DOI:
10.1039/D1BM01406D
(Paper)
Biomater. Sci., 2022,
10, 216-226
Palladium cubes with Pt shell deposition for localized surface plasmon resonance enhanced photodynamic and photothermal therapy of hypoxic tumors†
Received
8th September 2021
, Accepted 10th November 2021
First published on 13th November 2021
Abstract
Multifunctional phototherapy nanoagents for imaging-guided synergistic photothermal therapy (PTT) and photodynamic therapy (PDT) are highly desirable in the field of solid tumor therapy. Nevertheless, the tumor microenvironment (TME) inherently associated with hypoxia significantly hampers the photodynamic effect of these multifunctional nanoagents. Herein, Pd nanocubes coated with an ultrathin Pt shell were prepared and further conjugated with fluorescein labeled and thiol functionalized polyethylene glycol (FITC-PEG-SH) (denoted as Pd@Pt-PEG). The deposition of a Pt shell on Pd nanocubes not only enhances the photothermal performance, exhibiting excellent hyperthermia outcomes and impressive photothermal (PT) imaging quality, but also leads to the formation of singlet oxygen (1O2) induced by plasmonic excitation. In the meantime, the catalytic activity of the Pt layer is enhanced by electronic coupling and the plasmonic effect, which induces the decomposition of endogenous overexpressed hydrogen peroxide (H2O2) in tumors to generate O2 for conquering TME and augmenting 1O2 generation for efficacious tumor cell apoptosis. The modification of FITC-PEG-SH improves the biocompatibility and provides outstanding fluorescence (FL) imaging properties. Upon NIR laser irradiation, Pd@Pt-PEG allows in situ O2 generation and dual-mode imaging-guided synergistic PTT/PDT that effectively kills hypoxic tumor cells, which makes it a promising nanotherapeutic agent for enhanced tumor therapy.
1. Introduction
Tumor hypoxia is a hallmark of the tumor microenvironment (TME) in nearly all solid tumors, attributed to the high oxygen consumption caused by heterogeneous tumor cell growth and low oxygen supply efficiency caused by dysfunctional vasculature.1–3 Under such hypoxic circumstances undesirable tumor metabolites like hydrogen peroxide (H2O2) are generated, which in turn promote tumor cell mutagenesis and metastasis.1,4–6 Besides, the existence of hypoxia highly limits the treatment efficacy of many oxygen-dependent therapies like chemotherapy and photodynamic therapy (PDT), leading to a poor clinical prognosis.7–9 Given this issue, many intriguing tactics were devised aiming to transport O2 to the tumor region or generate O2in situ to alleviate the hypoxia.3,7–19 Among them, in situ oxygenation through the catalytic decomposition of endogenous H2O2 to O2via catalase or catalase mimics has received growing attention.14–19 Nevertheless, the burden of extra catalase carriers and the instability of catalase mimics may limit their practical application. Accordingly, there is an urgent need for exploring nanomaterials with inherent catalase-like activity for alleviating hypoxia and improving therapeutic efficacy in tumor therapy.
Recently, Pt-based nanostructures have been synthesized as a catalyst with the capability of decomposing H2O2 to generate O2 owing to their extraordinary inherent catalytic properties, high catalytic stability, and remarkable catalytic durability.18,20–22 Current research suggests that the synthesis of Pt nanoshells on metal substrates can increase the catalytic efficiency, which could be attributed to strong surface charge accumulation arising from the compressive strain on Pt shells.23–26 Among various metals, Pd is a good substrate candidate to form a Pd–Pt core–shell nanocomposite on account of their similar lattice constants.23,26–29 Beyond that, in the case of the plasmonic Pd@Pt nanocomposite, the energetic hot electrons triggered by laser irradiation-induced localized surface plasmon resonance of the Pt shell would be transferred to the surface of Pd@Pt cubes increasing the surface electron density, which efficiently enhances the catalytic activity further.30–33 Remarkably, the superimposition of Pt and Pd endowed the nanocomposite with enhanced catalase-like activity and durabilty compared to mere Pt nanoparticles owing to their electronic coupling and plasmonic effect.
In addition, recent studies have shown that light-mediated plasmonic excitation of metal nanoparticles like Au and Pt nanoparticles can sensitize and form singlet oxygen (1O2) from O2, which demonstrates that metal nanoparticles have potential for applications in photodynamic therapy (PDT).34–40 On top of that, the nanostructure based noble metal generally has adequate photothermal stability and photothermal conversion efficiency which is promising for application in photothermal therapy (PTT).41–43 Thus, it is feasible to rationally design a multifunctional Pt-based nanocomposite simultaneously possessing an in situ O2 generation capacity and phototherapeutic functionality (PDT/PTT). In this study, we deposit an ultrathin shell of Pt on Pd nanocubes (denoted as Pd@Pt) through a facile solution-phase method. The deposition of a Pt shell would boost the local electric field leading to greatly enhanced photothermal conversion efficiency. Besides, upon irradiation, the Pt shell would be able to sensitize and form 1O2 efficiently due to light-mediated plasmonic excitation.34,36 Meanwhile, the endogenous H2O2, which is overexpressed in the tumor cells, would be converted into O2 by the catalase-like activity of Pt to efficiently relieve tumor hypoxia and amplify 1O2 generation. As a method to extend the circulation, favor the tumor accumulation, and achieve an outstanding fluorescence imaging property, FITC-PEG-SH was utilized to surface modify the cubes.44,45 Both the in vitro and in vivo experimental results verify that the Pd@Pt-PEG nanoagents possess good biocompatibility and enable a fluorescence and photothermal (FL/PT) imaging-guided synergistic antitumor effect due to the capacity of combining PDT and PTT.
2. Results and discussion
2.1 Material fabrication and characterization
The whole synthesis and therapeutic mechanism of the versatile nanoagents are vividly diagrammed in Scheme 1. First, the Pd cubes with a uniform size of about 16–17 nm were synthesized. As presented in Fig. 1a, the Pd cubes imaged by transmission electron microscopy (TEM) had a uniformsize and cubical morphology, which was confirmed by the corresponding size distribution analysis (Fig. 1b). Then an ultrathin layer of Pt was deposited onto the surface of the Pd nanocubes via a solution-phase method. The average edge size of the synthesized Pd@Pt nanocubes was approximately 18–19 nm, slightly bigger than the initial Pd cubes, which was confirmed by the TEM picture and particle size distribution diagram shown in Fig. 1c and d. Scanning transmission electron microscopy (STEM) and elemental distribution mapping images were obtained to confirm that the core–shell Pd@Pt nanocubes were successfully synthesized. What can be seen in Fig. 1e–h is that the Pd was concentrated in the inner area, while the surrounding Pt was relatively sparse, confirming the successful synthesis of the Pd@Pt cubes. Subsequently, FITC-PEG-SH was used to modify the Pd@Pt cubes to improve the biocompatibility, and tumor accumulation, and to acquire fluorescence imaging capabilities. X-ray photoelectron spectroscopy (XPS) analyses of the Pd cubes, Pd@Pt cubes, and Pd@Pt-PEG were performed to reveal their chemical compositions. It can be seen from Fig. 1i that the oxygen and carbon atomic ratio increased while that of palladium and platinum decreased greatly after conjugation with polyethylene glycol, which provides evidence for a successful modification. The strong peaks for –OH, C
O and C–O in the Fourier-transform infrared spectroscopy (FTIR) spectra (Fig. 1j) also support this conclusion. Besides, the corresponding UV-Vis spectra were measured to assess the optical properties, which exhibit a wide NIR absorption range and verify their potential to be used in PTT (Fig. 1k). The molar extinction coefficient of the Pd@Pt-PEG nanocomposites was estimated to be 2 × 105 M−1 cm−1. Furthermore, an obvious characteristic absorption peak of FITC was observed in Fig. S1,† verifying the successful FITC-PEG-SH conjugation.
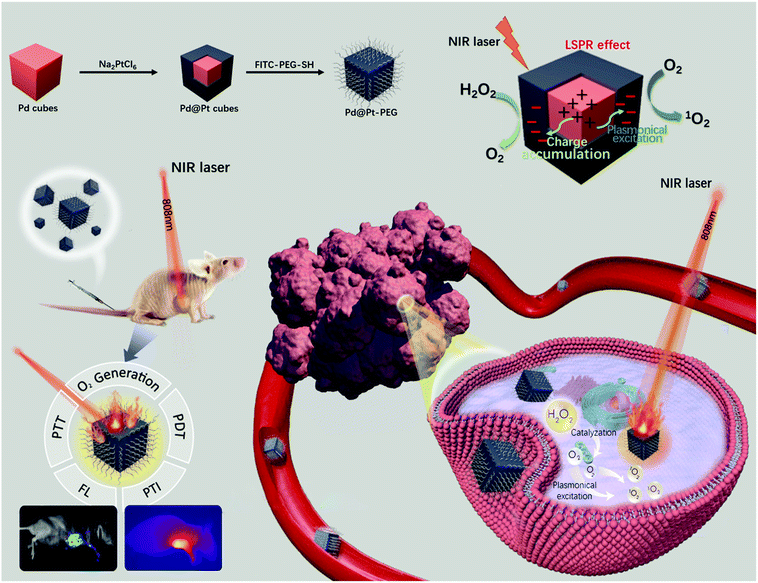 |
| Scheme 1 Schematic diagram of the whole synthesis and therapeutic application of Pd@Pt-PEG. | |
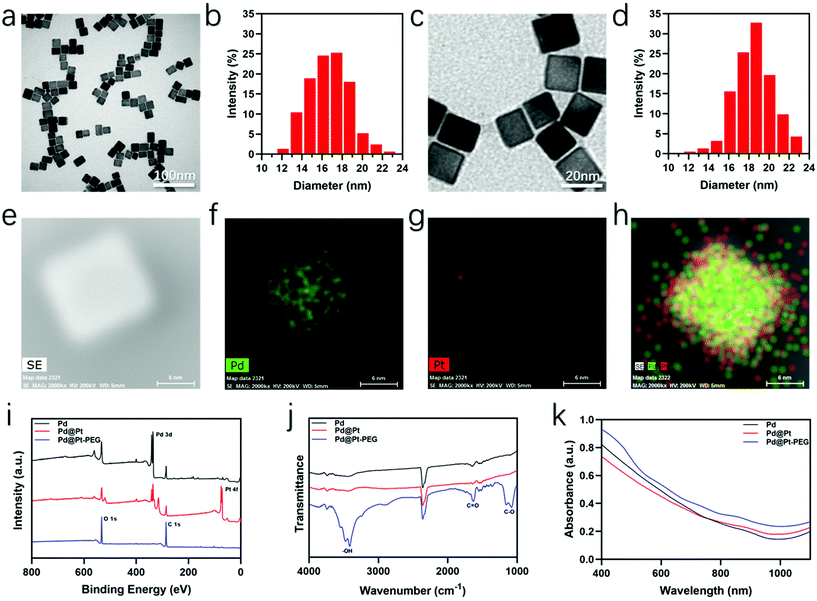 |
| Fig. 1 Characterization. (a) TEM image of the Pd cubes and (b) the corresponding histogram of size distribution. (c) TEM image of the Pd@Pt cubes and (d) the corresponding histogram of size distribution. (e–h) STEM images and corresponding elemental mapping of Pd@Pt cubes. (i) XPS spectra, (j) FTIR spectra, and (k) UV-vis absorbance spectra of Pd cubes, Pd@Pt cubes, and Pd@Pt-PEG. | |
2.2
In vitro photothermal effect, singlet oxygen production and catalytic property detection
The photothermal effect of the nanoagents was systematically assessed by recording their temperature variations. As presented in Fig. 2a–c, the temperature change of Pd@Pt-PEG at various concentrations exhibits concentration and irradiation density dependent characteristics. Intriguingly, the temperature of the Pd@Pt-PEG suspension at a concentration of 200 and 400 μg mL−1 increased above 70 °C in 5 min while the temperature of the water barely changed. Fig. 2d demonstrates that the excellent photothermal stability of the nanoagents gives them considerable potential for PTT. Subsequently, the photothermal conversion efficiency of the Pd@Pt-PEG suspension (200 μg mL−1, 1 mL) was assessed by irradiating with a laser for 5 min. The temperature variation is recorded in Fig. S2† and the corresponding negative natural logarithm of the cooling time is shown in Fig. S3.† According to these results, the photothermal conversion ratio of Pd@Pt-PEG was calculated to be approximately 74.5% and detailed calculation steps are presented in the ESI.† Thus, it can be concluded that the Pd@Pt-PEG nanoagents can be utilized as efficacious PTT agents in tumor phototherapy.
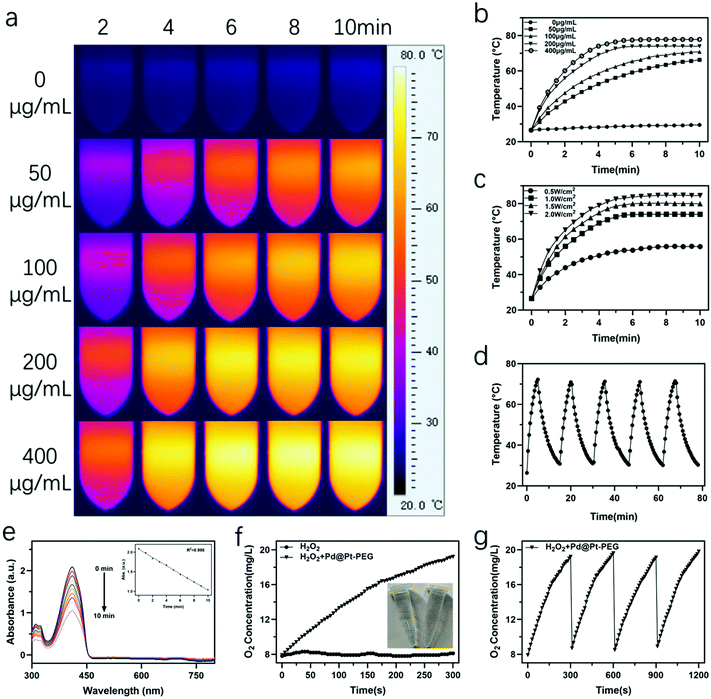 |
| Fig. 2 Photothermal conversion, singlet oxygen production and oxygen generation. (a) The thermographs and (b) corresponding time–temperature curves of the Pd@Pt-PEG dispersions with diverse concentrations irradiated by a laser (808 nm, 1 W cm−2). (c) The time-temperature curves of the Pd@Pt-PEG dispersions (200 μg mL−1) at different laser power densities. (d) Photothermal stability of Pd@Pt-PEG. (e) UV-vis absorbance spectra of the DPBF solution containing Pd@Pt-PEG under laser irradiation for 10 min. The absorbance at 410 nm was recorded per min. Inset: the linear relationship between absorbance at 410 nm versus time. (f) O2 generation after adding Pd@Pt-PEG into H2O2. Inset: digital graph of the H2O2 solution with and without Pd@Pt-PEG. (g) Catalytic durability of Pd@Pt-PEG. | |
Inspired by previous reports34–40 that metal nanoparticles can sensitize and form 1O2 under light excitation, we have also conducted experiments to find whether Pd@Pt-PEG can produce 1O2 under NIR irradiation. 1,3-Diphenylisobenzofuran (DPBF) was utilized for 1O2 detection since the oxidation of DPBF by ROS would decrease the intensity of its characteristic absorbance at 410 nm. The results in Fig. 2e show that the absorption at 410 nm decreased gradually within 10 min, pointing to the conclusion that the Pd@Pt-PEG nanoagents have an efficient 1O2 production ability under NIR irradiation.
The catalytic effect of platinum has been widely reported.18,19 In this study, we employed a JPBJ 608 oxygen probe to evaluate the catalytic activity of Pd@Pt-PEG nanoagents for inducing O2 generation through the decomposition of H2O2. As presented in Fig. 2f, with the addition of Pd@Pt-PEG nanoagents into a hydrogen peroxide solution, the dissolved O2 concentration increased sharply, accompanied by obvious bubble generation. The results significantly indicate the superior catalytic performance of Pd@Pt-PEG nanoagents for the decomposition of H2O2 and the elevation of O2 levels. Moreover, the catalytic capability remained stable during the continuous addition of H2O2 for four cycles (Fig. 2g), confirming outstanding catalytic durability, which is attractive for attenuating tumor hypoxic conditions and simultaneously enhancing therapeutic treatments for hypoxic tumors.
2.3
In vitro cellular experiment
Prior to the biomedical applications of the as-prepared nanoagents, the potential cytotoxicity and cellular internalization were investigated. The CCK-8 kit was utilized to assess the cytotoxicity of the nanoagents. The cell viability data presented in Fig. 3a validated that Pd@Pt-PEG did not exhibit distinct toxicity in LM8 and L929 cells after 24 h of incubation. Afterwards, confocal laser scanning microscopy (CLSM) was carried out for the observation of cellular internalization. As shown in Fig. 3b, the nanoagents were clearly observed surrounding the nucleus and colocalized in the lysosomes at 12 and 24 h, demonstrating efficient internalization. 2′,7′-Dichlorodihydrofluorescein diacetate (DCFH-DA) was employed to prove that Pd@Pt-PEG can produce singlet oxygen in cells. As presented in Fig. 3d, an obvious fluorescence signal can be observed in LM8 cells treated with Pd@Pt-PEG under laser irradiation, while there was no sign of green fluorescence in those groups pretreated with N-acetylcysteine (NAC, a highly efficient ROS scavenger) and other control groups, implying potential application of PDT in vivo. The [(Ru(dpp)3)]Cl2 probe (RDPP) was utilized to verify intracellular O2 generation.46Fig. 3c shows that the red fluorescence faded over time, leading to the conclusion that Pd@Pt-PEG could efficiently catalyze endogenous H2O2 to O2 attenuating tumor hypoxia and enhancing the PDT effect.
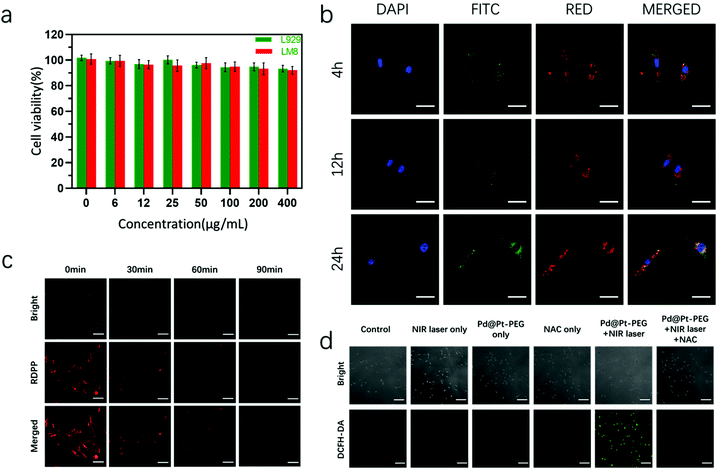 |
| Fig. 3 (a) Cell viabilities of L929 and LM8 cells after incubation with Pd@Pt-PEG at various concentrations for 24 h. (b) CLSM images of LM8 cells after incubation with Pd@Pt-PEG for 4, 12, and 24 h. Scale bars: 20 μm. (c) Intracellular O2 detection at different times (30, 60, and 90 min). Scale bars: 100 μm. (d) Intracellular singlet oxygen production in LM8 cells after different treatments. Scale bars: 200 μm. | |
Motivated by the enhanced hyperthermia effect and the fascinating capability of NIR-induced singlet oxygen production shown above with Pd@Pt-PEG, we further evaluated the in vitro photo-therapeutic efficiency. The effects of PDT or PTT on cell viability were investigated separately. For PDT identification experiments, LM8 cells were irradiated with a laser on ice to reduce the photothermal effects of Pd@Pt-PEG and to induce the PDT effect. As observed from Fig. S4,† in terms of the cell viability of the PDT group, the survival ratio turns out to be about 56.55%. When the LM8 cells were incubated with NAC for 4 h in advance to remove the PDT effect, the result showed a survival rate of approximately 37.53%. Subsequently, when the LM8 cells were cultured with Pd@Pt-PEG and treated with laser irradiation without ice and NAC intervention, the synergistic PDT/PTT effect showed much higher cytotoxicity, killing more than 90% of the tumor cells. For the purpose of intuitively observing the phototherapeutic efficacy, calcein-AM and PI were utilized for live and dead staining. As presented in Fig. 4a, nearly all of the cells in the PTT + PDT group were killed while the majority of the cells in the control group survived, coinciding with the above consequences and sufficiently demonstrating that synergistic PDT/PTT has a superior therapeutic effect compared with other groups. Besides, a similar conclusion can be inferred from the flow cytometric analysis (Fig. 4b). Taken together, the experimental results confirm that the Pd@Pt-PEG agents exhibit excellent biocompatibility and a significant therapeutic effect on the growth inhibition of tumor cells.
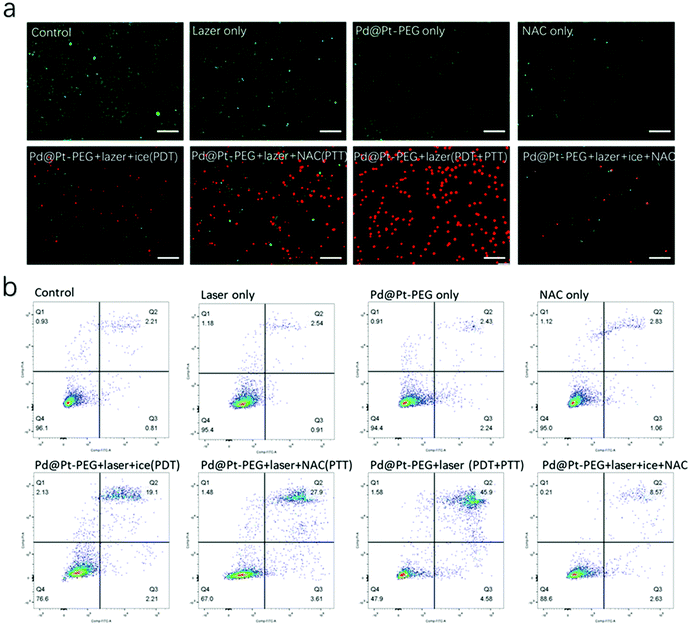 |
| Fig. 4 (a) CLSM images of live and dead cells and (b) flow cytometry analysis of LM8 cells after different treatments. Scale bar: 200 μm. | |
2.4
In vivo imaging performance and antitumor efficiency
Inspired by the superior performance of Pd@Pt-PEG, as shown in the cellular experiment, we proceeded to make use of the nanoagents for in vivo diagnosis and treatment of tumors. The in vivo imaging abilities were first assessed. The modification of FITC-PEG-SH on Pd@Pt provides an outstanding fluorescence imaging property. The fluorescence signal could be seen covering the tumor area at 2 h post-injection (Fig. 5a), which was probably because of the enhanced permeability and retention effect. Then the fluorescence signal intensity in the tumor site increased gradually and reached its peak after 12 h, indicating the effective passive targeted aggregation of the nanoagents into the tumor tissue and an optimum time window for light therapy. Here, the fluorescence signal intensity decreased progressively as time progressed, which indicates the sustained clearance of the nanoagents. Ex vivo fluorescence imaging of tumors and organs and the semiquantitative analysis of the fluorescence signal were also conducted at 24 h. As can be inferred from Fig. 5b and c, an inevitable accumulation in the liver, spleen, and kidney occurred, which may be attributed to the clearance of the monocyte phagocytic system and renal excretion. Meanwhile, an obvious fluorescence signal was still detected in the tumor region. The good fluorescence imaging properties enabled real-time observation of the distribution of Pd@Pt-PEG in vivo and provided a precise imaging-guided tumor therapy.
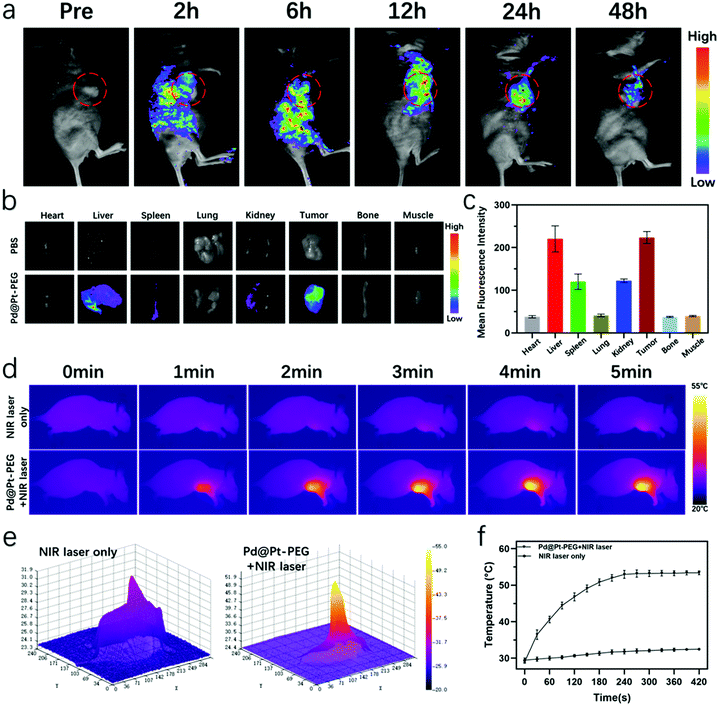 |
| Fig. 5 (a) In vivo fluorescence images of LM8 tumor-bearing mice before and after intravenous administration of Pd@Pt-PEG at different times. (b) Ex vivo fluorescence images and (c) corresponding quantitative analysis of fluorescence intensities of major organs and tumors harvested from mice at 24 h. (d) In vivo thermographs of mice irradiated by a laser (808 nm, 1 W cm−2) with or without intravenous injection of Pd@Pt-PEG. (e) Corresponding 3D thermographs of the tumor regions upon irradiation at the 5th min and (f) corresponding time–temperature curves. | |
In addition, in vivo photothermal images and temperature variations in the tumor area were recorded using an infrared thermal camera (Fotric 225), as shown in Fig. 5d. The photothermal imaging signals in the tumor area of the Pd@Pt-PEG-injected group were enhanced, revealing a remarkable temperature elevation and an extraordinary PT imaging capability of nanoagents at 5 min. The corresponding 3D photothermal images presented in Fig. 5e directly display temperature variations from the center to the periphery of the tumor which provide more evidence for improving tumor diagnostics. As illustrated in Fig. 5f, the tumor temperature of the Pd@Pt-PEG injected group rapidly increased to a mean value of 53.2 °C and remained relatively stable, which was sufficient to ablate the tumor. By contrast, there was only a slight increase in the temperature around the tumor for the NIR laser only group. In summary, these data indicate that Pd@Pt-PEG not only efficiently accumulated inside the tumor but can also be utilized as excellent nanoprobes for PT imaging-guided phototherapy.
To assess the oxygen generation properties of Pd@Pt-PEG in vivo, we further performed animal experiments in LM8 tumor-bearing mice. As illustrated in Fig. S5,† an obvious signal appeared from the generation of oxygen under the catalytic effect of the Pd@Pt-PEG nanoagents for the decomposition of endogenous H2O2. To validate the in vivo phototherapeutic efficacy of Pd@Pt-PEG, the mice were divided randomly into 6 groups (n = 5) and received different treatments in terms of the experimental design. The tumor volume of mice recorded in Fig. 6a indicates that a better anti-tumor effect was clearly observed in the PTT + PDT group in comparison to the single mode PTT group. These results were also verified by the average weight of excised tumors on day 21 (Fig. 6c) and representative tumor digital photos and corresponding H&E staining images (Fig. 6e). As expected, the mice in the PTT/PDT combination therapy group had the best odds of survival and a longer survival time at day 50 (Fig. 6d). Besides, neither a distinct change in the body weight change curves (Fig. 6b), nor noticeable damage in the H&E staining images (Fig. S6†) was observed for all of the treatment groups, indicating negligible toxicity of Pd@Pt-PEG.
 |
| Fig. 6 (a) Relative tumor volumes, (b) body weights, (c) weights of the excised tumors, and (d) survival rate curves of mice of different groups. Data are indicated as mean ± SD. Significant difference at **p < 0.01. (e) Representative graphs and corresponding H&E staining of tumor masses harvested from different groups. Scale bar: 100 μm. | |
2.5 Biodistribution and long-term biotoxicity evaluation
Finally, blood circulation of Pd@Pt-PEG was evaluated. The circulation half-life in blood was roughly 4 hours (Fig. S7†). A relatively long blood circulation time can offer better accumulation of the nanoagents at the tumor regions. To further assess the biodistribution, the Pd contents in major organs were detected using ICP-MS after 24 h of injection. Fig. S8† proves that approximately 16.5% of the nanoparticles were detected to be accumulated in the tumors, which guaranteed their efficient therapeutic performance. Fig. S9† indicates that a substantial amount of nanoparticles was cleared from the mice through feces and urine. Besides, the serum biochemistry and hematology analysis were performed to validate the long-term biotoxicity of Pd@Pt-PEG after intravenous injection at different times. As can be seen from Fig. S10,† the liver function index [alanine aminotransferase (ALT) and aspartate aminotransferase (AST)], the kidney function index [creatinine (Cr) and blood urea nitrogen (BUN)], the myocardial enzyme index [creatinine kinase (CK) and creatinine kinase-MB (CK-MB)] and the hematology parameters [white blood cells (WBCs), red blood cells (RBCs) and mean corpuscular volume (MCV)] all appear to be within the normal range, verifying the nontoxic nature of the nanoagents. Finally, the Pd@Pt-PEG has favorable biocompatibility and promising prospects for application in therapy.
3. Experimental section
3.1. Photothermal performance and stability of Pd@Pt-PEG
To assess the photothermal properties, the prepared suspension was diluted to diverse concentrations (0, 50, 100, 200, and 400 μg mL−1), and then irradiated with an NIR laser for 10 min. The infrared thermal camera was utilized for recording temperature variations. Unless otherwise stated, the NIR laser was used at 1 W cm−2. For photothermal stability measurements, 1 mL of the Pd@Pt-PEG suspension (200 μg mL−1) was exposed to laser irradiation for 5 min and cooled naturally for 10 min for 5 cycles.
3.2. Catalytic property test of Pd@Pt-PEG
The catalytic properties were investigated through measuring the generation of O2 from the decomposition of H2O2 using a portable dissolved oxygen meter at room temperature. Briefly, 0.5 mL of the Pd@Pt-PEG suspension (100 μg mL−1) was added to 10 ml of H2O2 (8.8 mM) and the oxygen level was recorded over time and compared with the H2O2 solution without Pd@Pt-PEG. Meanwhile, the continuous O2 generation with the repetitive addition of H2O2 every 5 min was monitored over time.
3.3. Cytotoxicity assessment
The CCK-8 kit was used to determine the cytotoxicity. The L929 and osteosarcoma LM8 cells (the LM8 and L929 cell lines were obtained from the Cell Bank of the Chinese Academy of Sciences (Shanghai, China)) were seeded and cultured overnight. Subsequently, the old medium was replaced by a new culture medium with diverse concentrations of Pd@Pt-PEG (0, 6, 12, 25, 50, 100, 200, and 400 μg mL−1). A CCK-8 kit was utilized following a standard protocol to assess the cell viability after 24 h.
3.4. Cellular uptake studies
Briefly, LM8 cells were cultured overnight in glass-bottomed dishes (35 mm) to ensure that the cells firmly adhere to the bottom. Then, Pd@Pt-PEG dispersed in the medium (100 μg mL−1) was added into the above dishes and cultured for 4, 12 and 24 h, respectively. Lyso-Tracker Red and DAPI were utilized to stain the lysosomes and nucleus in turn. Finally, the cells were collected and imaged under a CLSM.
3.5. Determination of singlet oxygen production
DPBF was utilized to detect 1O2. Briefly, 2 mL of the Pd@Pt-PEG suspension (100 μg mL−1) was combined with 50 μL of DPBF (1 mg mL−1), then irradiated with a laser (1 W cm−2, 10 min). The UV-visible absorption at 410 nm of DPBF was determined per min. For intracellular 1O2 determination, LM8 cells were incubated in the medium with Pd@Pt-PEG (100 μg mL−1, 4 h), meanwhile LM8 cells precultured with NAC (4 h) were used as a control. Afterwards, the LM8 cells were cultured with DCFH-DA (10 μM, 20 min) and washed three times. Finally, the cells were irradiated with a laser (1 W cm−2, 5 min) and observed under a CLSM.
3.6. Determination of intracellular oxygen production
The intracellular O2 levels were determined utilizing RDPP, whose fluorescence can be well-quenched by the O2 molecule.46 LM8 cells were cultured in a medium with Pd@Pt-PEG (100 μg mL−1) for different times (30, 60, and 90 min) and further cultured in a 10 μM RDPP medium. Then the intracellular RDPP was excited at 488 nm and its fluorescence was observed under a CLSM.
3.7. Cytotoxicity of Pd@Pt-PEG under NIR irradiation
To investigate the photo-therapeutic efficiency, the LM8 cells were cultured with Pd@Pt-PEG in the medium (200 μg mL−1, 4 h). Effects of PDT and PTT with Pd@Pt-PEG on cell viability were investigated separately. For the PDT experiments, the cells were exposed to laser irradiation (1 W cm−2, 10 min) on ice to make sure that the temperature fluctuation of the wells was within 10 °C. For PTT experiments, the cells were precultured with NAC (4 h) followed by irradiation with a laser (10 min). To investigate synergistic PDT and PTT treatments, the cells were irradiated with a laser at room temperature without NAC pretreatment. Finally, a CCK-8 kit was utilized for cell viability determination. For observing the cytotoxicity intuitively, the LM8 cells treated by diverse protocols above were stained with calcein-AM and PI and imaged under a CLSM.
3.8. Flow cytometry apoptosis analysis
Cell apoptosis was evaluated using flow cytometry. The LM8 cells were incubated at a concentration of 3 × 105 cells per well overnight, and subsequently treated with different abovementioned treatments. Finally the cells were collected, washed, stained with annexin V-FITC and PI and analyzed using flow cytometry.
3.9. Animal model establishment
Female Balb/c mice (six-weeks-old) were injected with LM8 cells (2 × 106, 100 μL) subcutaneously to establish a xenograft LM8 tumor model. The mice would be utilized in the following experiments as the tumor size [(length × width2)/2] reached approximately 100 mm3.
3.10.
In vivo FL and PT imaging
The LM8 tumor-bearing mice were injected with 0.1 mL Pd@Pt-PEG (2 mg mL−1) intravenously. FL imaging was achieved using a Night OWL II LB983 at 2, 6, 12, 24 and 48 h post injection. Organs and tumors were harvested 24 h post injection and imaged in the same way. When it comes to in vivo PT imaging experiments, the tumor sites were exposed to laser irradiation (1 W cm−2, 5 min) 12 h post injection and the images were recorded using an infrared thermal camera.
3.11.
In vivo tumor growth inhibition experiments
To investigate the in vivo phototherapy efficiency of the nanoagents after intravenous injection, the LM8 tumor-bearing mice were randomly divided into the following six groups (n = 5): (i) control; (ii) NIR laser irradiation only; (iii) Pd@Pt-PEG treatment only; (iv) NAC only; (v) Pd@Pt-PEG + NIR laser + NAC (PTT group); and (vi) Pd@Pt-PEG + NIR laser irradiation (PTT + PDT group). Pd@Pt-PEG (2 mg mL−1, 0.1 mL) was injected intravenously. The tumor area was exposed to laser irradiation (1 W cm−2, 10 min) at 12 h postinjection. Tumor size and body weight were recorded every three days. Major organs and tumors were harvested for further histological analysis after 21 days of treatment.
3.12. Biodistribution and long-term toxicity assessment
We first examined the blood circulation of the nanoagents. The LM8 tumor-bearing mice were treated with an intravenous injection of Pd@Pt-PEG (n = 5). The blood samples were collected at different times. Inductively coupled plasma mass spectrometry (ICP-MS) was used to examine the concentration of Pd in the blood. Then the biodistribution experiment was performed. After 24 h of intravenous injection of the nanoagents, the tumors and the major organs were harvested, weighed, and homogenized, and the accumulation of Pd was examined via ICP-MS. To assess the long-term toxicity of the particles, healthy mice were treated with intravenous injection of the nanoagents at a single dose of 10 mg kg−1. Serum biochemistry and hematology analysis were carried out at different times (1 day, 1 week, and 1 month). Meanwhile, mice treated with an injection of saline were used as the control.
3.13. Statistical analysis
The data were indicated as mean ± SD. The Student's test and two-way ANOVA were applied to compare differences between groups, *p < 0.05 and **p < 0.01 was defined as statistically significant.
4. Conclusion
In conclusion, versatile nanotherapeutic agents were successfully synthesized by coating an ultrathin shell of Pt on Pd nanocubes followed by conjugation with FITC-PEG-SH. The nanoparticles displayed an outstanding photothermal effect and excellent 1O2 producing capacity upon 808 nm NIR laser irradiation. In addition, benefiting from superior catalytic properties, the nanoparticles can efficiently decompose overexpressed endogenous H2O2 inside TME into O2, which distinctly attenuates the hypoxic environment and simultaneously improves the PDT efficacy. Furthermore, extensive in vivo and in vitro results verified that the nanoparticles can be utilized for FL/PT imaging-guided synergistic PTT/PDT treatment. Thus, our study developed a new versatile nanocomposite using a facile synthesis procedure with high yields, which holds great promise for biomedical applications in the future.
Conflicts of interest
The authors declare no conflict of interest.
Acknowledgements
The authors gratefully acknowledge financial support from the Key University Science Research Project of Jiangsu Province (21KJA350003), Scientific Research Project of Jiangsu Provincial Health and Health Commission (Z2021070), Key research and development projects of Xuzhou Science and Technology Bureau (KC21178) and The General Program of Natural Science Foundation of Jiangsu Province (BK20201154). All animal procedures were performed in accordance with the Guidelines for Care and Use of Laboratory Animals of Xuzhou Medical University and approved by the Ethics Committee at the Xuzhou Medical University.
References
- E. B. Rankin and A. J. Giaccia, Science, 2016, 352, 175–180 CrossRef CAS.
- W. R. Wilson and M. P. Hay, Nat. Rev. Cancer, 2011, 11, 393–410 CrossRef CAS.
- X.-W. Wang, X.-Y. Wang, X.-Y. Zhao, G.-Q. Li, Z.-J. Yang, Y.-H. Gong, Z. Liu and L. Cheng, Appl. Phys. Rev., 2020, 7, 041411 CAS.
- F. Hajizadeh, I. Okoye, M. Esmaily, M. G. Chaleshtari, A. Masjedi, G. Azizi, M. Irandoust, G. Ghalamfarsa and F. Jadidi-Niaragh, Life Sci., 2019, 237, 116952 CrossRef CAS PubMed.
- V. L. Dengler, M. Galbraith and J. M. Espinosa, Rev. Biochem. Mol. Biol., 2014, 49, 1–15 CrossRef CAS.
- Y. Lou, P. C. McDonald, A. Oloumi, S. Chia, C. Ostlund, A. Ahmadi, A. Kyle, U. Keller, S. Leung and D. Huntsman, Cancer Res., 2011, 71, 3364–3376 CrossRef CAS.
- J. Zhen, S. Tian, Q. Liu, C. Zheng, Z. Zhang, Y. Ding, Y. An, Y. Liu and L. Shi, Biomater. Sci., 2019, 7, 2986–2995 RSC.
- H. Wang, Y. Chao, J. Liu, W. Zhu, G. Wang, L. Xu and Z. Liu, Biomaterials, 2018, 181, 310–317 CrossRef CAS.
- X. Li, N. Kwon, T. Guo, Z. Liu and J. Yoon, Angew. Chem., Int. Ed., 2018, 57, 11522–11531 CrossRef CAS.
- A. Sharma, J. F. Arambula, S. Koo, R. Kumar, H. Singh, J. L. Sessler and J. S. Kim, Chem. Soc. Rev., 2019, 48, 771–813 RSC.
- W. Tang, Z. Zhen, M. Wang, H. Wang, Y.-J. Chuang, W. Zhang, G. D. Wang, T. Todd, T. Cowger, H. M. Chen, L. Liu, Z. Li and J. Xie, Adv. Funct. Mater., 2016, 26, 1757–1768 CrossRef CAS.
- K. Wang, Z. Zhang, L. Lin, J. Chen, K. Hao, H. Tian and X. Chen, Chem. Mater., 2019, 31, 3313–3323 CrossRef CAS.
- L.-H. Liu, Y.-H. Zhang, W.-X. Qiu, L. Zhang, F. Gao, B. Li, L. Xu, J.-X. Fan, Z.-H. Li and X.-Z. Zhang, Small, 2017, 13, 1701621 CrossRef.
- Y. Cheng, H. Cheng, C. Jiang, X. Qiu, K. Wang, W. Huan, A. Yuan, J. Wu and Y. Hu, Nat. Commun., 2015, 6, 8785 CrossRef CAS.
- Q. Jia, J. Ge, W. Liu, X. Zheng, S. Chen, Y. Wen, H. Zhang and P. Wang, Adv. Mater., 2018, 30, 1706090 CrossRef PubMed.
- S. Z. F. Phua, G. Yang, W. Q. Lim, A. Verma, H. Chen, T. Thanabalu and Y. Zhao, ACS Nano, 2019, 13, 4742–4751 CrossRef CAS.
- Q. Sun, Z. Wang, B. Liu, T. Jia, C. Wang, D. Yang, F. He, S. Gai and P. Yang, Chem. Eng. J., 2020, 390, 124624 CrossRef CAS.
- S. Liang, X. Deng, Y. Chang, C. Sun, S. Shao, Z. Xie, X. Xiao, P. Ma, H. Zhang, Z. Cheng and J. Lin, Nano Lett., 2019, 19, 4134–4145 CrossRef CAS.
- J. Dang, H. He, D. Chen and L. Yin, Biomater. Sci., 2017, 5, 1500 RSC.
- D. Sun, X. Pang, Y. Cheng, J. Ming, S. Xiang, C. Zhang, P. Lv, C. Chu, X. Chen, G. Liu and N. Zheng, ACS Nano, 2020, 14, 2063–2076 CrossRef CAS PubMed.
- Z. Lu, J. Gao, C. Fang, Y. Zhou, X. Li and G. Han, Adv. Sci., 2020, 7, 2001223 CrossRef CAS PubMed.
- J. Wei, J. Li, D. Sun, Q. Li, J. Ma, X. Chen, X. Zhu and N. Zheng, Adv. Funct. Mater., 2018, 28, 1706310 CrossRef.
- J. Zhang, M. B. Vukmirovic, Y. Xu, M. Mavrikakis and R. R. Adzic, Angew. Chem., Int. Ed., 2005, 44, 2132–2135 CrossRef CAS PubMed.
- K. Sasaki, H. Naohara, Y. Cai, Y. M. Choi, P. Liu, M. B. Vukmirovic, J. X. Wang and R. R. Adzic, Angew. Chem., Int. Ed., 2010, 49, 8602–8607 CrossRef CAS.
- K. D. Gilroy, A. Ruditskiy, H.-C. Peng, D. Qin and Y. Xia, Chem. Rev., 2016, 116, 10414–10472 CrossRef CAS PubMed.
- J. Kim, H. Kim, W. J. Lee, B. Ruqia, H. Baik, H. S. Oh, S. M. Paek, H. K. Lim, C. H. Choi and S. I. Choi, J. Am. Chem. Soc., 2019, 141, 18256–18263 CrossRef CAS.
- J. R. Kitchin, J. K. Nørskov, M. A. Barteau and J. G. Chen, Phys. Rev. Lett., 2004, 93, 156801 CrossRef CAS PubMed.
- X. Wang, Y. Orikasa, Y. Takesue, H. Inoue, M. Nakamura, T. Minato, N. Hoshi and Y. Uchimoto, J. Am. Chem. Soc., 2013, 135, 5938–5941 CrossRef CAS PubMed.
- S. Habas, H. Lee, V. Radmilovic, G. A. Somorjai and P. Yang, Nat. Mater., 2007, 6, 692–697 CrossRef CAS PubMed.
- F. Wang, C. Li, H. Chen, R. Jiang, L. D. Sun, Q. Li, J. Wang, J. C. Yu and C.-H. Yan, J. Am. Chem. Soc., 2013, 135, 5588–5601 CrossRef CAS.
- Z. Fang, Y. Wang, C. Liu, S. Chen, W. Sang, C. Wang and J. Zeng, Small, 2015, 11, 2593–2605 CrossRef CAS PubMed.
- H. Cheng, K. Fuku, Y. Kuwahara, K. Mori and H. Yamashita, J. Mater. Chem. A, 2015, 3, 5244–5258 RSC.
- H. Cheng, T. Kamegawa, K. Mori and H. Yamashita, Angew. Chem., Int. Ed., 2014, 53, 2910–2914 CrossRef CAS PubMed.
- R. Vankayala, A. Sagadevan, P. Vijayaraghavan, C.-L. Kuo and K. C. Hwang, Angew. Chem., Int. Ed., 2011, 50, 10640–10644 CrossRef CAS PubMed.
- R. Vankayala, C.-L. Kuo, A. Sagadevan, P.-H. Chen, C.-S. Chiang and K. C. Hwang, J. Mater. Chem. B, 2013, 1, 4379–4387 RSC.
- Y.-Z. Chen, Z. U. Wang, H. Wang, J. Lu, S.-H. Yu and H.-L. Jiang, J. Am. Chem. Soc., 2017, 139, 2035–2044 CrossRef CAS PubMed.
- L. Gao, R. Liu, F. Gao, Y. Wang, X. Jiang and X. Gao, ACS Nano, 2014, 8, 7260–7271 CrossRef CAS PubMed.
- N. Macia, R. Bresoli-Obach, S. Nonell and B. Heyne, J. Am. Chem. Soc., 2019, 141, 684–692 CrossRef CAS.
- S. J. Chadwick, D. Salah, P. M. Livesey, M. Brust and M. Volk, J. Phys. Chem. C, 2016, 120, 10647–10657 CrossRef.
- W. Zhang, Y. Li, J. Niu and Y. Chen, Langmuir, 2013, 29, 4647–4651 CrossRef CAS PubMed.
- X. Wang, X. Zhong, J. Li, Z. Liu and L. Cheng, Chem. Soc. Rev., 2021, 50, 8669–8742 RSC.
- E. K. Lim, T. Kim, S. Paik, S. Haam, Y. M. Huh and K. Lee, Chem. Rev., 2015, 115, 327–394 CrossRef CAS.
- M. Ali, Y. Wu and M. El-Sayed, J. Phys. Chem. C, 2019, 123, 15375–15393 CrossRef CAS.
- H. Otsuka, Y. Nagasaki and K. Kataoka, Adv. Drug Delivery Rev., 2012, 64, 246–255 CrossRef.
- N. M. Gulati, P. L. Stewart and N. F. Steinmetz, Mol. Pharm., 2018, 15, 2900–2909 CrossRef CAS.
- P. Zhang, J. Guo, Y. Wang and W. Pang, Mater. Lett., 2002, 53, 400–405 CrossRef CAS.
Footnote |
† Electronic supplementary information (ESI) available. See DOI: 10.1039/d1bm01406d |
|
This journal is © The Royal Society of Chemistry 2022 |
Click here to see how this site uses Cookies. View our privacy policy here.