DOI:
10.1039/D1BM01374B
(Paper)
Biomater. Sci., 2022,
10, 243-257
Arsenite-loaded albumin nanoparticles for targeted synergistic chemo-photothermal therapy of HCC†
Received
31st August 2021
, Accepted 30th October 2021
First published on 30th November 2021
Abstract
Arsenic trioxide (ATO, As2O3), an active ingredient of traditional Chinese medicine, has been approved by the U.S. Food and Drug Administration as an effective therapeutic agent for acute promyelocytic leukemia (APL). However, the application of ATO in treating advanced solid tumors like hepatocellular carcinoma (HCC) is still restricted by limited therapeutic efficacy and insufferable side effects. To solve this problem, we reported a general and facile strategy using human serum albumin (HSA) as a template for synthesizing a series of ATO-based nanoparticles with uniform single-albumin size. Then, we prepared a multifunctional drug delivery system (MDDS) based on MnAs/HSA termed MnAs/ICG/HSA-RGD, and tested its efficacy both in vitro and in vivo. Our results revealed that the photothermal effect of MnAs/ICG/HSA-RGD can not only cause irreversible damage to the tumor but also accelerate the discharge of As and Mn2+ ions, enabling responsive chemotherapy and magnetic resonance imaging. Interestingly, the expression of HSP90, vimentin, and MMP-9 in tumor cells was inhibited during the treatment, resulting in less metastasis and recurrence. Moreover, no apparent side effect has been observed during the treatment. Therefore, MnAs/ICG/HSA-RGD can be considered as a promising option for HCC with excellent therapeutic efficacy and minimum side effects.
Introduction
As one of the severe malignancies threatening human health, hepatocellular carcinoma (HCC) occurs frequently and ranks as the second leading cause of tumor-related mortality worldwide.1,2 Patients with early to intermediate-stages can be treated with locoregional therapies such as resection, ablation, and interventional therapy.3,4 Unfortunately, HCC is a kind of cancer with genetic heterogeneity and therapy resistance. Thus, many patients with HCC ultimately enter the advanced stage, at which point only intervention, chemotherapy and targeted therapy can be used for the purpose of delaying the natural progression of this disease.5,6 On the other hand, poor tumor accumulation and rapid renal clearance of chemotherapeutic drugs could lead to significant drug resistance and substantial therapy failure.7,8 For this reason, patient outcomes have only improved modestly and incrementally, and their overall 5-year survival rate has shown little progress over the last decade.9,10 Therefore, new therapeutic strategies against HCC remain an unmet medical need.
Photothermal therapy (PTT) has received increasing attention over several decades. Compared with traditional therapy, PTT has been regarded as a convenient stimuli-responsive method, which is noninvasive and highly efficient, and causes minimal damage to normal tissues due to the use of a near-infrared (NIR) laser.11–13 Agent-mediated PTT makes use of photothermal transduction agents (PTAs) by converting the energy of a laser into heat and precisely ablates tumor tissues under the guidance of NIR fluorescence. An ideal PTA should have excellent biocompatibility, high photothermal conversion efficiency, and sufficient accumulation in tumor tissues.14–16 Indocyanine green (ICG) is a widely used NIR dye for fluorescence imaging in clinics, such as in liver surgery and ophthalmic angiography. Therefore, its use has been reported in PTT for tumors, and some improvements in treatment outcomes have been observed.17,18 However, the limited penetration of light and insufficient accumulation of PTAs in tumors lead to incomplete ablation and result in tumor recurrence outside the range of irradiation.19 Many efforts have been dedicated to address these problems. Alternatively, a combination of laparoscopy-assisted PTT and chemotherapy may provide another promising approach.20,21
Arsenic trioxide (ATO) is a generally accepted agent for single or combination therapy of acute promyelocytic leukemia (APL), and many preliminary studies against solid tumors have been carried out.22–27 However, as a single agent, ATO demonstrates limited effectiveness against HCC.28 Its clinical application is significantly restricted by either considerable toxicity at a relatively high dose (0.5–2.0 mg per kg body weight), leading to liver failure, peripheral neuropathies, and cardiac toxicity, or poor bioavailability to solid tumors.29,30 Nanoparticle-based delivery systems have attracted particular attention for delivering ATO owing to their capacities to increase drug accumulation in tumor tissues and lower drug exposure of normal tissues.31,32 To date, various drug delivery systems based on nanomaterials, such as liposomes,33 mesoporous silica nanoparticles,25,34,35 and polymeric vesicles,36,37 have been actively studied to improve the therapeutic index of ATO to solid tumors. Among these drug delivery systems, the albumin-based delivery system is considered as a promising candidate for delivering ATO,38–41 especially after human serum albumin (HSA)-paclitaxel (Abraxane®) has been proven effective as a clinical agent of non-small cell lung cancer and breast cancer.42–44 It is encouraging that a smart HSA-ATO nanodrug has been demonstrated to be promising for treating chronic myeloid leukemia (CML) recently.45 Though ATO loaded bovine serum albumin (BSA) nanoparticles (NPs) have been reported,46 their relatively large size (600–800 nm) restricts their further biomedical applications. Therefore, it is meaningful and interesting to construct arsenic loaded ultrasmall HSA nanodrugs with improved pharmacokinetic profiles. More intriguingly, the incorporation of PTT into this ATO nanodrug-based chemotherapy has potential synergistic benefits, such as boosting drug release at the tumor site, which is critical to increasing anticancer efficacy and minimizing side effects.
Herein, we report a facile biomineralization method to synthesize a pH-responsive ATO and ICG co-loaded ultrasmall NP within a single HSA molecule as a template, resulting in a multifunctional theranostic NP, MnAs/ICG/HSA-RGD, which enables HCC-targeted dual-modal imaging and photothermal-enhanced chemotherapy. After intravenous injection, these NPs could selectively accumulate in tumors. Upon exposure to the mildly acidic tumor microenvironment, MnAs/ICG/HSA-RGD NPs collapse to discharge arsenite and Mn2+ ions for killing cancer cells and enabling T1-weighted magnetic resonance imaging (MRI), respectively.25,34 Under the guidance of real-time monitoring with MRI and fluorescence imaging permitted by ICG, NIR laser irradiation could be performed with precision, which leads to better efficiency in eliminating cancer cells with the photothermal effect. Besides, the heat generated by the photothermal effect would also greatly accelerate the release of arsenite and Mn2+ ions, boosting the efficacy of the arsenite-based chemotherapy. Furthermore, arsenite could inhibit the overexpression of some critical proteins that help cancer cells recover from heat damage and promote cell migration and invasion, such as HSP90, vimentin, and MMP-9, thus enhancing the efficiency of PTT. Collectively, our nanodrug achieves effective synergistic chemo-photothermal therapy with real-time monitoring using MRI and fluorescence dual-modal imaging, and is able to accomplish tumor ablation and prevent potential tumor metastasis and recurrence. This is the first attempt of combinational chemo-photothermal therapy with single-albumin-based nanodrugs for HCC treatment.
Experimental section
Methods
Arsenic trioxide (As2O3, ATO), manganese acetate, indocyanine green (ICG), manganese acetate (90%), zinc acetate (90%), nickel acetate (90%), gadolinium acetate, tetrahydrate ferric chloride, N-hydroxysuccinimide (NHS), 1-ethyl-3-(3-dimethylaminopropyl)carbodiimide hydrochloride, N,N-diisopropylethylamine (DIPEA), and human serum albumin (HSA) were purchased from Sigma-Aldrich (St Louis, MO). Ammonium hydroxide was purchased from Sinopharm Chemical Reagent Co. Ltd (Shanghai, China). t-Boc-N-amido-PEG3-acid, bis(2,5-dioxopyrrolidin-1-yl) succinate, and amine-terminated cRGD were purchased from Sangon Biotech (Shanghai) Co., Ltd. Annexin V-FITC and propidium iodide (PI) were purchased from Thermo Fisher Scientific (Waltham, MA, USA). Dulbecco's phosphate-buffered saline (D-PBS, pH 7.4), fetal bovine serum (FBS), Roswell Park Memorial Institute 1640 (RPMI 1640), Dulbecco's modified Eagle's medium (DMEM), and 0.05% trypsin-EDTA were purchased from Gibco Life Technologies (AG, Switzerland). All chemicals were used as received without further purification. Ultrapure water was produced using a Millipore water purifier.
Characterization
Transmission electron microscopy (TEM) images were captured on a JEM-2100 microscope (Hitachi, Tokyo, Japan) at an accelerating voltage of 200 kV. Ultraviolet visible (UV-vis) and fluorescence spectra were acquired on a Hitachi U-3900 ultraviolet visible spectrophotometer and a Hitachi F-7000 fluorophotometer, respectively. Element analysis of Fe, Mn, Zn, Ni, Gd, and As was carried out with inductively coupled plasma atomic emission spectroscopy (ICP-AES) or inductively coupled plasma mass spectroscopy (ICP-MS). MRI and T1 relaxation time measurements were performed on a 0.5 T NMI20 Analyzing and Imaging System (Niumag Corporation, Suzhou, China). An 808 nm laser was generated by a laser transmitter from Hi-Tech Optoelectronics Co., Ltd. Thermal images were taken using a Testo 885-1 Thermal Imager (Testo, Inc., Germany). Confocal fluorescence microscopy images were taken on an Axio Observer (Carl Zeiss AG, Germany). In vivo MRI was performed on a 7 T Varian micro MRI System (Varian Medical Systems, Inc., USA). In vivo fluorescence images were captured using an IVIS Lumina II in vivo Imaging System (PerkinElmer, Inc., USA).
Preparation of RGD-labeled HSA
Synthesis of cRGD-PEG3-NH2.
t-Boc-N-amido-PEG3-acid (27.7 mg, 0.10 mmol), EDC·HCl (23.0 mg, 0.12 mmol), and NHS (13.8 mg, 0.12 mmol) were added to anhydrous DMF (1 mL), and the resulting solution was stirred at room temperature for 1 h, to which amine-terminated cRGD (72.4 mg, 0.12 mmol) in anhydrous DMF (1.0 mL) was added. The resulting mixture was stirred for 18 h at room temperature and concentrated. The crude product was re-dissolved in dichloromethane (DCM, 3.5 mL). Trifluoroacetic acid (TFA, 1.5 mL) was added dropwise, and the resulting solution was stirred for another 30 min. Purification with an ion-pairing reversed-phase high-performance liquid chromatography (RP-HPLC) system using an RPC18 column (250 × 4.6 mm, 5 micrometers) and gradient elution (Eluant A: water containing 0.05% TFA; Eluant B: acetonitrile containing 0.05% TFA) and lyophilization gave cRGD-PEG3-NH2 in 65% yield (52.4 mg). ESI-MS (m/z): calcd for [M + H]+ 807.4, found 807.8.
Synthesis of cRGD-PEG3-acid NHS ester.
To a solution of cRGD-PEG3-NH2 (40.3 mg, 0.05 mmol) and DIPEA (ca. 0.005 mol) in anhydrous DMF (2 mL) in an ice bath, a solution of bis(2,5-dioxopyrrolidin-1-yl) succinate (93.7 mg, 0.3 mmol) in DMF (2 mL) was slowly added. The resulting solution was stirred at room temperature overnight and concentrated. Purification with HPLC (water with 0.1% TFA/CH3CN with 0.1% TFA) and subsequent lyophilization gave cRGD-PEG3-acid NHS ester in 55% yield (27.6 mg). ESI-MS (m/z): calcd for [M + H]+ 1004.5, found 1004.9.
Preparation of cRGD-labeled HSA.
cRGD-PEG3-acid NHS ester (10 mg, 0.01 mmol) in 1 mL carbonate/bicarbonate buffer (0.1 M, pH = 9) was added to a stirred solution of HSA in DMF (1 mL, 25 mg mL−1). The resulting solution was stirred for 6 h at room temperature. Dialysis (MWCO 5 kDa) and subsequent lyophilization gave cRGD-labeled HSA as a white powder stored at 4 °C for further use.
Synthesis of MAs/HSA and MnAs/ICG/HSA-RGD
Typically, 10 mg of HSA in 25 mL of deionized water was prepared, and 1 mL of 50 mM metal chloride solution was added into the HSA solution. The resulting mixture was vigorously stirred for 2 h at 37 °C, to which 1 mL of 100 mM NaAsO2 aqueous solution was added. 5 min later, 1 mL of 1 M NaOH solution was added. The resulting mixture was vigorously stirred at 37 °C for 8 h. Unreacted HSA and inorganic ions were removed by centrifugation at 800g for 30 min to give the corresponding MAs/HSA.
MnAs/HAS-RGD was prepared similarly except that cRGD-labeled HSA was used instead of HSA. To 10 mL of 20 mg mL−1 MnAs/HSA-RGD aqueous solution was added 1 mL of 0.5 M ICG DMSO solution. The resulting solution was stirred for 8 h in the dark. Purification via dialysis against deionized water gave MnAs/ICG/HSA-RGD. The loading capacities (LCs) and encapsulation efficiency (EEs) of ICG, As and Mn ions were calculated according to the following equations (X represents ICG, As, or Mn):
Evaluation of drug release
To profile the release of metal ions and arsenite from various formulations of this nanoplatform, 3 mL (20 mg mL−1) of various arsenite-loaded albumin NPs (FeAs/HSA, NiAs/HSA, ZnAs/HSA, GdAs/HSA, MnAs/HSA, MnAs/ICG/HSA, and MnAs/ICG/HSA-RGD) were loaded into dialysis bags (MWCO = 10 kDa), respectively. Then the dialysis bags were left in 800 mL of 1× PBS buffer (pH 7.4) or citrate buffer (pH 6.0) at 37 °C with gentle stirring, respectively. At different time points, 1 mL of each bulk solution was collected and digested for analyzing the released amount of metal ions and arsenite by ICP-MS. The experiments were performed in triplicate for each formula. The release profiles of MnAs/ICG/HSA-RGD with 808 nm laser irradiation at 37 °C in different buffers were also acquired. The experiments were carried out similarly except for additional 808 nm laser irradiation for 5 min every hour.
To profile the release of ICG, 3 mL of ICG/HSA or MnAs/ICG/HSA-RGD (8 mg mL−1 ICG) in a dialysis bag (MWCO = 5 kDa) was left in 100 mL of PBS (pH 7.4) or PBS containing 10% FBS. 0.5 mL of each bulk solution was collected at different time points and subjected to UV-vis-NIR spectroscopy for evaluating the released ICG.
Cell culture
HeLa, A549, SMMC-7721, and Huh7 cells were purchased from the Cell Bank of Type Culture Collection of the Chinese Academy of Sciences (Shanghai, China). SMMC-7721 cells were cultured in Roswell Park Memorial Institute 1640 (RPMI-1640) supplemented with 10% v/v fetal bovine serum (FBS). HeLa, Huh-7, and A549 cells were cultured in Dulbecco's Modified Eagle's Medium (DMEM) supplemented with 10% v/v FBS. All cells were incubated at 37 °C under an atmosphere containing 5% CO2.
Cellular uptake of different nano-formulations
1 × 107 SMMC-7721 cells were incubated with MnAs/ICG/HSA or MnAs/ICG/HSA-RGD (with As concentration equivalent to 1 μM ATO) for different periods of time (0, 1, 2, and 3 h). The cells were washed with 1× PBS three times and collected into a 200 μL centrifuge tube for T1-weighted MRI with a 0.5 T NMI20 Analyzing and Imaging System. T1-Weighted MRI was performed with a multi-echo fast spin-echo sequence and the parameters were as follows: TR/TE = 100/12 ms, 256 matrices, and thickness = 1 mm, NS = 16. Then the collected cells were digested with 1 mL of 10% nitric acid for analyzing the uptake amount of As and Mn by ICP-MS. All experiments were carried out in triplicate.
Cytotoxicity assays
Cytotoxicities of various formulations were evaluated via MTT assays. Firstly, cells were seeded into a 96-well plate at a density of 5 × 103 cells per well in culture media and incubated under an atmosphere containing 5% CO2 at 37 °C for 12 h. Then, the cells were treated with various nanoformulations (FeAs/HSA, NiAs/HSA, ZnAs/HSA, GdAs/HSA, MnAs/HSA, MnAs/ICG/HSA, and MnAs/ICG/HSA-RGD) in culture media at different concentrations for 48 h. The experiments were carried out in quintuplicate for each formulation at each concentration. Then the media were replaced with DMEM or RPMI-1640 containing 0.5 mg mL−1 of MTT and the cells were incubated for another 4 h at 37 °C. After the media were discarded, 100 μL of DMSO was added to each well. The absorbance at 490 nm of each well was measured using a MultiSkan FC microplate reader (Thermo Scientific) immediately. Cell viabilities and IC50 were calculated accordingly.
Evaluation of photothermal effects on cells
Firstly, 5 × 104 SMMC-7721 cells were collected and seeded into a 24-well plate. After being cultured for 24 h, the cells were incubated with free ICG, HSA/ICG, MnAs/ICG/HSA, and MnAs/ICG/HSA-RGD in serum-free media at different ICG concentrations for 2 h, respectively, and irradiated with an 808 nm laser at 1 W cm−2 for 10 min. Then, the media were replaced with fresh culture media, and the cells were further incubated for another 24 h. Finally, cell viabilities were assessed with MTT assays as described in the previous section.
For fluorescence microscopy, SMMC-7721 cells seeded in 3.5 cm dishes were separated into eight groups (n = 3), which were treated with free ICG, ICG/HSA, MnAs/ICG/HSA, MnAs/ICG/HSA-RGD, laser irradiation only, ICG/HSA and irradiation, MnAs/ICG/HSA and laser irradiation, and MnAs/ICG/HSA-RGD and laser irradiation, respectively. The cells in each group were incubated with the drugs for 2 h with the ICG concentration fixed at 5 mg L−1. For the groups treated with a laser, cells were irradiated with an 808 nm laser for 5 min. After various treatments, they were co-stained with propidium iodide (PI) and calcein acetoxymethyl ester (calcein-AM). Confocal laser fluorescence microscopy (CLSM) images were captured with an Axio Observer.
Cell migration and invasion
For cell migration assays, about 50
000 SMMC-7721 cells were seeded into upper chambers with serum-free media containing various drugs. Culture media were added to lower chambers. Incubated for 24 h, the upper chamber cells were gently wiped out with cotton swaps, while the migrated cells were stained with crystal violet. Then the pictures of the chambers were taken using a Zeiss digital camera, and the number of migrating cells from 5 randomly selected fields was counted. For cell migration assays, the protocols were similar except that the bottom of the inserts (upper chambers) was precoated with Matrigel.
Animal ethics
All animal experiments in this research were conducted according to the protocols approved by the Ethical Guidelines on animal Care with an approval from the animal welfare committee of the Fifth Affiliated Hospital of Sun Yat-sen University (2018103001).
Establishment of flank tumors
To inoculate SMMC-7721 flank tumors, 5 × 106 cells were injected into the hock of the right leg. Tumors were generally palpable within one week, and tumor volumes were calculated with the equation volume = (length × width2)/2. When the tumors reached 30 mm3, the mice were randomized into different groups so that the difference in mean tumor size and standard deviation among groups was minimized.
Haematoxylin and eosin (H&E) and immunohistochemistry (IHC) assay
All main organs and tumors were collected and fixed with 4% formalin and paraffin. Then, they were cut into 5 μm sections and mounted on glass slides. Optical images were captured with a light microscope after H&E staining. For immunohistochemical studies, the sections of tumor specimens were prepared using the same method. After routine deparaffinization, rehydration, and antigen retrieval, the sections were incubated with primary antibodies overnight under a humidified atmosphere at 4 °C. The slides were stained with a secondary antibody for 30 min after washing three times. Subsequently, all these sections were treated with liquid DAB substrates and counterstained with hematoxylin.
In vivo biodistribution of nanoformulations
All mice were divided into three groups (n = 5) and intravenously injected with free ATO, MnAs/ICG/HSA, and MnAs/ICG/HSA-RGD at a dose equivalent to 1 mg As per kg body weight, respectively. Then, the mice were sacrificed 24 h later, and the samples of the main organs were collected (20 mg each). After chemical digestion, the concentration of As was detected by ICP-MS, which was converted to the percentage of injection dose (%ID) per gram of organ.
In vivo MRI
When subcutaneous SMMC-7721 tumors reached approximately 200 mm3, the mice were randomly separated into two groups (n = 5) and injected with MnAs/ICG/HSA and MnAs/ICG/HSA-RGD at a dose equivalent to 1.5 mg Mn per kg body weight, respectively. Coronal and transverse plane images were acquired using an MRI scanner (7.0 T) at 0, 1, 2, 4, and 6 h after injection with a multi-slice gradient-echo pulse sequence and the following parameters: TR/TE = 500/7.56 ms, 256 × 256 matrices, thickness = 1 mm, average = 4, and FOV = 50 × 50. Signal-to-noise ratios (SNRs) of the regions of interest (ROIs) were evaluated with ImageJ software. The efficiency of contrast enhancement was assessed with SNRpost/SNRpre.
In vivo fluorescence imaging
When subcutaneous SMMC-7721 tumors of nude mice reached 200 mm3, the mice were randomly separated into three groups and injected with HSA/ICG, MnAs/ICG/HSA, and MnAs/ICG/HSA-RGD at a dose equivalent to 5 mg ICG per kg body weight. Real-time NIR images were collected at selected time points after injection (0, 2, 4, 8, 24, and 36 h) with an IVIS Luminal imaging system. At the end of research, these mice were sacrificed after 36 h. Subsequently, the main organs and tumors were harvested for fluorescence imaging in the same system. Fluorescence intensities of ROIs were measured, and semi-quantitative analysis was conducted with Living Imaging software.
In vivo combinational therapy
When flank SMMC-7721 tumors reached about 100 mm3 in volume, the mice were randomized into eight groups (n = 5) in a way that the difference in mean tumor size and body weight among groups was minimized. The injection treatment was performed on days 2, 4, and 6 respectively, and laser irradiation was carried out on day 6 after the third injection finished for 2 h. The treatments were as follows: Group I, intravenous injection with PBS (0.1 M, 200 μL); Group II, intravenous injection with PBS (0.1 M, 200 μL) and irradiation with an 808 nm laser (1 W cm−2, 10 min) at 2 h post injection (p.i.); Group III, intravenous injection with free ATO (200 μL, 1 mg As per kg body weight); Group IV, intravenous injection with ICG/HSA (200 μL, 5 mg ICG per kg body weight) and treated with an 808 nm laser (1 W cm−2, 10 min) at 2 h p.i.; Group V, intravenous injection with MnAs/ICG/HSA (200 μL, 1 mg As per kg body weight, 5 mg ICG per kg body weight); Group VI, intravenous injection with MnAs/ICG/HSA (200 μL, 1 mg As per kg body weight, 5 mg ICG per kg body weight) and irradiation with an 808 nm laser (1 W cm−2, 10 min) at 2 h p.i.; Group VII, intravenous injection with MnAs/ICG/HAS-RGD (200 μL, 1 mg As per kg body weight, 5 mg ICG per kg body weight); Group VIII, intravenous injection with MnAs/ICG/HAS-RGD (200 μL, 1 mg As per kg body weight, 5 mg ICG per kg body weight) and irradiation with an 808 nm laser at 2 h p.i. Tumor volumes and body weights of mice were under continuous monitoring during the experiment. According to a consensus for flank tumor studies, the mice whose tumor volumes were over 1000 mm3 were regarded as dead by the end of treatment. The mice were sacrificed at the end of the experiment. Tumors and the main organs including the heart, liver, spleen, lungs, and kidneys were collected for H&E staining and IHC staining. Also, blood samples were collected for biochemical analysis.
Statistics
Two-way ANOVA and Turkey's multiple comparison tests were used. *p < 0.05, **p < 0.01, ***p < 0.001, and ****p < 0.0001. Quantitative data in this research are presented as mean ± standard error of the mean (SEM).
Results and discussion
Synthesis and characterization
We first assessed the efficacy of arsenite-loaded albumin NPs as a delivery nanoplatform for ATO. As shown in Scheme 1, the synthesis of arsenite-loaded albumin NPs is similar to the biomineralization process in nature, which has been well demonstrated as a feasible strategy in previous reports.47–50 Utilizing this approach, we synthesized multiple arsenite-loaded albumin NPs including FeAs/HSA, MnAs/HSA, NiAs/HSA, ZnAs/HSA, and GdAs/HSA. Transmission electron microscopy (TEM) images showed that the NPs were uniform and well-disperse with a diameter around 5 nm (Fig. 1a, Fig. S1–S5†). Gel filtration chromatography (GFC) profiles confirmed that the size of these NPs in 1× PBS were similar to that of HSA (Fig. 1b and Fig. S6a, ESI†), suggesting that each nanoparticle was formed by a single albumin macromolecule. Moreover, no obvious change in HD was observed after 24 h incubation 1× PBS containing 10% FBS (Fig. S6b†), indicating their excellent stability. Digital images revealed that these NPs were well dispersed in water and a significant Tyndall phenomenon could be clearly observed (Fig. S7†). We also tried to synthesize metal arsenite (MAs) NPs in the absence of HSA, which showed poor stability and precipitated after 24 h standing (Fig. S8a†). Unsurprisingly, these nanoparticles were found amorphous with TEM (Fig. S8b†), indicating that the introduction of HSA highly improved the stability and morphology of MAs NPs. We then profiled the releases of As (mostly in the form of arsenite) and metal (mostly in the form of metal ions) from MAs/HSA NPs in various environments (pH 7.4 and 6.0) via inductively coupled plasma mass spectrometry (ICP-MS). There were about 11% and 15% of As and Mn released from MnAs/HSA NPs incubated in pH 7.4 PBS for 24 h, as shown in Fig. 1c, while up to 80% and 83% of As and Mn were released when the environmental pH was adjusted to 6.0, which is similar to the pH value of the tumor microenvironment. Similar behaviors were also observed with other MAs/HSA NPs, though the release rates and efficiencies were different among various NPs (Fig. S9†). These results suggested that these MAs/HSA NPs were relatively stable in the physiological environment (pH 7.4) and susceptible to acidic environments, which was critical to pH-responsive release. We further evaluated the cytotoxicity of these nanoparticles against liver cancer cell lines. As shown in Fig. 1d, Fig. S10, and S11,† MAs/HSA NPs exhibited comparable cytotoxicity to free ATO against SMMC-7721 and Huh7 cells, which indicated that this HSA-based drug delivery system could effectively deliver ATO to liver cancer cells. Then, we chose MnAs/HSA NPs for further studies because of their potential activatable capability in contrast enhancement for MRI.
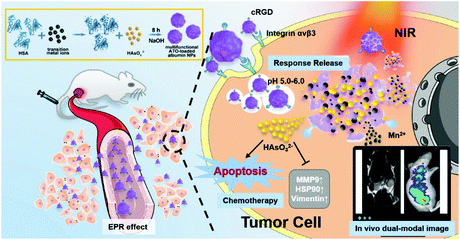 |
| Scheme 1 Schematic illustration of monodisperse arsenite-loaded nanoparticles synthesized with a single HSA as a template and their application as an efficient synergistic chemo-photothermal therapy agent for HCC under the guidance of targeted MRI/NIR dual-modal imaging in vivo. | |
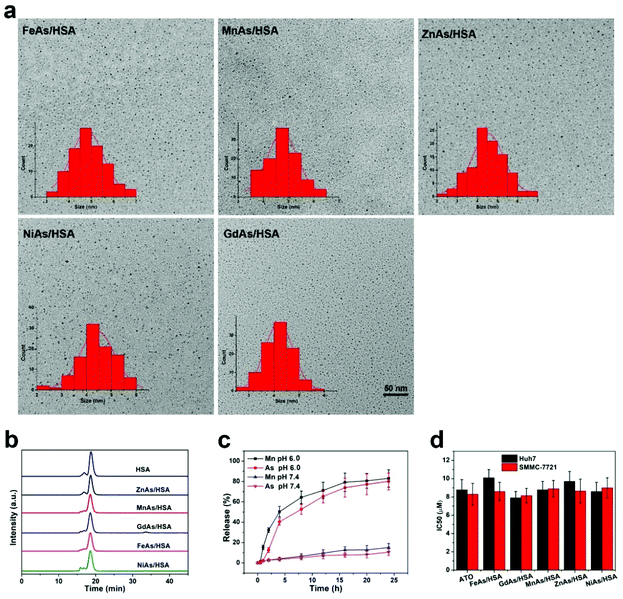 |
| Fig. 1 Characterization of MAs/HSA NPs. (a) TEM images and the corresponding size distributions of various ultrasmall arsenite-loaded albumin NPs. (b) Gel filtration chromatography (GFC) profiles of HSA and various arsenite-loaded single-albumin NPs. (c) Release profiles of MnAs/HSA at pH values 7.4 and 6.0. n = 3 per group. Please see the ESI† for release profiles of other MAs/HSA NPs. (d) Half maximal inhibitory concentrations (IC50) of ATO and various MAs/HSA NPs against SMMC-7721 cells and Huh7 cells for 48 h. n = 5 per group. | |
Preparation and characterization of MnAs/ICG/HSA-RGD
We tried to incorporate a targeting moiety, cRGD, and a photothermal therapy agent, ICG, to MnAs/HSA NPs to make MnAs/ICG/HSA-RGD. Firstly, we synthesized cRGD-labeled HSA. We used PEG3 as a linker to facilitate the exposure of cRGD on the surface of MnAs/HSA NPs. The identities of the intermediates were measured by electrospray ionization mass spectrometry (ESI-MS) (Fig. S12†). According to the analysis of matrix-assisted laser desorption/ionization time-of-flight (MALDI-TOF) mass spectrometry (Fig. S13†), about two to three cRGD molecules were conjugated to one HSA molecule on average. We then synthesized MnAs/HSA-RGD NPs according to Scheme 1. After purification, ICG molecules were loaded into MnAs/HSA-RGD through hydrophobic interactions. The as-prepared MnAs/ICG/HSA-RGD NPs were well dispersed and very stable in PBS, PBS containing 10% fetal bovine serum (FBS), and cell culture media (Fig. 2a). A representative TEM image reveals that MnAs/ICG/HSA-RGD NPs were monodisperse with a spheroidal shape and an average diameter of 5.0 ± 1.5 nm (Fig. 2b). Meanwhile, their average HD was 13.5 ± 2.3 nm as confirmed by dynamic light scattering (DLS), slightly larger than the diameter in the TEM image, which was probably due to the existence of a hydration shell formed in the aqueous solution (Fig. 2c). The X-ray photoelectron spectroscopy (XPS) of MnAs/HSA-RGD NPs showed that the peaks of As 3d5/2 and As 3d3/2 were around 44.3 eV and 48.5 eV, while the peaks of Mn 2p3/2 and Mn 2p1/2 were around 641.4 eV and 653.1 eV, respectively (Fig. S14†). As a result, the loading capacity (LC) of As in NPs measured by ICP-MS was about 1.3% and the encapsulation efficiency (EE) was 31.3%, and the molar ratio of Mn/As was 1.55
:
1.
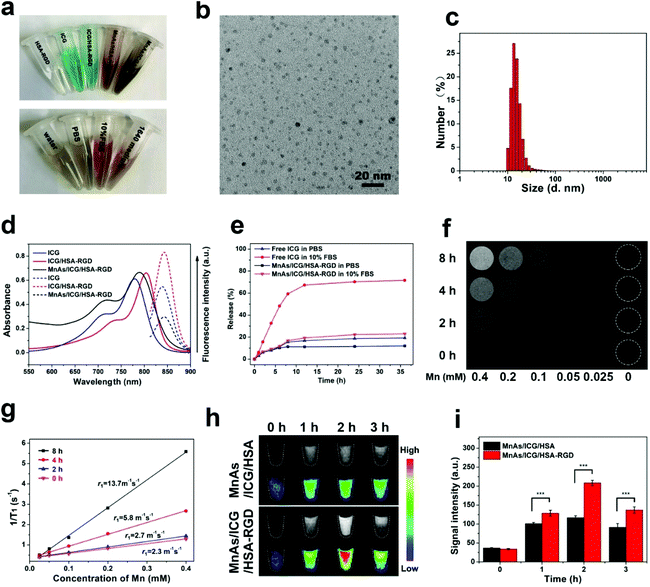 |
| Fig. 2 Synthesis and characterization of MnAs/ICG/HSA-RGD. (a) Top: Optical images of MnAs/ICG/HSA-RGD, ICG/HSA-RGD, MnAs/HSA, HSA, and free ICG in deionized water. Bottom: Optical images of MnAs/ICG/HSA-RGD in different solutions and media for 24 h. (b) A representative TEM image of MnAs/ICG/HSA-RGD. (c) Hydrodynamic diameter (HD) distribution of MnAs/ICG/HSA-RGD in deionized water, analyzed via dynamic light scattering (DLS). (d) UV-Vis-NIR absorbance spectra (solid lines) and fluorescence emission spectra (dotted lines, excitation: 790 nm) of MnAs/ICG/HSA-RGD, ICG/HSA-RGD, and free ICG. (e) Release profiles of ICG from MnAs/ICG/HSA-RGD under different conditions. Free ICG solution was used as a control. (f) T1-Weighted MR imaging of MnAs/ICG/HSA-RGD in PBS buffer (pH 6.0) incubated for different periods of time. (g) Relaxivity (r1) of MnAs/ICG/HSA-RGD in 1× MES buffer (pH 6.0) incubated for different periods of time. (h) T1-Weighted MR grey scale images and the corresponding pseudo color images of cells treated with MnAs/ICG/HSA and MnAs/ICG/HSA-RGD for indicated periods of time. (i) Quantitative analysis of signal intensities in (h). ***p < 0.001. | |
The calibration curves for HSA and ICG were acquired with UV-Vis spectrometry (Fig. S15†). The absorbance peak of MnAs/ICG/HSA-RGD was slightly red-shifted compared with those of free ICG and ICG/HSA-RGD. Moreover, stronger fluorescence emission was observed for ICG/HSA-RGD than for MnAs/ICG/HSA-RGD, which could be ascribed to the MnAs shell (Fig. 2d). These results indicate that ICG was successfully encapsulated in MnAs/ICG/HSA-RGD NPs, and the average LC and EE of ICG was determined to be 6.7% and 71%, respectively.
Then we assessed the stability of MnAs/ICG/HSA-RGD NPs by dialyzing ICG and MnAs/ICG/HSA-RGD solutions against PBS and 10% FBS solution. As shown in Fig. 2e, less than 20% of ICG was released from MnAs/ICG/HSA-RGD NPs during dialysis against both PBS and PBS containing 10% FBS for 35 h. In comparison, more than 35% and 70% of ICG was released from ICG solution when dialyzed against PBS and PBS containing 10% FBS for 35 h, respectively. Meanwhile, we found that the ICG loaded in MnAs/ICG/HSA-RGD NPs was more photo-stable than free ICG since the absorbance of free ICG at 808 nm was reduced by 78% after 30 min irradiation with the 808 nm laser at the power of 1.0 W cm−2, while the absorbance of ICG loaded in MnAs/ICG/HSA-RGD NPs was reduced by 49% under the same conditions (Fig. S16†). These results revealed that ICG in MnAs/ICG/HSA-RGD NPs was significantly stabilized, which was largely due to the strong hydrophobic interactions between ICG and HSA and the protection from the MnAs coating shell of these nanoparticles.
Activatable T1-weighted MRI
To evaluate the pH-responsive MR imaging capability of NPs, we incubated MnAs/ICG/HSA-RGD in PBS buffers with different pH values (6.0 and 7.4) and conducted MR imaging at different time points. The signal of MnAs/ICG/HSA-RGD in pH 6.0 PBS gradually increased over time (Fig. 2f), and r1 rose from 2.3 mM−1 s−1 to 13.7 mM−1 s−1 with the release of Mn2+ (Fig. 2g). In contrast, no distinct signal change of MnAs/ICG/HSA-RGD NPs was observed after incubation in pH 7.4 buffer (Fig. S17a†), and exhibited their stability in a neutral environment. We further assessed the pH-responsive contrast enhancement of MnAs/ICG/HSA-RGD for MRI in SMMC-7721 cells. As shown in Fig. 2h, the T1-weighted MRI signals gradually increased, reached a maximum at 2 h and then decreased. It is worth noting that MnAs/ICG/HSA-RGD exhibited significantly higher signals than MnAs/ICG/HSA due to the introduction of the RGD (Fig. 2h and i), which was also confirmed by the result of cellular uptake assessment of ICP-MS (Fig. S17b and c) and flow cytometry (Fig. S18†) of tumor cells. Interestingly, the changes in the intracellular concentrations of Mn and As showed a similar trend to those of MRI signals. These results demonstrated that MnAs/ICG/HSA-RGD enabled contrast-enhanced T1-weighted MRI for monitoring the drug release process, and the incorporation of the RGD group significantly improved the cellular uptake of tumor.
Anti-cancer activity in cells
We evaluated the cytotoxicity of MnAs/ICG/HSA-RGD NPs against a common liver cancer cell line, SMMC-7721, via flow cytometry and MTT assays. As shown in Fig. 3a, after co-incubation with MnAs/ICG/HSA-RGD for 24 h, about 51.3% of SMMC-7721 cells underwent cell apoptosis to various degrees (12.7% early apoptosis and 38.6% cell death). In contrast, only 37.2% and 37.3% of tumor cells treated with ATO and MnAs/ICG/HSA showed the sign for apoptosis, respectively. Consistent with the results of flow cytometry, MnAs/ICG/HSA-RGD NPs displayed significantly higher cytotoxicity (IC50: 3.2 ± 0.3 μM with respect to As) against SMMC-7721 than ATO and MnAs/HSA (IC50: 8.2 ± 0.7 and 8.3 ± 0.6 μM, respectively) (Fig. 3b and c). These results for cytotoxic experiments on A549 cells (Fig. S139 and S19b†) and HeLa cells (Fig. S19c and S19d†) were similar, indicating that MnAs/ICG/HSA-RGD NPs have no apparent heterogeneity for killing cancer cells. Since Mn ions and ICG showed little cytotoxicity and excellent biocompatibility in a range of concentrations (Fig. 3d), the cytotoxicity of MnAs/ICG/HSA-RGD NPs could be ascribed to tumor targeting and the accumulation of arsenite.
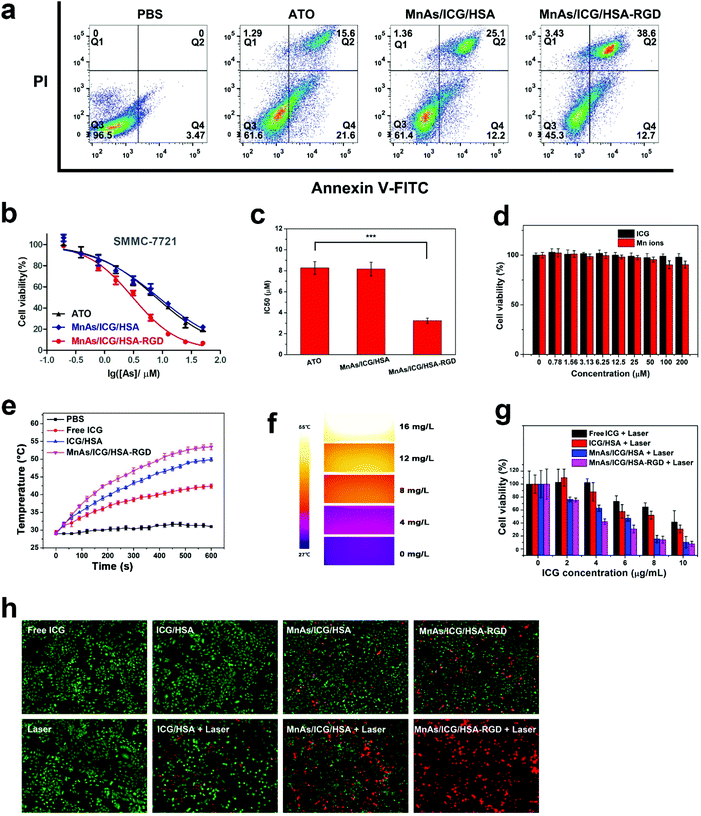 |
| Fig. 3 Anticancer activity of arsenite-loaded single-albumin NPs. (a) Evaluation of SMMC-7721 cell apoptosis and necrosis induced by free ATO, MnAs/ICG/HSA, or MnAs/ICG/HSA-RGD via flow cytometry. PBS was used as a control. (b) Cytotoxicity of free ATO, MnAs/ICG/HSA, and MnAs/ICG/HSA-RGD against SMMC-7721 cells. (c) IC50 (with respect to As) of free ATO, MnAs/ICG/HSA, and MnAs/ICG/HSA-RGD against SMMC-7721 cells which were calculated from (b). ***p < 0.001. (d) Relative cell viability of SMMC-7721 cells treated with different concentrations of ICG and Mn for 48 h, respectively. (e) Temperature changes of 1× PBS (1 mL, pH 7.4) containing ICG, ICG/HSA-RGD, and MnAs/ICG/HSA-RGD irradiated with an 808 nm laser (1 W cm−2 for 10 min). Blank 1× PBS was used as a control. ICG concentration is 8 mg L−1 for all solutions except for blank 1× PBS. (f) NIR thermal images of different MnAs/ICG/HSA-RGD solutions (concentration with respect to ICG) after laser irradiation (1 W cm−2 for 10 min). (g) Cytotoxicities of free ICG, ICG/HSA, MnAs/ICG/HSA, and MnAs/ICG/HSA-RGD against SMMC-7721 cells with laser irradiation. SMMC-7721 cells were incubated with different formulas for 4 h and irradiated with an 808 nm laser at 1 W cm−2 for 10 min. (h) Confocal images of SMMC-7721 cells after various treatments as indicated. Green fluorescent cells: live cells stained with calcein AM. Red fluorescent cells: dead cells stained with PI. | |
We then investigated the photothermal effects of MnAs/ICG/HSA-RGD. As shown in Fig. 3e, the temperature elevation of MnAs/ICG/HSA-RGD solution with near-infrared (NIR) irradiation was much higher than those of PBS, ICG, and ICG/HSA solutions under the same conditions, with the ΔTMAX of MnAs/ICG/HSA-RGD being 24.5 °C and the ΔTMAX of PBS being 3.67 °C, respectively. The photothermal effects of MnAs/ICG/HSA-RGD were also found to be concentration-dependent (Fig. S20†), which was consistent with the NIR images captured using an infrared thermal camera (Fig. 3f). These results revealed the outstanding efficiency of MnAs/ICG/HSA-RGD for photothermal capacity. Encouraged by the promising results, we investigated the efficiency of MnAs/ICG/HSA-RGD-based combinational therapy. The results of cytotoxicity assessment suggested that MnAs/ICG/HSA-RGD-based combinational therapy showed considerable cytotoxicity against SMMC-7721 cells (Fig. 3g). Furthermore, SMMC-7721 cells were treated with different drugs, irradiated with a laser, co-stained with propidium iodide (PI) and calcein AM, and subjected to laser scanning microscopy (LSM). Consistently, fluorescence images reveal that there were much more red fluorescence in the image of cells treated with MnAs/ICG/HSA-RGD than in the image of SMMC-7721 cells treated otherwise (Fig. 3h). Interestingly, chemotherapy without laser irradiation did not cause severe cell damage after a short period of treatment (Fig. 3h, MnAs/ICG/HSA-RGD), which is consistent with an apparent demarcation between MnAs/ICG/HSA-RGD-treated cells exposed (red) and unexposed (green) to a laser (Fig. S21†), indicating that PTT could significantly induce tumor cell death and accelerate drug release within a short time. This could probably be attributed to the sustained high temperature during photothermal therapy that caused irreversible cell damage and accelerated drug release (Fig. S22†). We also noticed that PTT alone did not lead to severe cell damage, either (Fig. 3h, ICG/HSA + laser), suggesting a strong synergistic effect between chemotherapy and photothermal therapy in this combinational therapy. These exciting results demonstrated the outstanding anti-cancer efficacy of MnAs/ICG/HSA-RGD with laser irradiation and encouraged us further to explore the synergistic mechanism behind this combinational therapy.
We then moved on to the investigation of the synergistic effect of the MnAs/ICG/HSA-RGD-based combinational therapy. It has been reported that HCC cells enduring sublethal PTT would become more aggressive in biological behavior.51,52 To verify this observation, we subcutaneously injected tumor cells with or without preheating in BALB/c nude mice and monitored the growth of the tumors. The results indicated that preheated HCC cells proliferated significantly faster than untreated HCC cells (Fig. 4a). Immunohistochemistry (IHC) analysis reveals that the expressions of heat shock protein 90 (HSP90), mesenchymal proteins vimentin, and matrix metalloproteinase 9 (MMP-9), which were closely related to metastasis and invasion,51,53,54 were upregulated in the tumor tissues inoculated with preheated SMMC-7721 cells (Fig. 4b). Western blotting (WB) analysis confirmed the upregulations of these proteins in preheated SMMC-7721 cells, which could be inhibited by the treatment of MnAs/ICG/HSA-RGD (Fig. 4c and d). Consistent with these results, cell migration and invasion assays suggested that the overexpressions of these proteins in preheated SMMC-7721 cells resulted in considerably enhanced cell metastasis and invasion while the downregulation of these proteins induced by MnAs/ICG/HSA-RGD substantially reduced the degrees of cell metastasis and invasion (Fig. 4e, f, and Fig. S23†). These results underscored the significant antitumor effect of PTT and chemotherapy in the combinational therapy using MnAs/ICG/HSA-RGD.
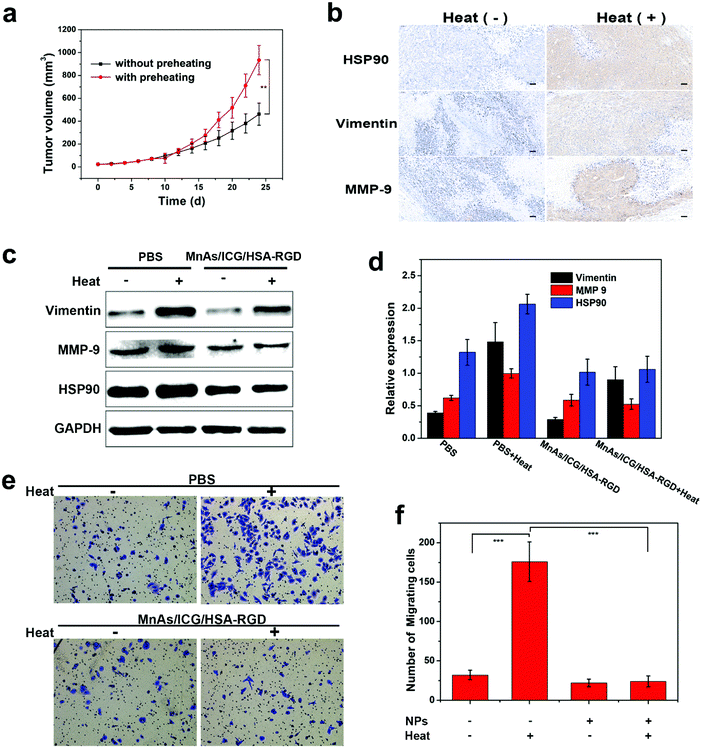 |
| Fig. 4 (a) Tumor volume changes of BALB/c nude mice inoculated with SMMC-7721 cells that were with or without preheating (in a 45 °C water bath for 10 min). **p < 0.01. (b) IHC images of tumor tissues collected from the mice in (a). Scale bars: 50 μm. (c) Western blotting of indicated proteins from SMMC-7721 cells after different treatments. Heating was conducted in a 45 °C water bath for 10 min. (d) Quantitative analysis on the results in (c). (e) Cell invasion assays. SMMC-7721 cells were treated with MnAs/ICG/HSA-RGD NPs or 1× PBS. Preheating was conducted in a 45 °C water bath for 10 min. Cells were stained with crystal violet. (f) Quantitative analysis of (e). ***p < 0.001. | |
In vivo imaging and tumor treatment
Motivated by the potency of MnAs/ICG/HSA-RGD NPs in cells, we moved on to animal experiments with BALB/c nude mice. We firstly evaluated the pharmacokinetics and relative biodistribution of various formulas in subcutaneous SMMC-7721 tumor-bearing mice. Compared to the relatively short circulation half-life of free ATO (0.85 h), the blood half-lives of MnAs/ICG/HSA and MnAs/ICG/HSA-RGD were extended to 2.56 h and 2.27 h, respectively (Fig. 5a), which significantly improved bioavailability, promoted tumor uptake by passive targeting, and facilitated sustained drug release. Biodistribution analysis revealed that the average accumulation of As in the tumors of the mice in the MnAs/ICG/HSA-RGD treated group was 1.7 times higher than that of the mice treated with MnAs/ICG/HSA, and 5.2 times higher than free ATO treated mice, which might ascribe to the enhanced permeability and retention (EPR) effect and the RGD targeting capability (Fig. 5b). These results revealed the excellent tumor-targeting capacity of MnAs/ICG/HSA-RGD resulting from the prolonged blood circulation and the incorporation of tumor-targeting RGD peptides.
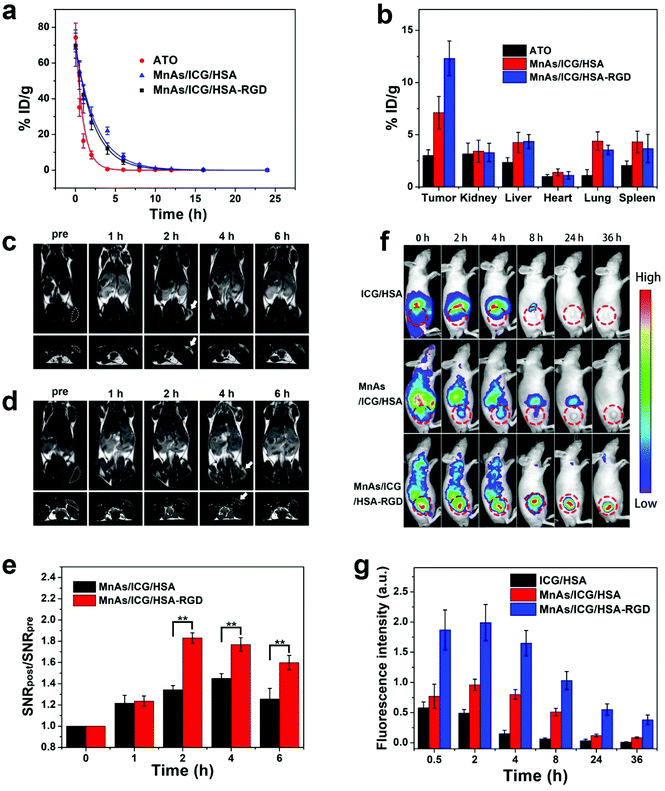 |
| Fig. 5 (a) Blood circulation curves of free ATO, MnAs/ICG/HSA, and MnAs/ICG/HSA-RGD in BALB/c nude mice. (b) Biodistribution of free ATO, MnAs/ICG/HSA, and MnAs/ICG/HSA-RGD in tumor tissues and different organs of SMMC-7721-bearing BALB/c nude mice at 24 h after intravenous injection. Representative MR images of SMMC-7721 tumor-bearing BALB/c nude mice injected with MnAs/ICG/HSA-RGD (c) and MnAs/ICG/HSA (d) acquired at different time points post injection on a MRI scanner (7.0 T). Tumor regions are indicated with white arrows. (e) Quantitative analysis of the relative SNRs of tumor regions in the MR images of the model mice subjected to different treatments as indicated. **p < 0.01. (f) Representative fluorescence images of tumor-bearing mice injected with ICG/HSA, MnAs/ICG/HSA, and MnAs/ICG/HSA-RGD for different times, respectively. (g) Semi-quantitative analysis on the fluorescence intensities of tumor regions in the fluorescence images of SMMC-7721-bearing mice subjected to different treatments as indicated. | |
As we previously reported, manganese NPs could be used for activatable T1-weighted MRI as Mn2+ ions are an excellent T1 contrast agent.34,55 We therefore evaluated the in vivo imaging performance of MnAs/ICG/HSA-RGD. These results indicated that for the mice injected with MnAs/ICG/HSA-RGD, strong T1 signals could be detected in the tumor region within 2 h (Fig. 5c). In contrast, relatively low T1 signals of the tumor region were detected when injected with MnAs/ICG/HSA (Fig. 5d). Signal-to-noise ratios (SNRs) of the tumor regions were carefully calculated and relative SNRs (SNRpost/SNRpre) were used for quantitative analysis (Fig. 5e). The relative SNR of MnAs/ICG/HSA-RGD treated mice was significantly higher than that of the MnAs/ICG/HSA group at various time points, reaching 1.95 at 2 h post-injection and decreasing to 1.8 and 1.5 at 4 h and 6 h post-injection, respectively. These results exhibited a feasibility of MnAs/ICG/HSA-RGD for activatable MR imaging.
Due to the presence of ICG, MnAs/ICG/HSA-RGD could also be activated by the NIR laser. We assessed the imaging performance of MnAs/ICG/HSA-RGD for fluorescence imaging (Fig. 5f). For the mice injected with ICG/HSA, fluorescence signals built up in the liver region and decreased rapidly over time without noticeable tumor accumulation. For the mice injected with MnAs/ICG/HSA-RGD or MnAs/ICG/HSA, considerable fluorescence appeared in the tumor. However, stronger signals in the tumor and weaker signals in the liver were observed in the MnAs/ICG/HSA-RGD injected mice, indicating a better tumor targeting of MnAs/ICG/HSA-RGD. Semiquantitative analysis on the NIR fluorescence images indicated that the fluorescence in the tumor of the mice injected with MnAs/ICG/HSA-RGD peaked at 2 h after injection, which was much higher than those of the mice injected with MnAs/ICG/HSA or ICG/HSA (Fig. 5g). Notably, strong signals in the tumor region of the mice injected with MnAs/ICG/HSA-RGD could still be observed even at 36 h after injection, suggesting an excellent accumulation and retention of MnAs/ICG/HSA-RGD in the tumor (Fig. S24†). Consistent with MRI, these results demonstrate great potential of multimodal imaging with MnAs/ICG/HSA-RGD for improved spatial resolution and precise tumor localization.
Encouraged by the outstanding imaging performance, we further assessed the therapeutic performance of MnAs/ICG/HSA-RGD NPs in vivo. The tumor-bearing nude mice were randomly allocated into eight groups (n = 5 per group): Group I was intravenously injected with 1× PBS; the mice in Group II were injected with 1× PBS and irradiated with a laser; the mice in Group III were treated with ATO (in 100 μL 1× PBS); the mice in Group IV were intravenously injected with ICG/HSA and irradiated with a laser; Group V was intravenously injected with MnAs/ICG/HSA; the mice in Group VI were intravenously injected with MnAs/ICG/HSA and irradiated with a laser; the mice in Group VII were intravenously injected with MnAs/ICG/HSA-RGD; the mice in Group VIII were intravenously injected with MnAs/ICG/HSA-RGD and irradiated with a laser. The dosage of the formulas containing ICG was 5 mg per kg (body weight) and the dosage of the remaining formulas was adjusted accordingly to match the injection volume or the As dosage. During the PTT treatment, the NIR images and temperature of the tumor regions in the groups subjected to laser irradiation were recorded using an infrared thermal camera (Fig. 6a, Fig. S25†). Due to the excellent targeting capacity and outstanding photothermal conversion capacity of MnAs/ICG/HSA-RGD NPs, a significant temperature increase was observed in Group VIII (up to 49.5 °C) with laser irradiation, and the duration of the temperature above 45 °C lasted for over 5 min, which could lead to grievous damage to cancer cells. Meanwhile, the tumors in Groups IV and VI showed a modest temperature increment (up to 40.1 °C and 43.0 °C), respectively. Not surprisingly, no noticeable change in temperature was observed in Group II. It is noteworthy that after laser irradiation, the tumors in Group VIII underwent local burns and the tumors were eliminated by the day (about 18 d later) when the wound was healed (Fig. S26†).
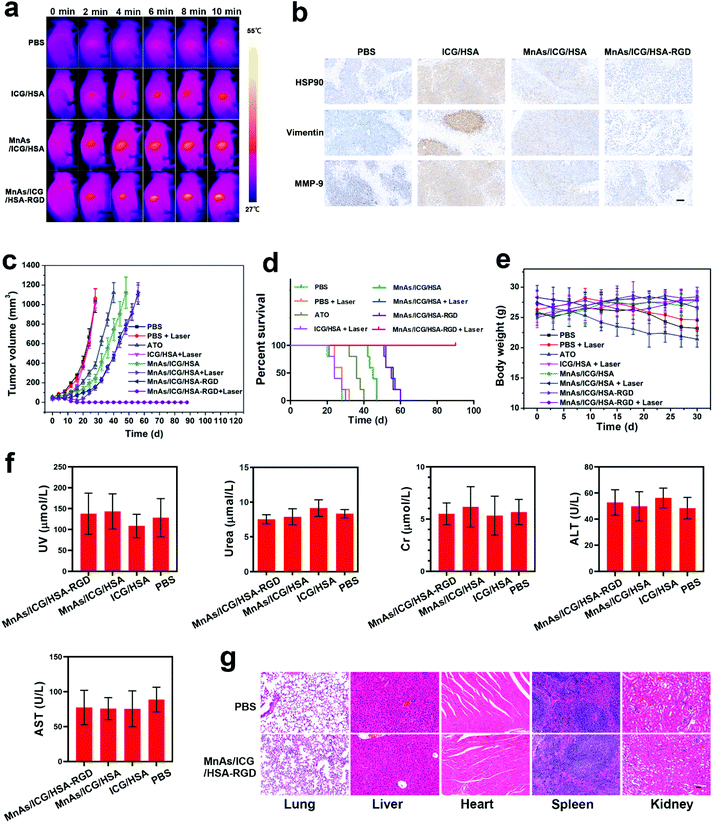 |
| Fig. 6 The combinational cancer therapy in vivo. (a) Photothermal images of mice bearing SMMC-7721 tumors, which were treated with various nano-formulations and irradiated with an 808 nm laser at 1 W cm−2 for 10 min. (b) Immunohistochemistry (IHC) images of tumor tissues collected from mice from different groups, which were all subjected to laser irradiation. Scale bar: 100 μm. (c) Tumor volumes of mice from different groups. (d) Survival curves of mice from different groups (n = 5). (e) Body weight records of mice during the treatment from each group. (f) Biochemistry indexes of mice from different groups after treatment (n = 5 per group). (g) H&E staining of the main organs collected in Group VIII (mice treated with MnAs/ICG/HSA-RGD) and the control group (treated with PBS). Scale bars: 200 μm. | |
At 2 d after different treatments, the mice in Groups II, IV, VI, and VIII were randomly chosen for IHC assays. As shown in Fig. 6b, HSP 90, MMP-9, and vimentin were all upregulated in the tumors of the mice in Group IV due to the heating, while the upregulation of these proteins was significantly suppressed in Groups VI and VIII, in which the mice were treated with ATO containing NPs. Since the upregulation of these proteins promotes tumor metathesis and invasion, the inhibition of the overexpression of these proteins prevents the potential aggravated tumor growth due to sublethal PTT, indicating a promising synergistic effect between chemotherapy and PTT. The synergistic effect was further confirmed by monitoring tumor growth during the treatment (Fig. 6c). ATO or ATO containing HSA-based NPs (Groups III, V, VI, VII, and VIII) clearly inhibited tumor growth to varying degrees. In contrast, the tumors in Group I, II, and IV grew rapidly, resulting in a median survival time of less than 35 d (Fig. 6d). Compared to the results of the chemotherapy-only groups (Groups V and VII), the results of the combinational therapy groups (Groups VI and VIII) showed considerably enhanced inhibition of tumor growth and prolonged survival times. Notably, as shown in an image of a representative mouse in Group VIII, the tumor was completely ablated on day 18 after treatment (Fig. S26†). All mice in this group lived longer than 60 d. These results reveal the excellent efficacy of MnAs/ICG/HSA-RGD NPs for combinational cancer therapy.
It is noteworthy that the dosage of As in this study was much lower than those previously reported,34,45,56 which significantly reduced the side effects. According to the body weight curves, the average body weights of the mice in Groups V, VI, VII, and VIII remained almost the same during the treatment while that of the mice in Group III declined significantly, indicating the superior biocompatibility of HSA-based NPs (Fig. 6e). Besides, compared with the PBS treatment group, no appreciable lesions were observed in the Hematoxylin and Eosin (H&E) of organs collected from the mice treated with MnAs/ICG/HSA-RGD (Fig. 6f). Consistent with the H&E results, the biochemistry indexes, including uric acid (UA), urea, creatinine (Cr), aspartate transaminase (ALT), and alanine aminotransferase (AST), of the experimental mice were at normal levels as the control groups, suggesting that no apparent damage of kidney and liver functions happened (Fig. 6g). These results demonstrate the minimized adverse effects of MnAs/ICG/HSA-RGD NPs for combinational therapy.
Conclusion
In this research, we have developed a general and facile method of preparing a biocompatible HSA-based ultrasmall drug-delivery system within a single HSA as a template for biomedical applications. One of these nanoparticles, MnAs/ICG/HSA-RGD could achieve reliable tumor targeting delivery, sensitive pH-responsive release, and excellent synergistic chemo-photothermal therapy for HCC under the guidance of targeted MRI/NIR dual-modal imaging. We further explored the synergistic mechanism of MnAs/ICG/HSA-RGD. On the one hand, PTT in the MnAs/ICG/HSA-RGD-based combinational therapy is able to accelerate the release of chemotherapeutics apart from causing irreversible damage to tumor tissues via the exertion of high temperature. On the other hand, in vitro experiments revealed that ATO in the nano-system significantly suppressed the growth of liver tumor cells and inhibited cell metastasis and invasion. In this way, MnAs/ICG/HSA-RGD could inhibit in vivo tumor growth more effectively and greatly prolong the survival time of experimental animals. Therefore, tumors were eliminated after the combinational therapy without recurrence, and no significant side effect has been observed in the HCC tumor-bearing mice, as demonstrated by in vitro and in vivo experiments. We believe that this new combinational therapy provides a promising means for treating HCC with high therapeutic efficacy and reduced adverse effects, which is also inspiring for further development of effective HCC therapies.
Author contributions
Ke Zhang: Methodology, data curation, writing-original draft preparation. Dan Li: Conceptualization, investigation. Bin Zhou: Methodology, software. Jiani Liu: Visualization, investigation. Xiangjie Luo: Software, validation. Ruixue Wei: Formal analysis, Lizhu Wang: Formal analysis. Xiaojun Hu: Resources. Zhongzhen Su: Conceptualization, visualization. Hongyu Lin: Project administration, writing-reviewing and editing. Jinhao Gao: Conceptualization, writing-reviewing and editing. Hong Shan: Supervision, writing–review & editing, funding acquisition.
Conflicts of interest
The authors declare that they have no known competing financial interests or personal relationships that could have appeared to influence the work reported in this paper.
Acknowledgements
This work was supported by the National Key R&D Program of China (2018YFC0910600), the National Natural Science Foundation of China (81620108017, 81771879), the China Postdoctoral Science Foundation (2019M663274), the Guangdong Basic and Applied basic Research Foundation (2019A1515011311), and the Fundamental Research Funds for the Central Universities, Sun Yat-sen University (2021qntd35).
References
- F. Bray, J. Ferlay, I. Soerjomataram, R. L. Siegel, L. A. Torre and A. Jemal, CA Cancer J. Clin., 2018, 68, 394–424 CrossRef.
- K. A. McGlynn, J. L. Petrick and H. B. El-Serag, Hepatology, 2020, 73(0270-9139), 4–13 Search PubMed.
- A. Villanueva, N. Engl. J. Med., 2019, 380, 1450–1462 CrossRef CAS.
- L. A. Torre, F. Bray, R. L. Siegel, J. Ferlay, J. Lortet-Tieulent and A. Jemal, CA Cancer J. Clin., 2015, 65, 87–108 CrossRef PubMed.
- J. A. Marrero, L. M. Kulik, C. B. Sirlin, A. X. Zhu, R. S. Finn, M. M. Abecassis, L. R. Roberts and J. K. Heimbach, Hepatology, 2018, 68, 723–750 CrossRef.
- J. M. Llovet, R. Montal, D. Sia and R. S. Finn, Nat. Rev. Clin Oncol., 2018, 15, 599–616 CrossRef.
- I. Dagogo-Jack and A. T. Shaw, Nat. Rev. Clin Oncol., 2018, 15, 81–94 CrossRef CAS.
- M. W. Dewhirst and T. W. Secomb, Nat. Rev. Cancer, 2017, 17, 738–750 CrossRef PubMed.
- C. Ayuso, J. Rimola, R. Vilana, M. Burrel, A. Darnell, A. Garcia-Criado, L. Bianchi, E. Belmonte, C. Caparroz, M. Barrufet, J. Bruix and C. Bru, Eur. J. Radiol., 2018, 101, 72–81 CrossRef PubMed.
- A. Gerbes, F. Zoulim, H. Tilg, J. F. Dufour, J. Bruix, V. Paradis, R. Salem, M. Peck-Radosavljevic, P. R. Galle, T. F. Greten, J. C. Nault and M. A. Avila, Gut, 2018, 67, 380–388 CrossRef CAS PubMed.
- X. Hou, Y. Tao, Y. Pang, X. Li, G. Jiang and Y. Liu, Int. J. Cancer, 2018, 143, 3050–3060 CrossRef CAS.
- Y. Liu, P. Bhattarai, Z. Dai and X. Chen, Chem. Soc. Rev., 2019, 48, 2053–2108 RSC.
- B. C. Wilson and R. A. Weersink, Photochem. Photobiol., 2020, 96, 219–231 CrossRef CAS.
- H. S. Jung, P. Verwilst, A. Sharma, J. Shin, J. L. Sessler and J. S. Kim, Chem. Soc. Rev., 2018, 47, 2280–2297 RSC.
- M. Wang, Polymers, 2016, 8(10), 373 CrossRef.
- L. Zou, H. Wang, B. He, L. Zeng, T. Tan, H. Cao, X. He, Z. Zhang, S. Guo and Y. Li, Theranostics, 2016, 6, 762–772 CrossRef CAS PubMed.
- X. Huang, J. Wu, M. He, X. Hou, Y. Wang, X. Cai, H. Xin, F. Gao and Y. Chen, Mol. Pharm., 2019, 16, 2172–2183 CrossRef CAS.
- Z. Chen, P. Zhao, Z. Luo, M. Zheng, H. Tian, P. Gong, G. Gao, H. Pan, L. Liu, A. Ma, H. Cui, Y. Ma and L. Cai, ACS Nano, 2016, 10, 10049–10057 CrossRef CAS.
- Z. Sheng, D. Hu, M. Zheng, P. Zhao, H. Liu, D. Gao, P. Gong, G. Gao, P. Zhang, Y. Ma and L. Cai, ACS Nano, 2014, 8, 12310–12322 CrossRef CAS.
- Y. Su, Y. Liu, X. Xu, J. Zhou, L. Xu, X. Xu, D. Wang, M. Li, K. Chen and W. Wang, ACS Appl. Mater. Interfaces, 2018, 10, 38700–38714 CrossRef CAS PubMed.
- L. Wang, H. Lin, X. Chi, C. Sun, J. Huang, X. Tang, H. Chen, X. Luo, Z. Yin and J. Gao, Small, 2018, 14, e1801612 CrossRef.
- X. W. Zhang, X. J. Yan, Z. R. Zhou, F. F. Yang, Z. Y. Wu, H. B. Sun, W. X. Liang, A. X. Song, V. Lallemand-Breitenbach, M. Jeanne, Q. Y. Zhang, H. Y. Yang, Q. H. Huang, G. B. Zhou, J. H. Tong, Y. Zhang, J. H. Wu, H. Y. Hu, H. de The, S. J. Chen and Z. Chen, Science, 2010, 328, 240–243 CrossRef CAS.
- X. Chi, Z. Yin, J. Jin, H. Li, J. Zhou, Z. Zhao, S. Zhang, W. Zhao, C. Xie, J. Li, M. Feng, H. Lin, X. Wang and J. Gao, Nanotechnology, 2017, 28, 445101 CrossRef.
- H. Liu, Z. Zhang, X. Chi, Z. Zhao, D. Huang, J. Jin and J. Gao, Sci. Rep., 2016, 6, 31009 CrossRef CAS.
- K. Zhang, H. Lin, J. Mao, X. Luo, R. Wei, Z. Su, B. Zhou, D. Li, J. Gao and H. Shan, Biomater. Sci., 2019, 7, 2480–2490 RSC.
- T. D. Zhang, P. F. Zhang, S. R. Wang and T. Y. Han, Heilongjiang Med. J., 1973, 3, 66–67 Search PubMed.
- F. X. Rong and T. D. Zhang, J. New Med. Pharm., 1979, 6, 31–34 Search PubMed.
- J. Mao, L. Ma, Y. Shen, K. Zhu, R. Zhang, W. Xi, Z. Ruan, C. Luo, Z. Chen, X. Xi and S. Chen, Cell Death Dis., 2018, 9, 963 CrossRef.
- L. Leu and L. Mohassel, Am J Health Syst Pharm, 2009, 66, 1913–1918 CrossRef CAS PubMed.
- G. R. Sahu and R. K. Jena, Am. J. Hematol., 2005, 78, 113–116 CrossRef CAS PubMed.
- B. Pelaz, C. Alexiou, R. A. Alvarez-Puebla, F. Alves, A. M. Andrews, S. Ashraf, L. P. Balogh, L. Ballerini, A. Bestetti, C. Brendel, S. Bosi, M. Carril, W. C. Chan, C. Chen, X. Chen, X. Chen, Z. Cheng, D. Cui, J. Du, C. Dullin, A. Escudero, N. Feliu, M. Gao, M. George, Y. Gogotsi, A. Grunweller, Z. Gu, N. J. Halas, N. Hampp, R. K. Hartmann, M. C. Hersam, P. Hunziker, J. Jian, X. Jiang, P. Jungebluth, P. Kadhiresan, K. Kataoka, A. Khademhosseini, J. Kopecek, N. A. Kotov, H. F. Krug, D. S. Lee, C. M. Lehr, K. W. Leong, X. J. Liang, M. L. Lim, L. M. Liz-Marzan, X. Ma, P. Macchiarini, H. Meng, H. Mohwald, P. Mulvaney, A. E. Nel, S. Nie, P. Nordlander, T. Okano, J. Oliveira, T. H. Park, R. M. Penner, M. Prato, V. Puntes, V. M. Rotello, A. Samarakoon, R. E. Schaak, Y. Shen, S. Sjoqvist, A. G. Skirtach, M. G. Soliman, M. M. Stevens, H. W. Sung, B. Z. Tang, R. Tietze, B. N. Udugama, J. S. VanEpps, T. Weil, P. S. Weiss, I. Willner, Y. Wu, L. Yang, Z. Yue, Q. Zhang, Q. Zhang, X. E. Zhang, Y. Zhao, X. Zhou and W. J. Parak, ACS Nano, 2017, 11, 2313–2381 CrossRef CAS PubMed.
- S. Wilhelm, A. J. Tavares, Q. Dai, S. Ohta, J. Audet, H. F. Dvorak and W. C. W. Chan, Nat. Rev. Mater., 2016, 1, 16014 CrossRef CAS.
- H. Chen, S. Pazicni, N. L. Krett, R. W. Ahn, J. E. Penner-Hahn, S. T. Rosen and T. V. O'Halloran, Angew. Chem., Int. Ed., 2009, 48, 9295–9299 CrossRef CAS PubMed.
- Z. Zhao, X. Wang, Z. Zhang, H. Zhang, H. Liu, X. Zhu, H. Li, X. Chi, Z. Yin and J. Gao, ACS Nano, 2015, 9, 2749–2759 CrossRef CAS PubMed.
- J. Xin, K. Zhang, J. Huang, X. Luo, X. Gong, Z. Yang, H. Lin, H. Shan and J. Gao, Biomater. Sci., 2018, 7, 262–271 RSC.
- Q. Zhang, M. R. Vakili, X. F. Li, A. Lavasanifar and X. C. Le, Biomaterials, 2014, 35, 7088–7100 CrossRef CAS.
- C. Sun, H. Lin, X. Gong, Z. Yang, Y. Mo, X. Chen and J. Gao, J. Am. Chem. Soc., 2020, 142, 198–206 CrossRef CAS.
- Q. Jin, J. Liu, W. Zhu, Z. Dong, Z. Liu and L. Cheng, ACS Appl. Mater. Interfaces, 2018, 10, 332–340 CrossRef CAS PubMed.
- J. Xie, Y. Zheng and J. Y. Ying, J. Am. Chem. Soc., 2009, 131, 888–889 CrossRef CAS.
- S. Pan, L. Pei, A. Zhang, Y. Zhang, C. Zhang, M. Huang, Z. Huang, B. Liu, L. Wang, L. Ma, Q. Zhang and D. Cui, Biomaterials, 2020, 230, 119606 CrossRef CAS PubMed.
- P. Prasad, C. R. Gordijo, A. Z. Abbasi, A. Maeda, A. Ip, A. M. Rauth, R. S. DaCosta and X. Y. Wu, ACS Nano, 2014, 8, 3202–3212 CrossRef CAS PubMed.
- Q. Chen, C. Liang, C. Wang and Z. Liu, Adv. Mater., 2015, 27, 903–910 CrossRef CAS PubMed.
- Y. Wei, Y. Wang, D. Xia, S. Guo, F. Wang, X. Zhang and Y. Gan, ACS Appl. Mater. Interfaces, 2017, 9, 25138–25151 CrossRef CAS PubMed.
- F. Schettini, M. Giuliano, S. De Placido and G. Arpino, Cancer Treat. Rev., 2016, 50, 129–141 CrossRef CAS PubMed.
- Y. B. Peng, Z. L. Zhao, T. Liu, X. Li, X. X. Hu, X. P. Wei, X. B. Zhang and W. H. Tan, Angew. Chem., Int. Ed., 2017, 56, 10845–10849 CrossRef CAS PubMed.
- Z. Yang, M. Yang and J. Peng, Drug Dev. Ind. Pharm., 2008, 34, 834–839 CrossRef CAS PubMed.
- S. K. Sun, L. X. Dong, Y. Cao, H. R. Sun and X. P. Yan, Anal. Chem., 2013, 85, 8436–8441 CrossRef CAS PubMed.
- H. Nosrati, A. Rakhshbahar, M. Salehiabar, S. Afroogh, K. M. Hamidreza, H. Danafar and S. Davaran, J. Mol. Liq., 2018, 271, 639–646 CrossRef CAS.
- B. Zhang, H. Jin, Y. Li, B. Chen, S. Liu and D. Shi, J. Mater. Chem., 2012, 22, 14494 RSC.
- X. Lv, X. Wang, T. Li, C. Wei, Y. Tang, T. Yang, Q. Wang, X. Yang, H. Chen, J. Shen, H. Yang and H. Ke, Small, 2018, 14, e1802904 CrossRef PubMed.
- T. Su, J. Liao, Z. Dai, L. Xu, S. Chen, Y. Wang, Z. Peng, Q. Zhang, S. Peng and M. Kuang, Oncogene, 2018, 37, 3514–3527 CrossRef CAS PubMed.
- L. Shi, J. Wang, N. Ding, Y. Zhang, Y. Zhu, S. Dong, X. Wang, C. Peng, C. Zhou, L. Zhou, X. Li, H. Shi, W. Wu, X. Long, C. Wu and W. Liao, Nat. Commun., 2019, 10, 5421 CrossRef.
- S. Yoshida, M. Kornek, N. Ikenaga, M. Schmelzle, R. Masuzaki, E. Csizmadia, Y. Wu, S. C. Robson and D. Schuppan, Hepatology, 2013, 58, 1667–1680 CrossRef CAS.
- S. Dong, J. Kong, F. Kong, J. Kong, J. Gao, S. Ke, S. Wang, X. Ding, W. Sun and L. Zheng, J. Transl. Med., 2013, 11, 273 CrossRef PubMed.
- R. Wei, X. Gong, H. Lin, K. Zhang, A. Li, K. Liu, H. Shan, X. Chen and J. Gao, Nano Lett., 2019, 19, 5394–5402 CrossRef CAS.
- Z. Zhang, H. Liu, H. Zhou, X. Zhu, Z. Zhao, X. Chi, H. Shan and J. Gao, Nanoscale, 2016, 8, 4373–4380 RSC.
Footnotes |
† Electronic supplementary information (ESI) available. See DOI: 10.1039/d1bm01374b |
‡ Equal contribution. |
|
This journal is © The Royal Society of Chemistry 2022 |