DOI:
10.1039/D1BM01597D
(Paper)
Biomater. Sci., 2022,
10, 258-269
Multifunctional FeS2@SRF@BSA nanoplatform for chemo-combined photothermal enhanced photodynamic/chemodynamic combination therapy†
Received
15th October 2021
, Accepted 11th November 2021
First published on 16th November 2021
Abstract
Combination therapy has been widely studied due to its promising applications in tumor therapy. However, a sophisticated nanoplatform and sequential irradiation with different laser sources for phototherapy complicate the treatment process. Unlike the integration of therapeutic agents, we report a FeS2@SRF@BSA nanoplatform for the combination of chemo-combined photothermal therapy (PTT) enhanced photodynamic therapy (PDT) and chemodynamic therapy (CDT) to achieve an “all-in-one” therapeutic agent. Ultrasmall FeS2 nanoparticles (NPs) with a size of 7 nm exhibited higher Fenton reaction rates due to their large specific surface areas. A photodynamic reaction could be triggered and could generate 1O2 to achieve PDT under 808 nm irradiation. FeS2 NPs also exhibited the desired photothermal properties under the same wavelength of the laser. The Fenton reaction and photodynamic reaction were both significantly improved to accumulate more reactive oxygen species (ROS) with an increase of temperature under laser irradiation. Besides, loading of the chemotherapeutic drug sorafenib (SRF) further improved the efficacy of tumor treatment. To realize long blood circulation, bovine serum albumin (BSA) was used as a carrier to encapsulate FeS2 NPs and SRF, remarkably improving the biocompatibility and tumor enrichment ability of the nanomaterials. Additionally, the tumors on mice treated with FeS2@SRF@BSA almost disappeared under 808 nm irradiation. To sum up, FeS2@SRF@BSA NPs possess good biocompatibility, stability, and sufficient therapeutic efficacy in combination therapy for cancer treatment. Our study pointed out a smart design of the nanoplatform as a multifunctional therapeutic agent for combination cancer therapy in the near future.
1. Introduction
As a promising alternative to traditional cancer therapy, novel therapies has drawn increasing attention owing to their high efficacy and relative non-invasiveness.1–3 Therein, PDT refers to the killing of cancer cells via toxic ROS through irradiation of a photosensitizer (PS).4–7 Despite numerous trials, clinical translation and application of PDT are seriously hindered, mainly due to the superficial light penetration and the low oxygen level at the tumor site.8–11 In the meantime, ferrous-based inorganic functional nanomaterials, including ferrous oxides,12–15 ferrous sulfide,16–19 and M(Sn, Mn)Fe2O4,20,21 tend to release ferrous ions gradually and generate highly oxidized hydroxyl radicals (˙OH) at tumor sites through a Fenton reaction to achieve CDT, enhanced by the tumor microenvironment (TME).22–24 Unfortunately, CDT alone is not effective as expected due to the low level of H2O2 and low catalytic efficiency in vivo. To improve the efficiency of PDT and CDT, increasing the temperature at the tumor site has become a widely accepted method.25,26 For the PDT/PTT combination therapy, there is a spectral mismatch between the PS for PDT and PA for PTT, respectively. Furthermore, the lasers with two different wavelengths are both required for continuous irradiation to activate PDT and PTT, leading to a prolonged treatment time and complicated treatment process.27–32 To this end, Younis et al. reported a nanometer platform with a PDT agent (indocyanine green, ICG) anchoring onto the dual plasmonic PTT nanoagents (Au NRs/MoS2 hybrid) and achieved simultaneous PDT/PTT under single low power NIR laser irradiation.6 Unlike sophisticated integration of various agents, it has become an urgent issue to establish a nanoplatform that could realize combination therapy with a single agent.
Therefore, in this study, we focused on developing an “all in one” theranostic system for PTT enhanced PDT/CDT combination therapy with a simple nano-agent with single wavelength laser irradiation. It has been reported that ultrasmall multifunctional FeS2 NPs catalyze the formation of ROS to achieve CDT.33 Additionally, FeS2 NPs generate localized heat in the photothermal treatment and photoinduced electron/hole pairs to achieve PDT under 808 nm laser irradiation.34–37 Thus, FeS2 NPs integrate multiple functions including CDT, PDT and PTT for the combination therapy. Jin et al. developed the protein-assisted synthesis of ultrasmall FeS2 using bioremineralization.35 The tumors in FeS2@BSA-Ce6-treated mice completely disappeared. Nevertheless, the combination of PTT/PDT required two different laser wavelengths due to the effect of PDT from the photosensitizer Ce6. The employment of two lasers for synergistic therapy was cumbersome. On the other hand, Qu et al. synthesized a FeS2@C-PEG yolk–shell nanostructure with appropriate size for photodynamic and photothermal treatment.34 Unexpectedly, the actual loading rate of PS molecules was not taken into consideration, missing the superiority of the “all-in-one” treatment method. Therefore, it is of great significance to rationally design a multifunctional FeS2 nanoplatform to achieve the combination therapy.
Herein, we aim to develop an “all-in-one” therapeutic agent and establish a FeS2@SRF@BSA nanoplatform to realize chemo-combined PTT enhanced PDT/CDT for tumor therapy. Ultrasmall FeS2 NPs have a large surface area, which dramatically increased the contact area with H2O2 and greatly increased the ROS level. Meanwhile, FeS2 NPs also exhibited efficient photothermal properties, increasing the local temperature under 808 nm laser irradiation to further facilitate the reaction rate of CDT/PDT as “all-in-one” theranostic agents. In addition, loading of the chemotherapeutic drug SRF inhibited tumor cell proliferation as well as angiogenesis, and improved the ROS level, further promoting the accumulation of ROS, leading to enhancement of tumor treatment. To encapsulate FeS2 NPs and SRF, bovine serum albumin (BSA) is selected as a nano-drug carrier to form FeS2@SRF@BSA NPs. BSA entrapment improves the biocompatibility and tumor enrichment of the designed nanoplatform. More importantly, FeS2@SRF@BSA NPs can be well internalized by tumor cells, produce ROS and release SRF effectively to kill tumor cells, resulting in sufficient in vivo antitumor efficacy in a xenograft tumor model. In brief, with the synergistic effect of chemo-combined PTT enhanced PDT/CDT combination therapy, FeS2@SRF@BSA NPs served as “all-in-one” theranostic agents for cancer treatment.
2. Materials and methods
2.1 Materials
Ferrous chloride tetrahydrate (FeCl2·4H2O, 99%), 3-(4,5-dimethyl-2-thiazolyl)-2,5-diphenyl tetrazolium bromide (MTT), coumarin-6 (C6), 2′,7′-dichlorofluorescein diacetate (DCFH-DA) and the fluorescein isothiocyanate (FITC) isomer were obtained from Sigma-Aldrich. Calcein AM/PI was purchased from Gibco Life Technologies. Thioglycolic acid (TGA, 99%) and sodium thiosulfate pentahydrate (Na2S2O3·5H2O, 99.5%) were purchased from Macklin (Shanghai, China). SRF was bought from Meilun Biotechnology (Dalian, China). BSA, Dulbecco's Modified Eagle's medium (DMEM), fetal bovine serum (FBS), 0.05% trypsin-EDTA and penicillin–streptomycin solution (penicillin: 105 IU mL−1, streptomycin: 102 mg mL−1) were bought from Thermo Fisher Scientific Inc. (WI, USA). The murine breast cancer cells (4T1), 3T3 cells and human umbilical vein endothelial cells (HUVECs) were purchased from the National Collection of Authenticated Cell Cultures (Shanghai, China). 4,6-Diamidino-2-phenylindole (DAPI) was purchased from Beyotime (Shanghai, China).
2.2 Synthesis of FeS2 NPs
0.130 g of FeCl2·4H2O and 200 μL of TGA were dissolved in dimethyl sulfoxide (DMSO, 45 mL). Nitrogen was then bubbled in the solution for 30 min to remove oxygen. 0.725 g of Na2S2O3·5H2O dissolved in 5 mL of deionized water was then added dropwise under continuous stirring in an N2 environment for 15 min. The mixed solution was refluxed at 140 °C for 4 h to enable the growth and crystallization of the FeS2 NPs. After that, FeS2 NPs were collected via centrifugation (11
000 rpm, 20 min). The black solid product was washed with ethanol and deionized water three times and lyophilized under a vacuum freeze dryer.
2.3 Synthesis of FeS2@SRF@BSA NPs
BSA solution (1 mg mL−1) and glutaraldehyde solution (8%, w/v) were freshly prepared. SRF (2 mg) was dissolved in tetrahydrofuran (THF, 500 μL) and mixed with FeS2 NPs solution under ultrasonication for 90 seconds. The solution was added dropwise into 5 mL of BSA solution at a speed of 1 mL min−1 under stirring. Then, 5 μL of glutaraldehyde solution was added into the mixed solution. The mixed solution was stirred in a poor O2 environment for 3 h. The FeS2@SRF@BSA NPs were then collected via centrifugation (13
000 rpm, 5 min) and washed with deionized water. Similarly, FeS2@BSA NPs were synthesized via the addition of FeS2 NPs alone. The products were dispersed in deionized water and stored at −80 °C.
2.4 Material characterization
Transmission electron microscopy (TEM) images were obtained using a Hitachi H-7650 instrument. The crystal structure was monitored by X-Ray diffraction (XRD) using an X'TRA diffractometer (ARL, Switzerland). Elemental analysis was performed by X-Ray photoelectron spectroscopy (XPS, ESCALab 250, Thermo Scientific, USA). The sizes of nanomaterials were detected via a Zetasizer Nano system. The content of ferrous ions was determined by inductively coupled plasma-atomic emission spectrometry (ICP-AES, IRIS-HR, TJA Co, USA).
2.5 Photothermal properties
To explore the photothermal properties of FeS2 NPs and the influence of BSA, FeS2 NPs and FeS2@SRF@BSA NPs at various concentrations were irradiated under 808 nm at 1.0 W cm−2 for 10 min, respectively. The temperature was monitored using an electronic thermometer. Temperature fluctuations of the materials were recorded to test the photothermal stability. For the detection of the photothermal conversion efficiency, the solution was continuously irradiated (808 nm, 1 W cm−2) until reaching a stable level. After turning off the laser, the solution was cooled down to the initial temperature. The photothermal conversion efficiency (η) was obtained using the formula, | 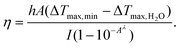 | (1) |
where h is the heat transfer coefficient, A is the surface area of the container, ΔTmax,min is the temperature change of the nanoparticle dispersion at the maximum steady-state temperature, ΔTmax,H2O is the temperature change of water at the maximum steady-state temperature, I is the laser power and Aλ is the absorbance factor of nanoparticles at 808 nm in aqueous solution.
2.6 Detection of ROS production
FeS2@SRF@BSA NPs were dissolved in N,N-dimethyl-4-nitrosoaniline (RNO) solutions under different pH conditions (1.55 mg mL−1 of L-histidine solution, 3 μg mL−1 of RNO in PBS solution) in the dark and mixed sufficiently. Then, H2O2 solution (100 μM) was added and treated with or without a laser (808 nm, 1 W cm−2). The ultraviolet absorbance at 440 nm was recorded at a preset time point to detect the ROS production and determine the PDT/CDT effects of the materials.
2.7
In vitro SRF release
The SRF loading capacity at FeS2@SRF@BSA NPs was measured at 264 nm using high performance liquid chromatography (HPLC, LC-2030, Shimadzu, Japan). The column temperature was maintained at 25 °C with a mobile phase consisting of acetonitrile and water at a volume ratio of 75
:
25 and a flow rate of 1 mL min−1. The supernatant during the above synthesis process was collected at 264 nm. The drug loading (DL) capacity was calculated using the following equation: |  | (2) |
The drug release of FeS2@SRF@BSA NPs in vitro was studied by the dialysis method. SRF-loaded NPs were suspended under different pH conditions and dialyzed using 10 mL of PBS (37 °C, 72 h). The amount of SRF released from NPs was determined by HPLC. To assess the additional effects of laser to drug release, SRF-loaded NPs were dispersed in PBS at different pH values and irradiated (808 nm, 1 W cm−2, 5 min) for 2 h, 6 h and 10 h, respectively.
2.8 Cellular uptake
4T1 cells were grown in DMEM supplemented with 10% (v/v) of FBS and penicillin (100 IU mL−1)/streptomycin (100 μg mL−1) in a humidified 5% CO2 at 37 °C. Cellular uptake of the nanoparticles was detected by flow cytometry (FC) and confocal laser scanning microscopy (CLSM). 4T1 cells were seeded in 6-well plates (3 × 105 cells per well) and incubated for 24 h. FeS2 NPs or FeS2@BSA NPs were labeled with C6 dye and stirred overnight in the dark to load onto the nanoparticles. Fresh medium containing FeS2@C6 NPs or FeS2@BSA@C6 NPs was added and cultured for different times. The cells were collected, centrifuged (1000 rpm, 5 min), washed with PBS, filtered and then analyzed by FC.
For CLSM analysis, a 4T1 cell suspension was seeded in 35 mm imaging dishes and incubated in DMEM for 24 h. The primary medium was replaced with fresh medium containing FeS2@C6 NPs or FeS2@BSA@C6 NPs at a C6 concentration of 0.2 μg mL−1 at the same volume, and cultured for different times. After incubation, the cells were washed with PBS three times, fixed with 4% (w/v) of paraformaldehyde solution and then stained with DAPI solution (10 μg mL−1) for CLSM. DAPI (blue) was used to detect nuclei (C6: Ex = 466 nm, Em = 504 nm; DAPI: Ex = 340 nm, Em = 488 nm).
2.9 Determination of the production of intracellular ROS
The intracellular ROS level was determined by FC and CLSM. 4T1 cells were seeded in 6-well plates for 24 h (3 × 105 cells per well). Fresh medium was supplied containing SRF, FeS2 NPs, FeS2@BSA NPs or FeS2@SRF@BSA NPs and further cultured for 8 h. Serum-free medium was added containing DCFH-DA (10 μM) to each well and incubated in the dark for 30 min. After washing with PBS 3 times, 808 nm laser irradiation was conducted at 1 W cm−2 for 5 min. Cells without laser treatment were used as a control. The cells were then resuspended in PBS and analyzed by FC.
For CLSM, 4T1 cells were cultured for 24 h in 35 mm imaging dishes. Fresh medium was supplied containing SRF, FeS2 NPs, FeS2@BSA NPs or FeS2@SRF@BSA NPs, and then cultured for another 8 h. Serum-free medium containing DCFH-DA (10 μM) was added to each well and incubated in the dark for 30 min. After incubation, the cells were washed with PBS 3 times, and then subjected to 808 nm laser irradiation (1 W cm−2, 5 min). Cells without laser treatment were used as a control. Then the cells were stained with DAPI (10 μg mL−1) for CLSM.
2.10 Cell viability
4T1 cells were seeded into a 96-well plate (3000 cells per well). After 24 h, the cells were cultured with samples at various concentrations for another 8 h and then treated with or without a laser (808 nm, 1 W cm−2, 5 min). After incubation for 24 h, the cells were washed with PBS, supplied with 100 μL of fresh medium and 20 μL of MTT solution (5 mg mL−1) and cultured for another 4 h. The medium in each well was replaced with 140 μL of DMSO and the absorbance was monitored using a microplate reader at 570 nm. The cytotoxicity was expressed as the percentage of cell viability relative to the untreated control group. FeS2 or FeS2@BSA NPs (the concentration of Fe ranging from 6.25 to 200 μg mL−1) were added to 3T3 cells and HUVEC cells and incubated for 24 h to determine the cellular compatibility.
A Calcein-AM/PI staining kit was used to detect live/dead cells in 12 well plates. 4T1 cells were incubated with SRF, FeS2 NPs, FeS2@BSA NPs, or FeS2@SRF@BSA NPs for 8 h, and then irradiated with a laser (808 nm, 1 W cm−2, 5 min) or without a laser. After incubation for 24 h, Calcein-AM (0.67 μM) and PI (1.5 μM) were incubated in buffer solution for 15 min. Images were obtained with a fluorescence microscope.
2.11 Cell apoptosis assay
4T1 cells were incubated with SRF, FeS2 NPs, FeS2@BSA NPs, or FeS2@SRF@BSA NPs for 8 h and treated with or without a laser (808 nm, 1 W cm−2, 5 min). After incubation for 24 h, the cells were collected and resuspended in 500 μL of binding buffer containing Annexin V-FITC (5 μL) and PI (5 μL) for 15 min in the dark. The fluorescence intensity signal was detected using a flow cytometer.
2.12
In vitro and in vivo NIR imaging
4T1 cells were incubated with FeS2 NPs, FeS2@SRF@BSA NPs (the concentration of Fe was at 50 μg mL−1 in DMEM) and PBS groups for 8 h and treated with a laser (808 nm, 1 W cm−2, 5 min). During this process, an infrared thermal camera (Fluke Ti27 IR Fusion Technology) was used to record the real-time temperature. Tumor-bearing FeS2 NP, FeS2@SRF@BSA NP (5 mg kg−1 of Fe) and PBS groups were exposed to a laser (808 nm, 1 W cm−2, 5 min) after 24 h of injection. The increase in the temperature of the tumor site was monitored and imaged simultaneously using an infrared thermal imager.
2.13
In vivo fluorescence imaging
BALB/c mice (5 weeks old, 16–20 g, female) were obtained from the Sun Yat-sen University Laboratory Animal Center. The animals used have been reviewed and approved by the Institutional Animal Care and Use Committee (IACUC), Sun Yat-Sen University, and the approval number is SYSU-IACUC-2021-000639. 4T1 cells (1 × 107 cells) were injected subcutaneously into the right backside of each mouse to prepare tumor-bearing mice. All the in vivo experiments were conducted in compliance with the criteria of the National Regulation of China for Care and Use of Laboratory Animals. The Cy5.5 dye was mixed with FeS2 NPs or FeS2@BSA NPs and stirred overnight in the dark to load onto the nanoparticles. Tumor-bearing mice were injected with FeS2@Cy5.5 NPs or FeS2@BSA@Cy5.5 NPs, respectively. Fluorescence images were performed in vivo at different time points (4 h, 8 h, 12 h, 24 h and 48 h). After injection for 48 h, the main organs and tumors of the mice were removed for in vitro fluorescence imaging and quantitative fluorescence analysis.
2.14
In vivo cancer therapy
Mice with the tumor volume reaching ∼100 mm3 were randomly divided into eight groups (5 mice per group) for different treatments: (a) PBS; (b) SRF; (c) FeS2 NPs; (d) FeS2 NPs with a laser; (e) FeS2@BSA NPs; (f) FeS2@BSA NPs with a laser; (g) FeS2@SRF@BSA NPs; (h) FeS2@SRF@BSA NPs with a laser (5 mg kg−1 mouse of Fe dose, a 808 nm laser with 1 w cm−2 for 5 min). The nanoplatform was administered via the caudal vein on day 0. The tumor sizes and body weights were measured every day and the tumor volumes were calculated using the equation: V = (length) × (width)2/2. The Kaplan–Meier survival curves were obtained by recording the percentage of mice when the tumor volume was >1500 mm3 or the mice died. The mice were sacrificed at day 30 and the tumors as well as major organs were collected. Tumor paraffin sections were stained with hematoxylin and eosin (H&E), and subjected to TUNEL to determine the anti-tumor effect.
2.15
In vivo safety evaluation
To explore the biosafety of the materials, blood biochemical index analysis and tissue analysis were performed on experimental animals. Mice in the treatment group and control group were sacrificed at 30 days, and their blood was collected for further analysis. Major organs (heart, liver, spleen, lungs, kidneys) were collected and stained with hematoxylin and eosin (H&E).
2.16. Statistical analysis
Group variation was presented as the Mean ± Standard Deviation (S.D.). Differences between each group were regarded statistically significant at *p < 0.05, highly significant at **p < 0.01, and ***p < 0.001 was considered very highly statistically significant as assessed by Student's t-test.
3 Results and discussion
3.1 Material synthesis and characterization
Ultrasmall FeS2 NPs were synthesized via a facile thermal injection reaction.38 TEM images showed that FeS2 NPs were predominantly in the uniform spherical phase, with an average diameter of ∼7 nm (Fig. 1A and B). To improve the passive tumor targeting ability of the NPs, FeS2 NPs and SRF were embedded with BSA to form composite NPs. The size of the NPs increased to ∼100 nm with irregular spherical agglomeration (Fig. 1C and D). We incubated the FeS2@SRF@BSA NPs with the supernatant of lysed 4T1 cells and observed the size decrease of the NPs, which was possibly caused by the release of FeS2 from NPs after incubation (Fig. S1A and B†). FeS2 NPs were characterized by XRD to confirm the crystal structures (Fig. 1E). All the diffraction peaks were in accordance with the FeS2 standard peaks (PDF no. 42-1340). The chemical constitution of FeS2 NPs was determined by XPS (Fig. 1F–H). The Fe 2p binding energies of 707.2 eV and 720.1 eV belonged to the Fe2+ species of FeS2 NPs. The peak of S 2p3/2 at 162.7 eV and S 2p1/2 at 163.8 eV also confirmed the presence of sulfur in FeS2 NPs. The results above indicated the successful synthesis of FeS2 NPs as expected. FeS2@SRF@BSA NPs were also characterized by XRD and XPS. The results in Fig. S2† indicated that the entrapment of BSA and loading of SRF did not change the structure and construction of the nanoplatform. Furthermore, the suspension stability test of FeS2@SRF@BSA NPs revealed the expected stability in various physiological solutions. The size of the NPs remained stable in PBS containing 10% FBS for 3 days, implying the favorable suspension stability of FeS2@SRF@BSA (Fig. S3†). In addition, the UV-vis absorption spectra of FeS2@SRF@BSA NPs revealed the successful synthesis of FeS2@SRF@BSA (Fig. S4†). The SRF loading of FeS2@SRF@BSA is 8.3% (wt%) according to HPLC measurement, which was appropriate for effective drug delivery and treatment.
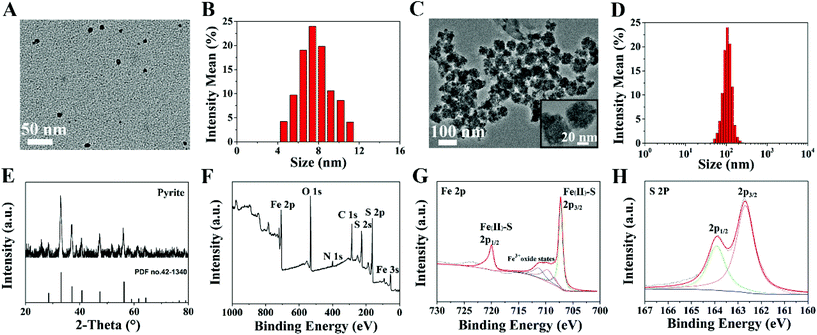 |
| Fig. 1 (A) TEM image and (B) particle size distribution of FeS2 NPs. (C) TEM image and (D) particle size distribution of FeS2@SRF@BSA NPs. (E) XRD of the synthesized FeS2 NPs. (F) XPS full spectrum of FeS2 NPs. (G) XPS spectra of Fe 2p and (H) S 2p for FeS2 NPs. | |
To assess the photothermal effects of FeS2 and FeS2@SRF@BSA NPs, each aqueous dispersion was irradiated with an 808 nm laser at a power intensity of 1.0 W cm−2 for 10 min. Both FeS2 NPs and FeS2@SRF@BSA NPs exhibited an obvious exothermic heating trend under irradiation (Fig. 2A). The photothermal effect of FeS2@SRF@BSA NPs was slightly weaker than that of FeS2 NPs, possibly caused by the thermal conductivity and viscosity of the medium around the NPs. Fortunately, when the irradiation power was 1 W cm−2, the temperature in FeS2@SRF@BSA solution would heat up to 41.6 °C within 10 min at a Fe concentration of 50 μg mL−1, indicating that the photothermal property of NPs was not seriously affected by albumin. The photothermal heating curve of FeS2@SRF@BSA NPs indicated that the heating property was power-dependent and concentration-dependent (Fig. 2B and C). The heating curve of FeS2 NP solution exhibited similar trends (Fig. S5A and B†). The heating property of the FeS2@SRF@BSA solution did not change significantly after multiple cycles of laser irradiation and natural cooling, revealing the desired photothermal stability (Fig. S5C†). Moreover, both FeS2 NPs and FeS2@SRF@BSA NPs exhibited high photothermal conversion efficiencies of 48.2% and 45.5%, respectively (Fig. S6†). Consequently, the synthesized FeS2@SRF@BSA NPs exhibited effective photothermal heating and photothermal conversion abilities as photothermal agents.
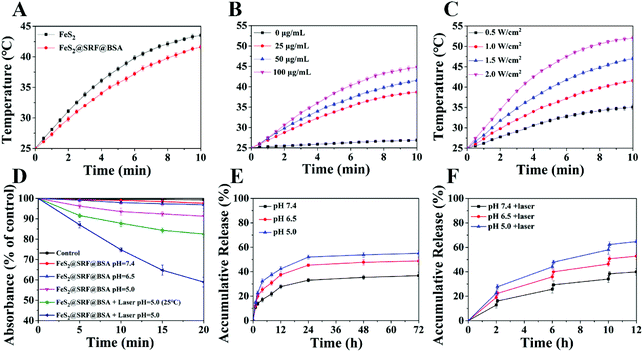 |
| Fig. 2 (A) Temperature increment curves of FeS2, the FeS2@SRF@BSA suspension (Fe: 50 μg mL−1) under 808 nm NIR illumination (1.0 W cm−2). Temperature increment curves of FeS2@SRF@BSA suspensions with various (B) concentrations and (C) irradiation densities. (D) Relative absorbance of RNO under different conditions. (E) SRF release profiles from FeS2@SRF@BSA at different pH values. (F) SRF release profiles from FeS2@SRF@BSA with or without NIR laser irradiation. | |
In the following, we detected the ROS production to investigate the CDT/PDT efficiency of FeS2@SRF@BSA NPs at three different pH values (Fig. 2D). The decrease of the RNO content at pH 5.0 was slightly higher than that at pH 6.5 or pH 7.4, reflecting a mild pH dependence. ROS accumulation was only caused by the ˙OH from the Fenton reaction in the absence of laser irradiation. Next, to compare the temperature effect on ROS generation, a water bath was used to control the temperature under laser irradiation. At the same pH value (5.0), the RNO content decreased by 17.5% under laser irradiation at 25 °C, indicating that the energy from an 808 nm laser reacted with oxygen molecules and produced 1O2via the photodynamic effect. The specific surface area of the NPs increased the contact area with O2 and increased the incident light with the generation of ROS significantly increasing. More importantly, in the absence of temperature control, the absorbance value of RNO significantly decreased by 41.4%, since FeS2@SRF@BSA NPs could rapidly increase the local temperature under 808 nm laser irradiation to further increase the reaction rate of CDT/PDT. The results clearly reflected PTT enhanced ROS generation and the advantage of “all-in-one” theranostic agents.
The drug release of FeS2@SRF@BSA NPs was then determined at different pH values (5.0, 6.5 and 7.4). In Fig. 2E, an acidic solution (pH 5.0) was found to accelerate the SRF release, reaching 55.7% in 72 h, consistent with previous reports.39,40 Next, the influence of laser irradiation on the release of SRF was investigated. As shown in Fig. 2F, after three cycles of irradiation, the maximum release percentage of SRF reached 64.7% within 12 h at a pH value of 5.0. Heating generated by irradiation induced the release of SRF from the BSA or FeS2@SRF@BSA NPs, contributing to effective tumor therapy.
3.2
In vitro cell experiments
To test the cellular uptake efficiency of coumarin-loaded nanodrugs FeS2@C6 NPs and FeS2@BSA@C6 NPs, 4T1 cells were incubated with NPs for different times (2 h, 4 h, 6 h, 8 h, and 12 h). FC results (Fig. 3A, and Fig. S7A†) and quantitative analysis are shown in Fig. 3B. Internalization of NPs is a complicated process, influenced by particle size, particle morphology, and the appearance of a surface trimer. The uptake level of NPs depended on the incubation time. FeS2@C6 NPs were ingested faster than FeS2@BSA@C6 NPs and reached a plateau at 6 h. While the mean fluorescence intensity of FeS2@BSA@C6 NPs reached a maximum at 8 h, after which the uptake began to reduce. The internalization level in the FeS2@BSA@C6 NP group was slightly higher than that of the FeS2@C6 NP group at 8 h. Since BSA encapsulation could enhance blood circulation, FeS2@BSA@C6 NPs were more advantageous for in vivo treatment, so 8 h was selected as the interval between drug administration and laser irradiation in the following experiments. Meanwhile, CLSM results showed that the NPs coupled with C6 were effectively absorbed by 4T1 cells within 8 hours (Fig. 3C) and fluorescence semi-quantitative analysis of the images is shown in Fig S7B.
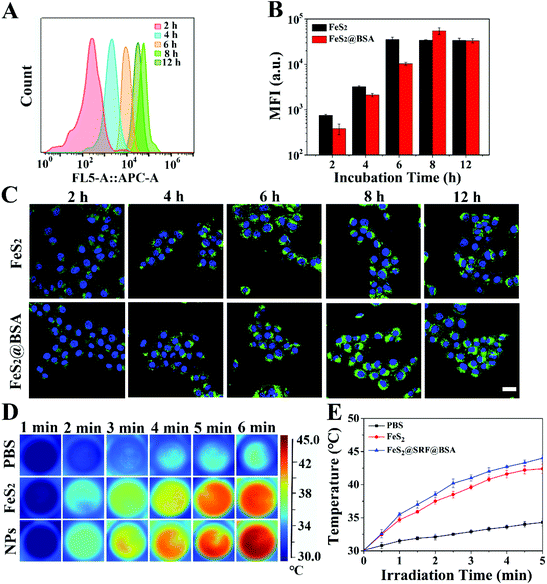 |
| Fig. 3 (A) Fluorescence histograms of the 4T1 cells incubated with FeS2@BSA@C6 NPs for different times. (B) The quantitative analysis of FC (MFI, mean fluorescence intensity). (C) Fluorescence images of 4T1 cells incubated with FeS2@C6 NPs or FeS2@BSA@C6 NPs for 2 h, 4 h, 6 h, 8 h, and 12 h (scale bar: 30 μm). (D) The temperature variation of cells in 6-well plates treated with different formulations for 8 h, before or after irradiation (808 nm, 1.0 W cm−2) for different times. NPs in the figures refer to the FeS2@SRF@BSA nanoplatform. (E) Temperature changes of medium during laser irradiation. | |
Additionally, in Fig. 3D, FeS2@SRF@BSA NPs induced intracellular hyperthermia when exposed to a laser. In the control group, the medium had a moderate temperature increase to 34.3 °C with irradiation (808 nm, 1.0 W cm−2, 5 min), while the temperature of the experimental groups increased rapidly in the presence of FeS2 NPs or FeS2@SRF@BSA NPs. The temperature of the FeS2@SRF@BSA NP group increased rapidly to 44 °C after irradiation (Fig. 3E). The increase of temperature greatly enhanced the Fenton reaction and photocatalytic reaction. The obvious intracellular photothermal performance of FeS2@SRF@BSA NPs provided the capacity for in vivo combined therapy.
Intracellular ROS produced by FeS2@SRF@BSA NPs were detected using 2,7-dichlorodihydrofluorescein diacetate (DCFH-DA) as a probe by FC (Fig. 4A) and CLSM (Fig. 4B). The green fluorescence was enhanced with an increase of intracellular ROS. The negative group containing only cells and the PBS group containing cells and DCFH-DA were used as controls. The PBS group showed only sporadic green fluorescence, suggesting the presence of a small amount of endogenous ROS in cells. For the FeS2 NP group, FeS2@BSA NP group and FeS2@SRF@BSA NP group, the fluorescence signal was stronger than that of the control group, due to the Fenton reaction mediated by Fe2+ to generate ˙OH. As expected, the cells of the three groups showed bright green fluorescence signals after irradiation, indicating the accumulation of a large amount of intracellular ROS under the synergistic effect of NIR laser irradiation and FeS2@SRF@BSA NPs. Laser irradiation caused the increase of temperature and facilitated the effect of the Fenton reaction and photodynamic reaction. Besides, from CLSM images, it can be observed that 4T1 cells treated with the FeS2@SRF@BSA NP group under laser irradiation exhibited significantly stronger fluorescence than other groups, since FeS2@SRF@BSA NPs exhibited the best internalization ability owing to their BSA encapsulation. Meanwhile, it has been reported that SRF could induce ROS production in tumor cells.41 These results indicated that FeS2@SRF@BSA NPs could effectively increase the ROS amount in cells. In brief, FeS2@SRF@BSA NPs presented the advantage of “all-in-one” and realized PTT enhanced CDT/PDT.
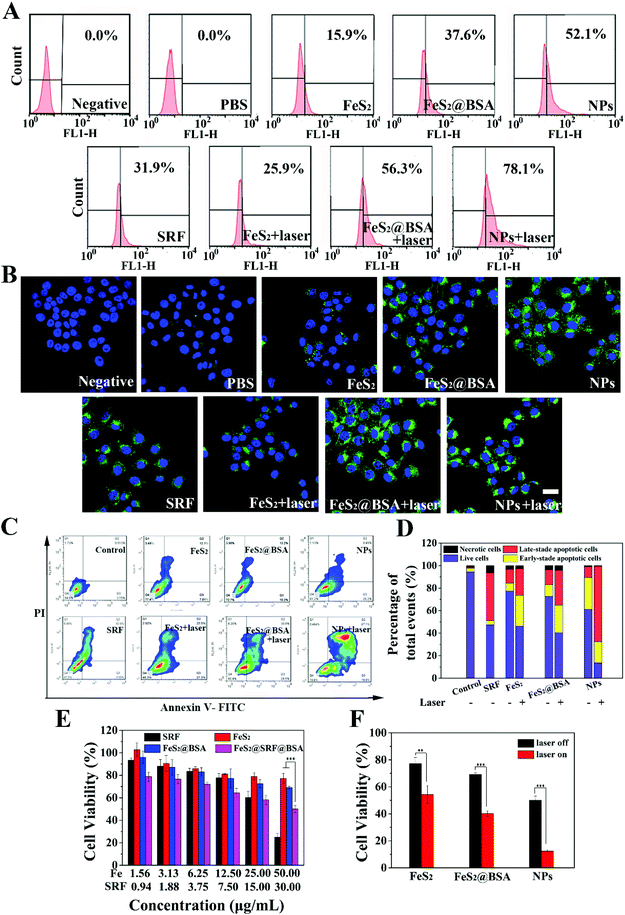 |
| Fig. 4 (A) FC and (B) CLSM analysis of intracellular ROS level in 4T1 cells with different treatments. ROS production detected by fluorescence of DCFH-DA (green) in 4T1 cells, and DAPI (blue) was used to detect nuclei (scale bar: 30 μm). (C) FC apoptosis assay of 4T1 cells caused by FeS2 NPs, FeS2@BSA NPs, and FeS2@SRF@BSA NPs with or without irradiation (808 nm, 1.0 W cm−2, 5 min) and (D) quantitative analysis. (E) The cell viability of 4T1 cells incubated with the nanoparticles at different concentrations. (F) The cell viability of 4T1 cells incubated with the nanoparticles with or without NIR laser irradiation. (Fe: 50 μg mL−1) (808 nm, 1.0 W cm−2, 5 min). NPs in the figures refer to the FeS2@SRF@BSA nanoplatform (*p < 0.05, **p < 0.01, ***p < 0.001). | |
The cell apoptosis was caused by a combination of multiple effects. FC was employed to test the cell viability of 4T1 cells treated by our designed nanoplatform (Fig. 4C and D). The apoptotic rate of the group with BSA was higher than that of the NPs alone, reflecting the increase of BSA entrapment to cell internalization of FeS2 NPs and the anti-tumor effect of NPs. After laser irradiation, the apoptosis rate of FeS2@SRF@BSA NPs treated cells was dramatically increased, showing that FeS2@SRF@BSA NPs could induce apoptosis of tumor cells through chemo-combined PTT enhanced PDT/CDT combination therapy. Meanwhile, the potential cytotoxicity of FeS2 NPs and FeS2@BSA NPs was tested via the MTT method (Fig. S8†). The relative survival rate of cells incubated with FeS2 NPs and FeS2@BSA NPs was higher than 80% at a Fe concentration of 200 μg mL−1, indicating the mild cytotoxicity of materials to normal tissues. Owing to the acidic environment and high level of hydrogen peroxide in 4T1 cells, the MTT assay was carried out to further determine the antitumor activity of the material in vitro. The 4T1 cells were treated with FeS2 NPs, FeS2@BSA NPs or FeS2@SRF@BSA NPs at a series of concentrations for 8 h, with or without irradiation (808 nm, 1.0 W cm−2, 5 min, Fig. 4E and F). The cell viability decreased gradually with an increase in the concentration of Fe, induced by the Fenton reaction to induce CDT. When the Fe concentration was 50 μg mL−1, the cell viability of the FeS2@SRF@BSA NP group was 50%, which was much lower than that of the FeS2 group. The appropriate drug release rate of FeS2@SRF@BSA NPs enhanced the antitumor effect, with a cell viability of only 12.4% in the FeS2@SRF@BSA NP group after laser irradiation. Laser irradiation could stimulate the photodynamic effect of FeS2@SRF@BSA NPs and increase the temperature, thus improving the reaction rate of CDT/PDT. These results demonstrated the anti-tumor ability of FeS2@SRF@BSA NPs by chemo-combined PTT enhanced PDT/CDT combination therapy. In order to visually determine the cytotoxicity of the NPs, 4T1 cells were co-stained with Calcein-AM/PI. The dead or live cells were differentiated by red and green fluorescence, respectively (Fig. S9†). When the Fe concentration reached 50 μg mL−1 and the irradiation time was 5 min, cell mortality was extremely high. The results further confirmed the antitumor effect using FeS2@SRF@BSA NPs as an NIR-triggered PDT/CDT nanoplatform.
3.3
In vivo imaging and biodistribution
To further investigate the distribution of FeS2 NPs and FeS2@BSA NPs in tumors and various organs, Cy5.5 dye labeled NPs were injected into 4T1 tumor-bearing mice through the tail vein, and their enrichment in the tumor site was monitored by fluorescence imaging (Fig. 5A and C). The fluorescence signal of FeS2 NPs was mainly distributed in liver tissues and it is less in tumors, exhibiting the poor tumor targeting ability. After 48 h of injection, the fluorescence signal of FeS2 NPs almost disappeared. The in vivo circulation time was short for the small size of FeS2 NPs, while FeS2@BSA NPs showed a strong fluorescence intensity and a relatively long duration in tumor tissue. With the extension of circulation time in vivo, the maximum fluorescence intensity was obtained in the tumor site at 24 h. All mice were dissected 48 hours after injection. The main organs and tumors of the mice were removed for in vivo fluorescence imaging (Fig. 5B and D). The fluorescence intensity of FeS2@SRF@BSA NPs in the tumor site was much higher than that of the FeS2 group. BSA entrapment increased the particle size and effectively improved the tumor enrichment ability of FeS2 NPs. The NPs were also distributed in the liver, kidneys and other organs, and gradually expelled from the body over time.
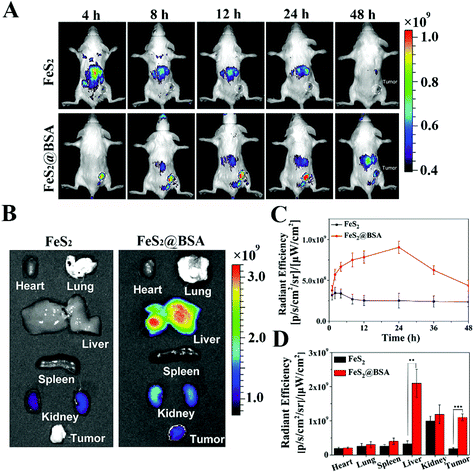 |
| Fig. 5 (A) In vivo fluorescence imaging of 4T1 cancer-bearing mice after the injection of FeS2 NPs and FeS2@BSA NPs at different time points. (B) Ex vivo fluorescence imaging of major tissues at 48 h post injection. (C) Semiquantitative fluorescence intensity statistics of tumor tissues at different times. (D) The quantification of the fluorescence intensity of various tissues (*p < 0.05, **p < 0.01, ***p < 0.001). | |
3.4
In vivo combined tumor therapy
On the basis of the results from in vitro experiments, we further explored the combined anti-tumor ability of NPs on 4T1 cancer-bearing mice. 4T1 cancer-bearing mice were randomly divided into 8 groups: (a) PBS, (b) SRF, (c) FeS2, (d) FeS2 with a laser, (e) FeS2@BSA, (f) FeS2@BSA with a laser, (g) FeS2@SRF@BSA, and (h) FeS2@SRF@BSA with a laser (5 mg kg−1 mouse of Fe dose, an 808 nm laser with 1 w cm−2 and 5 min). The nanoparticles or saline were intravenously injected three times (0 d, 3 d, 6 d). Mice of laser groups were exposed to an 808 nm laser at 24 h after each injection. A real-time temperature change of the tumor site was monitored using an infrared thermal camera (Fig. 6A). Under the irradiation of a laser (808 nm, 1 W cm−2), the tumor site temperature of the mice increased from 39 °C to 53 °C within 5 min after injection of FeS2@SRF@BSA NPs (Fig. 6B). The temperature range was suitable for photothermal treatment, and the increase of temperature was much higher than that of the control group. Nevertheless, FeS2 NPs alone exhibited a slight temperature increase, mainly due to the seldom accumulation at the tumor site. The results above indicated that FeS2@SRF@BSA NPs achieved the desired photothermal therapeutic effect and provided the appropriate temperature for PTT to enhance CDT and PDT. Furthermore, the effect of the combination therapy was studied in vivo. The groups with FeS2 had CDT, while the groups with FeS2 had laser integrated CDT, PDT and PTT. The visual image of the tumor tissue (Fig. 6C) and the changes in the average tumor volume of each group on a daily basis (Fig. 6D) were presented. Without laser irradiation, the tumor volume treated by FeS2 NPs, FeS2@BSA NPs or FeS2@SRF@BSA NPs only slightly inhibit the tumor with the tumor growth rate still being relatively fast, which could be attributed to the anti-tumor effect of the Fenton reaction by FeS2 NPs in the absence of laser irradiation. In contrast, the tumor growth of the laser groups was significantly inhibited. The FeS2@SRF@BSA NPs with laser groups showed the best therapeutic effect. BSA entrapment is attributed to improving the tumor enrichment ability of FeS2 NPs effectively for the production of ROS to clear the tumor effectively. Besides, laser irradiation promoted the release of chemotherapeutic drugs. SRF accurately acted at tumor sites and inhibited the growth of tumor cells and angiogenesis. The results above demonstrated that FeS2@SRF@BSA NPs with an 808 nm laser gave the obvious therapeutic effect of chemo-combined PTT enhanced PDT/CDT combination therapy. H&E staining and TUNEL staining further performed at the tumor site of the control group showed no obvious damage, while those performed at the tumor site of FeS2@SRF@BSA NPs (laser group) showed a large number of apoptotic cells, which was consistent with the treatment results (Fig. 6E and F). In addition, mice in the FeS2@SRF@BSA NPs with laser groups survived up to 30 days until they were executed, while 60% of mice in the control group died or exceeded the established end-value 1500 mm3 after 22 days of treatment (Fig. S10†). Thus, the FeS2@SRF@BSA NPs with laser group could effectively prolong the survival time of mice. Therefore, FeS2@SRF@BSA NPs had the promising potential to be a clinical nanodrug for chemo-combined PTT enhanced PDT/CDT combination therapy.
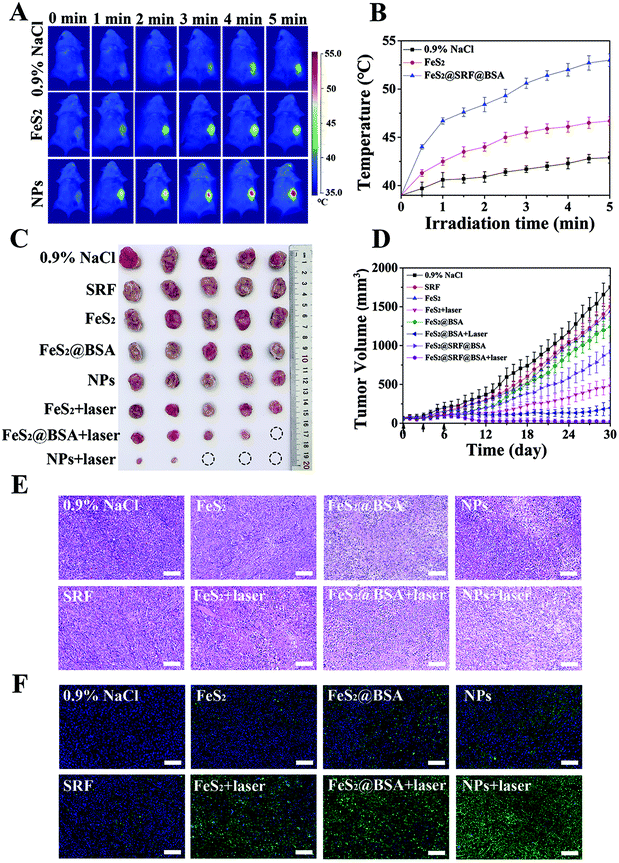 |
| Fig. 6 (A) The infrared thermal images of 4T1-bearing mice after injection of FeS2 NPs and FeS2@SRF@BSA NPs under different irradiation times. (808 nm, 1.0 W cm−2). (B) Temperature changes of tumors monitored during laser irradiation. (C) The photograph of tumors collected from different mice groups. (D) Relative tumor volume curves of different mice groups after the various treatments (n = 5). (E) H&E and (F) TUNEL staining of tumor tissue. NPs in the figures refer to FeS2@SRF@BSA (scale bar: 100 μm). | |
For the application of nanomaterials in vivo, the biosafety of NPs should be particularly taken into consideration. During 30 d of treatment, there was no significant difference in the body weight between the treatment group and the control group, reflecting the high biocompatibility of FeS2@SRF@BSA NPs (Fig. S11†). Then H&E staining of major organs in each group showed no significant histopathological changes in the biopsy, suggesting negligible long-term adverse toxicity (Fig. S12†). Hepatic function indicators in each group were used to identify potential toxicity in vivo, including alanine aminotransferase (ALT), aspartate aminotransferase (AST), and alkaline phosphatase (ALP) as well as renal function indicators including blood urea nitrogen (BUN), creatinine (CRE) and creatine kinase (CK). All these results were at the normal reference level, suggesting low toxicity to the liver and kidneys (Fig. S13†).
4. Conclusion
In this study, FeS2@SRF@BSA NPs were established to achieve a chemo-combined PTT enhanced PDT/CDT combination therapy. A facile thermal injection reaction method was developed to prepare ultrasmall FeS2 NPs with a size of 7 nm, which gave a superior reaction rate of CDT/PDT and achieved better ROS generation ability due to their large specific surface areas. The “all-in-one” multifunctional FeS2 NPs exhibited a high level of photothermal efficiency under 808 nm irradiation at 1.0 W cm−2 for 5 min, which significantly improved the CDT and PDT effects with an increase in temperature. Additionally, modification of SRF further improved the efficacy of tumor therapy. In vitro and in vivo anti-tumor experiments showed that the designed nanoplatform dramatically inhibited the growth of 4T1 tumors when laser irradiation was employed. More importantly, encapsulation of FeS2 NPs and SRF with BSA led to NPs with a size of ∼100 nm to maintain long blood circulation. In vivo imaging revealed efficient tumor accumulation of those NPs after injection. To sum up, our study illustrated a simple and smart strategy of NPs with the integration of multiple functionalities for chemo-combined PTT enhanced PDT/CDT cancer combination therapy.
Conflicts of interest
There are no conflicts to declare.
Acknowledgements
This work was supported by the National Natural Science Foundation of China (51773231), the Natural Science Foundation of Guangdong Province (2014A030312018, 2016A030313315) and the Science, Technology and Innovation Commission of Shenzhen Municipality (JCYJ20190807160801664).
References
- D. Dolmans, D. Fukumura and R. K. Jain, Nat. Rev. Cancer, 2003, 3, 380–387 CrossRef CAS.
- A. Hak, V. R. Shinde and A. K. Rengan, Photodiagn. Photodyn. Ther., 2021, 33, 102205–102212 CrossRef CAS PubMed.
- E. Jung, J. Lee, Y. Lee, S. Seon, M. Park, C. Song and D. Lee, ACS Appl. Bio Mater., 2021, 4, 4450–4461 CrossRef CAS.
- S. Kwiatkowski, B. Knap, D. Przystupski, J. Saczko, E. Kedzierska, K. Knap-Czop, J. Kotlinska, O. Michel, K. Kotowski and J. Kulbacka, Biomed. Pharmacother., 2018, 106, 1098–1107 CrossRef CAS.
- K. I. Dhanalekshmi, P. Magesan, K. Sangeetha, X. Zhang, K. Jayamoorthy and N. Srinivasan, Photodiagn. Photodyn. Ther., 2019, 28, 324–329 CrossRef CAS.
- M. R. Younis, C. Wang, R. B. An, S. J. Wang, M. A. Younis, Z. Q. Li, Y. Wang, A. Ihsan, D. J. Ye and X. H. Xia, ACS Nano, 2019, 13, 2544–2557 CAS.
- L. Cheng, C. Wang, L. Z. Feng, K. Yang and Z. Liu, Chem. Rev., 2014, 114, 10869–10939 CrossRef CAS PubMed.
- X. S. Li, J. F. Lovell, J. Yoon and X. Y. Chen, Nat. Rev. Clin. Oncol., 2020, 17, 657–674 CrossRef PubMed.
- Z. J. Xie, T. J. Fan, J. An, W. Choi, Y. H. Duo, Y. Q. Ge, B. Zhang, G. H. Nie, N. Xie, T. T. Zheng, Y. Chen, H. Zhang and J. S. Kim, Chem. Soc. Rev., 2020, 49, 8065–8087 RSC.
- N. Kudinova and T. Berezov, Biomed. Khim., 2009, 55, 558–569 CAS.
- P. Agostinis, K. Berg, K. A. Cengel, T. H. Foster, A. W. Girotti, S. O. Gollnick, S. M. Hahn, M. R. Hamblin, A. Juzeniene, D. Kessel, M. Korbelik, J. Moan, P. Mroz, D. Nowis, J. Piette, B. C. Wilson and J. Golab, CA-Cancer J. Clin., 2011, 61, 250–281 CrossRef PubMed.
- S. Lei, L. A. Wang, F. X. Lin, K. Zeng, M. Z. Wanga and X. W. Gea, Chin. J. Chem. Phys., 2020, 33, 376–384 CrossRef CAS.
- W. X. Du, T. Z. Liu, F. F. Xue, X. J. Cai, A. Chen, Y. Y. Zheng and H. R. Chen, ACS Appl. Mater. Interfaces, 2020, 12, 19285–19294 CrossRef CAS.
- H. W. Zhang, F. Lu, W. Pan, Y. G. Ge, B. J. Cui, S. H. Gong, N. Li and B. Tang, Biomater. Sci., 2021, 9, 3814–3820 RSC.
- L. Wang, M. Huo, Y. Chen and J. Shi, Biomaterials, 2018, 163, 1–13 CrossRef CAS PubMed.
- D. J. She, S. J. Peng, L. Liu, H. H. Huang, Y. Y. Zheng, Y. P. Lu, D. Y. Geng and B. Yin, Chem. Eng. J., 2020, 400, 12 CrossRef.
- F. Wu, Q. C. Zhang, M. Zhang, B. H. Sun, Z. C. She, M. Q. Ge, T. Y. Lu, X. H. Chu, Y. Wang, J. X. Wang, N. L. Zhou and A. Li, ACS Appl. Mater. Interfaces, 2020, 12, 10142–10155 CrossRef CAS PubMed.
- S. T. Xiao, Y. Lu, M. Feng, M. Dong, Z. Cao, X. G. Zhang, Y. Chen and J. Liu, Chem. Eng. J., 2020, 396, 13 CrossRef.
- Z. M. Tang, H. L. Zhang, Y. Y. Liu, D. L. Ni, H. Zhang, J. W. Zhang, Z. W. Yao, M. Y. He, J. L. Shi and W. B. Bu, Adv. Mater., 2017, 29, 8 Search PubMed.
- K. T. Lee, Y. J. Lu, F. L. Mi, T. Burnouf, Y.-T. Wei, S.-C. Chiu, E.-Y. Chuang and S. Y. Lu, ACS Appl. Mater. Interfaces, 2017, 9, 1273–1279 CrossRef CAS.
- J. C. Shen, T. W. Rees, Z. G. Zhou, S. P. Yang, L. N. Ji and H. Chao, Biomaterials, 2020, 251, 14 CrossRef.
- Z. M. Tang, Y. Y. Liu, M. Y. He and W. B. Bu, Angew. Chem., Int. Ed., 2019, 58, 946–956 CrossRef CAS PubMed.
- J. Xin, C. Deng, O. Aras, M. Zhou, C. Wu and F. An, J. Nanobiotechnol., 2021, 19, 192 CrossRef CAS PubMed.
- T. He, Y. Yuan, C. Jiang, N. T. Blum, J. He, P. Huang and J. Lin, Angew. Chem., Int. Ed., 2021, 60, 6047–6054 CrossRef CAS.
- A. C. V. Doughty, A. R. Hoover, E. Layton, C. K. Murray, E. W. Howard and W. R. Chen, Materials, 2019, 12, 14 CrossRef.
- Y. L. Li, Y. B. Deng, X. Tian, H. T. Ke, M. Guo, A. J. Zhu, T. Yang, Z. Q. Guo, Z. S. Ge, X. L. Yang and H. B. Chen, ACS Nano, 2015, 9, 9626–9637 CrossRef CAS.
- Y. Cao, H. F. Dong, Z. Yang, X. M. Zhong, Y. Chen, W. H. Dai and X. J. Zhang, ACS Appl. Mater. Interfaces, 2017, 9, 159–166 CrossRef CAS.
- Q. Y. Jia, J. C. Ge, W. M. Liu, S. Liu, G. L. Niu, L. Guo, H. Y. Zhang and P. F. Wang, Nanoscale, 2016, 8, 13067–13077 RSC.
- M. Guo, H. J. Mao, Y. L. Li, A. J. Zhu, H. He, H. Yang, Y. Y. Wang, X. Tian, C. C. Ge, Q. L. Peng, X. Y. Wang, X. L. Yang, X. Y. Chen, G. Liu and H. B. Chen, Biomaterials, 2014, 35, 4656–4666 CrossRef CAS PubMed.
- Y. Jang, S. Kim, S. Lee, C. M. Yoon, I. Lee and J. Jang, Chem. – Eur. J., 2017, 23, 3719–3727 CrossRef CAS.
- W. S. Chen, J. Ouyang, H. Liu, M. Chen, K. Zeng, J. P. Sheng, Z. J. Liu, Y. J. Han, L. Q. Wang, J. Li, L. Deng, Y. N. Liu and S. J. Guo, Adv. Mater., 2017, 29, 7 Search PubMed.
- B. Li, Q. Zhou, H. Y. Wang, Y. C. Zha, P. L. Zheng, T. Yang, D. Ma, L. Qiu, X. M. Xu, Y. Hu, A. Roig, S. M. Yu and W. Xue, Chem. Eng. J., 2021, 403, 17 Search PubMed.
- C. Pan, M. T. Ou, Q. Z. Cheng, Y. Zhou, Y. K. Yu, Z. M. Li, F. Zhang, D. H. Xia, L. Mei and X. Y. Ji, Adv. Funct. Mater., 2020, 30, 11 Search PubMed.
- M. Li, H. M. Lin and F. Y. Qu, Chem. Eng. J., 2020, 384, 10 Search PubMed.
- Q. T. Jin, J. J. Liu, W. J. Zhu, Z. L. Dong, Z. Liu and L. Cheng, ACS Appl. Mater. Interfaces, 2018, 10, 332–340 CrossRef CAS.
- Z. Q. Meng, F. Wei, W. J. Ma, N. Yu, P. L. Wei, Z. J. Wang, Y. Q. Tang, Z. G. Chen, H. P. Wang and M. F. Zhu, Adv. Funct. Mater., 2016, 26, 8231–8242 CrossRef CAS.
- S. Khalid, E. Ahmed, Y. Khan, K. N. Riaz and M. A. Malik, ChemistrySelect, 2018, 3, 6488–6524 CrossRef CAS.
- Y. X. Bai, J. Yeom, M. Yang, S. H. Cha, K. Sun and N. A. Kotov, J. Phys. Chem. C, 2013, 117, 2567–2573 CrossRef CAS.
- T. Liu, W. L. Liu, M. K. Zhang, W. Y. Yu, F. Gao, C. X. Li, S. B. Wang, J. Feng and X. Z. Zhang, ACS Nano, 2018, 12, 12181–12192 CrossRef CAS PubMed.
- C. G. Qian, J. C. Yu, Y. L. Chen, Q. Y. Hu, X. Z. Xiao, W. J. Sun, C. Wang, P. J. Feng, Q. D. Shen and Z. Gu, Adv. Mater., 2016, 28, 3313–3320 CrossRef CAS.
- Q. Q. Guan, R. M. Guo, S. H. Huang, F. Zhang, J. Liu, Z. Y. Wang, X. Yang, X. T. Shuai and Z. Cao, J. Controlled Release, 2020, 320, 392–403 CrossRef CAS.
Footnote |
† Electronic supplementary information (ESI) available. See DOI: 10.1039/d1bm01597d |
|
This journal is © The Royal Society of Chemistry 2022 |