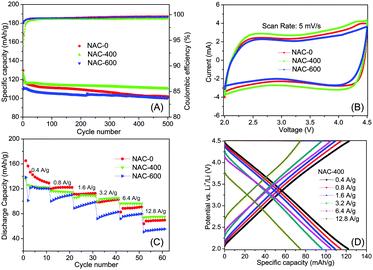 |
| Fig. 3 (A) Cycling performance of all NAC materials, (B) CV diagram of all NAC materials at scan rate of 5 mV s−1, (C) rate performance of all NAC materials, and (D) voltage profile of NAC-400 at different current densities. | |
The electrochemical performance of the NAC materials is considered depending on the N-doping of the materials. Both the specific capacity and cycling stability were improved by increasing the N content from 0 wt% in NAC-0 to 2.97 wt% in NAC-400. However, when the N-doping further increased to 3.98 wt% for NAC-600, the electrochemical performance, including the reversible capacity, capacity retention, and rate performance, becomes worse compared to that of NAC-400. The much increased oxidized pyridinic N found in NAC-600 is considered a key factor, resulting in the capacity drop due to the positive charge on N, which will affect the ion adsorption.20 The slightly reduced BET surface area of NAC-600 also affects the specific capacity drop.
The N-doping of NACs also helps to improve the conductivity, as supported by electrochemical impedance spectroscopy (EIS) (Fig. S3, ESI†). In particular, the diameter of the kinetic loop corresponds to the charge transfer resistance of NAC-400, which has a strong impact on the specific capacity and is much smaller than that of NAC-0. The linear galvanostatic charge/discharge profiles of NAC-400 were tested at 0.4–12.8 A g−1, indicating capacitive behavior with the adsorption/desorption of ions on the electrode surface, as shown in Fig. 3D.
The HS full cell was assembled using NAC-400 as the cathode and Si/C as the anode materials (see ESI†). During the charge process, PF6− ions were absorbed into the porous structure of the NAC, while Li+ ions from the electrolyte were alloyed with the Si/C anode. The discharge process was the reverse of the charge process. The mass ratio of the electrode active materials was optimized to 2
:
1 for the best electrochemical performance and energy/power density (see ESI†).
The CV profile of the HS shows a gradual deviation from the ideal rectangular shape with increasing scan rate, owing to the overlapping effects of two different energy-storage mechanisms (Fig. 4A). This observation is consistent with the voltage profile of the HS using galvanostatic charge/discharge method, which shows little deviation from the linear slope (Fig. 4B). The HS shows good cycling stability of 76.3% capacity retention in 8000 cycles at a high current density of 1.6 A g−1 (Fig. 4C). The Coulombic efficiency of the full cell is relative high, with an average value of 99.8%. The Ragone plot (power density vs. energy density, material level) of the HS is shown in Fig. 4D. The energy density and power density were calculated based on the total mass of active materials on both the cathode and the anode. The detailed calculation method of the energy and power densities is in the ESI.† The HS shows a high energy density of 230 W h kg−1 at 1747 W kg−1, which also remains at 141 W h kg−1 even when the power density is elevated to 30
127 W kg−1. Table S2 (ESI†) lists some Li-ion hybrid systems with typical energy and power densities for an approximate comparison, such as AC//hard carbon,31 AC//soft carbon,32 AC//LTO,33 AC//B-Si/SiO2/C,8 3D graphene//Fe3O4/graphene,7 CNS//MnO/CNS,9 as well as an example of a Na-ion hybrid system PSNC//PSOC-A.34 The performance of this work is still quite promising and is among the best reported for hybrid type supercapacitors.
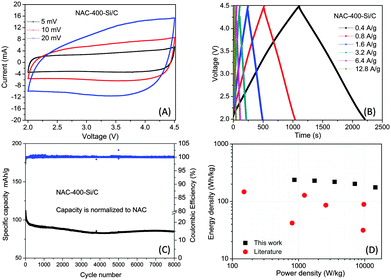 |
| Fig. 4 (A) CV diagram of the NAC-400-Si/C HS full cell at different scan rates, (B) voltage profile of the NAC-400-Si/C HS, (C) long cycling performance of the NAC-400-Si/C HS at 0.4 A g−1, specific capacity is normalized to NAC-400, and (D) Ragone plot of the NAC-400-Si/C HS. | |
We also found that a narrower voltage window helps to increase the long cycling stability, although it sacrifices the energy density. When the voltage window changes to 2–4.0 V, the energy density reduces by 30% at the same power density, while the cycling performance improves with a capacity retention of 88% after 8000 cycles (Fig. S4 and Table S1, ESI†).
Conclusions
In summary, novel N-doped activated carbons were synthesized via a one-step method. The obtained NAC materials showed excellent electrochemical performance with a specific capacity of up to 129 mA h g−1 and capacity retention of 86% in 500 cycles. The N-doping of the NACs was found to help improve the performance. The hybrid-type supercapacitor was further assembled and evaluated utilizing NAC and Si/C nanocomposites. With the optimized N/P ratio, the hybrid system showed high energy densities of 230–141 W h kg−1 at power densities from 1747 to 30
127 W kg−1, which are among the highest numbers reported for hybrid-type systems. A good long cycling stability was also obtained with a capacity retention of 76.3% after 8000 cycles for the hybrid-type supercapacitor.
Acknowledgements
The authors appreciate the General Motors project (Project No. 1550) and the International Postdoctoral Exchange Fellowship Program China (201372) for the financial support. We also thank Dr Peng Lu (General Motors) for his help with the XPS analyses.
References
- G. Wang, L. Zhang and J. Zhang, Chem. Soc. Rev., 2012, 41, 797–828 RSC.
- M. Armand and J.-M. Tarascon, Nature, 2008, 451, 652–657 CrossRef CAS PubMed.
- P. Sharma and T. S. Bhatti, Energy Convers. Manage., 2010, 51, 2901–2912 CrossRef CAS.
- Y. Wang and Y. Xia, Adv. Mater., 2013, 25, 5336–5342 CrossRef CAS PubMed.
- G. G. Amatucci, F. Badway, A. Du Pasquier and T. Zheng, J. Electrochem. Soc., 2001, 148, A930 CrossRef CAS.
- Q. Wang, Z. H. Wen and J. H. Li, Adv. Funct. Mater., 2006, 16, 2141–2146 CrossRef CAS.
- F. Zhang, T. Zhang, X. Yang, L. Zhang, K. Leng, Y. Huang and Y. Chen, Energy Environ. Sci., 2013, 6, 1623–1632 CAS.
- R. Yi, S. Chen, J. Song, M. L. Gordin, A. Manivannan and D. Wang, Adv. Funct. Mater., 2014, 24, 7433–7439 CrossRef CAS.
- H. Wang, Z. Xu, Z. Li, K. Cui, J. Ding, A. Kohandehghan, X. Tan, B. Zahiri, B. C. Olsen, C. M. B. Holt and D. Mitlin, Nano Lett., 2014, 14, 1987–1994 CrossRef CAS PubMed.
- K. Leng, F. Zhang, L. Zhang, T. Zhang, Y. Wu, Y. Lu, Y. Huang and Y. Chen, Nano Res., 2013, 6, 581–592 CrossRef CAS.
- M. S. Whittingham, Chem. Rev., 2004, 104, 4271–4302 CrossRef CAS PubMed.
- J. B. Goodenough and Y. Kim, Chem. Mater., 2010, 22, 587–603 CrossRef CAS.
- L. L. Zhang and X. S. Zhao, Chem. Soc. Rev., 2009, 38, 2520–2531 RSC.
- A. G. Pandolfo and A. F. Hollenkamp, J. Power Sources, 2006, 157, 11–27 CrossRef CAS.
- M. Inagaki, H. Konno and O. Tanaike, J. Power Sources, 2010, 195, 7880–7903 CrossRef CAS.
- J. Lee, J. Kim and T. Hyeon, Adv. Mater., 2006, 18, 2073–2094 CrossRef CAS.
- G. Lota, B. Grzyb, H. Machnikowska, J. Machnikowski and E. Frackowiak, Chem. Phys. Lett., 2005, 404, 53–58 CrossRef CAS.
- H. Guo and Q. Gao, J. Power Sources, 2009, 186, 551–556 CrossRef CAS.
- K. Jurewicz, K. Babeł, A. Źiółkowski and H. Wachowska, Electrochim. Acta, 2003, 48, 1491–1498 CrossRef CAS.
- M. Seredych, D. Hulicova-Jurcakova, G. Q. Lu and T. J. Bandosz, Carbon, 2008, 46, 1475–1488 CrossRef CAS.
- E. Raymundo-Piñero, M. Cadek and F. Béguin, Adv. Funct. Mater., 2009, 19, 1032–1039 CrossRef.
- F. Béguin, K. Szostak, G. Lota and E. Frackowiak, Adv. Mater., 2005, 17, 2380–2384 CrossRef.
- H. M. Jeong, J. W. Lee, W. H. Shin, Y. J. Choi, H. J. Shin, J. K. Kang and J. W. Choi, Nano Lett., 2011, 11, 2472–2477 CrossRef CAS PubMed.
- Y. Chen, X. Zhang, D. Zhang, P. Yu and Y. Ma, Carbon, 2011, 49, 573–580 CrossRef CAS.
- Z. Li, Z. Xu, H. Wang, J. Ding, B. Zahiri, C. M. B. Holt, X. Tan and D. Mitlin, Energy Environ. Sci., 2014, 7, 1708 CAS.
- C.-M. Chen, Q. Zhang, M.-G. Yang, C.-H. Huang, Y.-G. Yang and M.-Z. Wang, Carbon, 2012, 50, 3572–3584 CrossRef CAS.
- Z. Li, Z. Xu, X. Tan, H. Wang, C. M. B. Holt, T. Stephenson, B. C. Olsen and D. Mitlin, Energy Environ. Sci., 2013, 6, 871 CAS.
- C. O. Ania, V. Khomenko, E. Raymundo-Piñero, J. B. Parra and F. Béguin, Adv. Funct. Mater., 2007, 17, 1828–1836 CrossRef CAS.
- S. Biniak, G. Szymański, J. Siedlewski and A. Świ
tkowski, Carbon, 1997, 35, 1799–1810 CrossRef CAS.
- Y. Wang, D. C. Alsmeyer and R. L. McCreery, Chem. Mater., 1990, 2, 557–563 CrossRef CAS.
- J.-H. Kim, J.-S. Kim, Y.-G. Lim, J.-G. Lee and Y.-J. Kim, J. Power Sources, 2011, 196, 10490–10495 CrossRef CAS.
- M. Schroeder, M. Winter, S. Passerini and A. Balducci, J. Power Sources, 2013, 238, 388–394 CrossRef CAS.
- A. Jain, V. Aravindan, S. Jayaraman, P. S. Kumar, R. Balasubramanian, S. Ramakrishna, S. Madhavi and M. P. Srinivasan, Sci. Rep., 2013, 3, 3002 Search PubMed.
- J. Ding, H. Wang, Z. Li, K. Cui, D. Karpuzov, X. Tan, A. Kohandehghan and D. Mitlin, Energy Environ. Sci., 2015, 8, 941–955 CAS.
Footnotes |
† Electronic supplementary information (ESI) available. See DOI: 10.1039/c5ee03149d |
‡ These authors contributed equally to this work. |
|
This journal is © The Royal Society of Chemistry 2016 |