DOI:
10.1039/D1AN01895G
(Paper)
Analyst, 2022,
147, 101-108
A peroxidase-like activity-based colorimetric sensor array of noble metal nanozymes to discriminate heavy metal ions†
Received
18th October 2021
, Accepted 8th November 2021
First published on 9th November 2021
Abstract
Heavy metal ions (HMIs), including Cu2+, Ag+, Cd2+, Hg2+, and Pb2+ from the environment pose a threat to human beings and can cause a series of life-threatening diseases. Therefore, colorimetric sensors with convenience and flexibility for HMI discrimination are still required. To provide a solution, a peroxidase-like activity-based colorimetric sensor array of citrate-capped noble metal nanozymes (osmium, platinum, and gold) has been fabricated. Some studies reported that some HMIs could interact with the noble metal nanozymes leading to a change in their peroxidase-like activity. This phenomenon was confirmed in our work. Based on this principle, different concentrations of HMIs (Cu2+, Ag+, Cd2+, Hg2+, and Pb2+) were discriminated. Moreover, their practical application has been tested by discriminating HMIs in tap water and SiYu lake water. What is more, as an example of the validity of our method to quantify HMIs at nanomolar concentrations, the LOD of Hg2+ was presented. To sum up, our study not only demonstrates the differentiation ability of this nanozyme sensor array but also gives hints for using nanozyme sensor arrays for further applications.
Introduction
Due to the increase in development in industry and other related sectors, water containing heavy metal ions (HMIs) is continuously discharged into the ecosystem, particularly in developing countries.1 Thus, an excessive amount of HMIs accumulate in organisms and due to their nonbiodegradability, high toxicity, and high water solubility, HMIs cause severe problems to human beings, including by acting as mutagens and carcinogens.2,3Additionally, they can cause adverse effects on the bones, nervous system, kidneys, endocrine system, and so on.4–7 Accordingly, facile and reliable methods for the detection of HMI levels in water are needed for safeguarding public health and protecting the ecosystem.8 Common analytical techniques for HMI monitoring are generally spectroscopy-based approaches including atomic absorption spectroscopy,9 ion-coupled-plasma spectroscopy, inductively coupled plasma emission spectroscopy,10,11 X-ray fluorescence spectroscopy,12 and inductively coupled plasma mass spectroscopy.13 Although these techniques show outstanding sensitivity and accuracy, they require complicated procedures including time-consuming sample pretreatments and detection processes, expensive instrumentation, and well-trained professionals.7,8
Recently, nanomaterials having enzymatic activity (i.e., nanozymes) were modified to overcome natural enzyme limitations including increasing their stability, decreasing the cost, and improving their ability to be used under harsh conditions.14 Since Fe3O4 nanoparticles (NPs) were discovered as promising peroxidase mimics in 2007, many nanomaterials have been examined for mimicking the behavior of natural enzymes.15 Because of their multifunctionalities and tunable catalytic activity, nanomaterials have been employed in numerous applications including therapy,16,17 biomedical detection,18–20 protection of the environment,21 and so on. Inspired by their recognition of smell and taste systems, cross-reactive sensor arrays (e.g., artificial “tongue/nose”) were improved and used as a hopeful alternative to traditional “one-to-one” biosensors.22–24 Known simply as the “sensing array” approach, they depend on selectivity rather than specificity of the analytes, and different analytes provide unique response patterns. After that, linear discrimination analysis (LDA) or other multivariate statistical methods for data analysis can be used.7,21 Recently, nanozyme-based colorimetric sensor arrays have been used to identify different targets and create unique identifying patterns, these targets include biological macromolecules,25 small molecules,26 cells,27 and bacteria.24,28–30 Compared with the electronic tongue, colorimetry is simple, sensitive, and provides a ‘naked-eye’ quantification approach.24 Moreover, it provides a fast response, rapid detection and identification, and a simple method in terms of instrumentation.31 So far, finding new ways to apply nanozyme-based colorimetry in order to improve its performance in different analytical fields is still a hot topic for researchers.
Many metal nanomaterials have been introduced with peroxidase-like activity, including Pt, Ru, Pd, Au,14 and Os.32 These noble metal-based peroxidase-like activities show many advantages over natural peroxidase (HRP)33 and nowadays many efforts are being dedicated toward extending their applications. For example, sensing of DNA,34 kanamycin,35 nuclease,36 dopamine,37 pesticides,37,38 Pb2+,39 Hg2+,40 biothiols, proteins, and cells21 has been achieved. It is interesting to note that the surface manipulation of a nanozyme can allow for the tuning of enzyme-like activities of nanozymes.41–46 It can also help with the unique interactions with various analytes.41 Analytes that can enable interaction with nanozymes and alter their surface chemistry can change the enzymatic activity of nanozymes considerably.41,42 In the same manner as natural enzymes, nanozymes can change their activities due to the presence of HMIs.41 Previous studies suggest that the surface chemistry modification of the nanozyme can improve the selectivity of the nanozyme probe for the sensing of HMIs.47–49 Metal–metal interactions, chemical interactions with nanozymes which contain oxygen or sulfur, surface ligands, complex formations, and electrostatic contacts, can all cause alterations in the enzyme's ability to mimic nanozymes when they interact with HMIs. These different types of interactions can lead to the enhancement, inhibition, or change of their catalytic activity. Thus, this phenomenon can be applied for the particular qualification and quantification of the various analytes.41 For example, Pt NPs were used for the sensing of Hg2+. The Pt0 reduced Hg2+ to Hg and formed an amalgam on the surface of the Pt NPs. This formation resulted in the suppression of the peroxidase activity of the Pt NPs.50 Although there is high selectivity of Pt NPs, the interference between other HMIs can happen.51 Also, the catalytic activity (oxidase or peroxidase) of different nanozymes has been varied in the presence of several HMIs including Cu2+, Ag+, Cd2+, Hg2+, Pb2+, uranium, and arsenate.48,50,52,53 What is more, nanozymes can aggregate in high ionic strength media, which can cause an alteration of catalytic activity and lead to interference.41 So, the selection of a suitable ligand could resist the aggregation and produce reliable results.
Secondly, nanomaterials have been applied for the construction of many biosensor arrays, which are not only cheaper and more stable but also more convenient in manufacturing and modification.26 So far, the detection of HMIs has not attracted much attention with nanozyme-based sensor arrays, which if applied, will present a big opportunity for nanozyme-based biosensing. Previous studies have shown that HMIs pose various kinds of interactions with noble metal nanozymes and their capped ligands. For example, a colorimetric probe using citrate-capped Pt NPs was used for the detection of Ag+. The ligand (citrate) supported the reduction of Ag+ ion to Ag0, therefore the catalytic activity of Pt NPs was inhibited (turn-off mechanism) through a ligand-assisted metallophilic interaction. However, Hg2+ has the same inhibition effect, and for discrimination, a masking agent has been used for Hg2+ removal and selective detection of Ag+.54 Also, citrate-capped Au NPs were used for the detection of Hg2+. In this study, a citrate-assisted formation of gold amalgam and reduction of Hg2+ led to an increase of the peroxidase-like activity (turn-on mechanism).55 Inspired by these, we designed a peroxidase-like activity-based colorimetric sensor array of noble metal nanozymes for HMI discrimination without using any masking agent. Citrate was selected as a ligand due to its varied interaction mechanisms with HMIs as described above. Also, three noble metal ions Os, Pt, and Au, with different responses for HMIs were selected to build a biosensor array for HMI detection with a better discrimination ability. Using this nanozyme sensor array, five HMIs were successfully distinguished in different concentrations. The quantification at the nanomolar concentration was also considered. Furthermore, the practical applications of our proposed array were validated by the differentiation of the selected HMIs in tap water and SiYu lake water (Fig. 1).
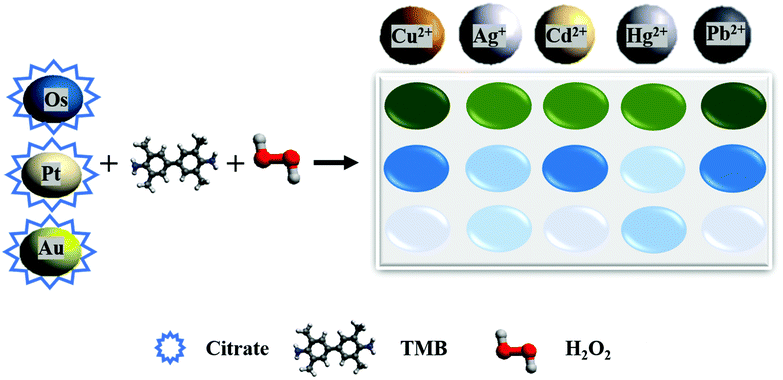 |
| Fig. 1 Schematic representation of our proposed nanozyme-based sensor array in the presence of HMIs. | |
Experimental section
Synthesis of citrate-capped nanozymes
The preparation of citrate-capped NPs was carried out according to the following steps. Briefly, 1 mL of K2OsCl6 and 1 mL of trisodium citrate dihydrate (40 mM) were mixed with 38 mL of DI water. Then, the mixture was stirred for 30 min at room temperature. After that, 200 μL of NaBH4 (50 mM) was added dropwise to the mixture. Next, this mixture was allowed to react under stirring at room temperature in dark conditions for 60 min to get citrate-Os NPs (0.25 mM). In the same way, citrate-Pt NPs (0.25 mM) and citrate-Au NPs (0.25 mM) were synthesized using 1 mL of H2PtCl6 (10 mM) and 1 mL of HAuCl4 (10 mM), respectively.32 Finally, the suspensions were stored in a refrigerator until analysis.
The peroxidase-like activities of nanozymes
The peroxidase mimicry of citrate-capped nanozymes was evaluated from their capability to catalyze the oxidation of the chromogenic substrates (TMB) in the presence of H2O2. In brief, 200 μL of TMB (6 mM) and 1 mL of H2O2 (1 mM) were added to 2.78 mL of phosphate buffer (20 mM, pH = 4). After that, a 20 μL suspension of citrate-capped nanozymes was added to the mixture. Lastly, the solution was incubated at 55 °C for 30 min before analysis.
Discrimination of different kinds of heavy metal ions
In a 96-well plate, a 5 × 6 region has been selected in which the blank and five HMIs (Cu2+, Ag+, Cd2+, Hg2+, and Pb2+) occupied a 5-well row. Briefly, phosphate buffer (55 μL, 20 mM, pH = 4) containing 5 μL of HMIs – with different concentrations – was added into each well. After that, TMB (5 μL, 6 mM), H2O2 (25 μL, 10 mM), and nanozymes (15 μL, at different concentrations) were immediately put into each well to make the total volume equal to 100 μL and incubated at 37 °C for 20 min. Next, the absorbance was measured at 652 nm using a microplate reader. Furthermore, the 5 HMIs were examined against the 3 nanozymes 5 times to generate a matrix of the training data (3 arrays × 5 HMIs × 5 replicates). The training-data matrix was analyzed using LDA.
Differentiation of heavy metal ions in real samples
In a 96-well plate, a 5 × 6 region has been selected in which the blank and five HMIs (Cu2+, Ag+, Cd2+, Hg2+, and Pb2+) occupied a 5-well row. Briefly, phosphate buffer (55 μL, 20 mM, pH = 4) containing 5 μL of HMIs was added into each well. Of note, the HMIs were prepared by using tap water and SiYu lake water. After that, TMB (5 μL, 6 mM), H2O2 (25 μL, 10 mM), and nanozymes (15 μL) were immediately put into each well to make the total volume equal to 100 μL and incubated at 37 °C for 20 min. Next, the absorbance was measured at 652 nm using a microplate reader. Furthermore, the 5 HMIs were examined against the 3 nanozymes 5 times to get a matrix of the training data (3 arrays × 5 HMIs × 5 replicates). The training data matrix was analyzed using LDA.
Results and discussion
Characterization of citrate-capped nanozymes
In this study, we selected noble metal nanozymes Os, Pt, and Au, as these elements are characterized by their stability, ease of manufacture, and easy modification of their surface, and their nanozymatic performance dependence on surface modification has been extensively studied.32,42,56–58 Also, the difference in the metallic core may influence both the enzyme-like performance and the interactions of the different analytes with the nanozyme surface.59,60 Therefore, the selectivity, due to different metallic cores, could be improved. TEM imaging was performed to verify the successful preparation of citrate-Os, citrate-Pt, and citrate-Au NPs. As shown in Fig. 2A–C, the average sizes of citrate-Os NPs, citrate-Pt NPs, and citrate-Au NPs were 1.7, 2.1, and 4.3 nm, respectively, which were calculated by Gaussian fitting. Moreover, the results show that the Os, Pt, and Au elements exist in citrate-Os NPs, citrate-Pt NPs, and citrate-Au NPs, respectively, and that the C and O elements obtained from citrate, were also observed (Fig. S1†).
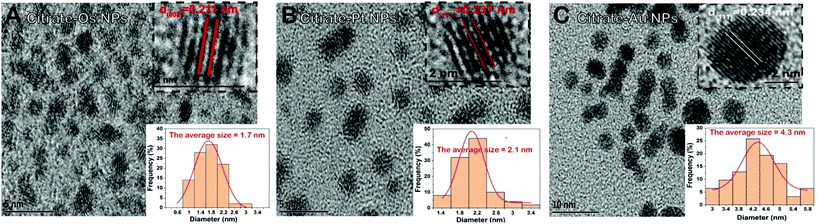 |
| Fig. 2 Illustrative TEM images of (A) Os, (B) Pt, and (C) Au nanozymes. | |
Modulation of the peroxidase-like activities of citrate-capped nanozymes by heavy metal ions
After the preparation of citrate-capped nanozymes, we studied their peroxidase-like activities, using the catalytic oxidation of the chromogenic substrates (TMB) in the presence of H2O2 at 652 nm. Fig. S2† shows good peroxidase-like activity of citrate-Os NPs, citrate-Pt NPs, and citrate-Au NPs. After that, the modulation effect of HMIs on the peroxidase-like activities was investigated. Fig. S3† shows the different effects of different HMIs on the three nanozymes. For Cu2+ and Pb2+ ions, a weak enhancement effect was observed in the case of citrate-Os, but there seems to be no enhancement effect in the case of citrate-Pt and citrate-Au. For Cd2+ ions, a weak inhibition effect was shown in the case of citrate-Pt,49 but there seems to be no inhibition effect in the case of citrate-Os and citrate-Au. Interestingly, Ag+ and Hg2+ ions can have the same response manner on two of the nanozymes as follows: either a slight enhancement toward citrate-Os or a clear inhibition effect toward citrate-Pt. Also, Ag+ and Hg2+ ions show strong enhancement effects toward citrate-Au, which was reported in previous studies.54,55 Although, there are similarities in the responses between these HMIs, our model shows a good separation ability for these HMIs (Fig. 3) without needing masking agents or any other additional steps. Based on our results, we conclude that various HMIs have different modulation influences on the enzyme-like activity of these three nanozymes. These may be attributed to different aspects. Firstly, the elevation of the hydroxyl radical (˙OH) by the decomposition of H2O2 on the surface of nanozymes. Secondly, the modulation of the surface charge of the nanozymes. Thirdly, the tendency of the analytes to interact with the nanozymes.60 Moreover, the different effects of the HMIs on a nanozyme refer to several interactions.41 Firstly, the metallophilic interaction such as Ag+, Hg2+, and Cd2+ with Pt. Secondly, metal–ligand interactions as in the case of Ag+, Cu2+, and Hg2+. Thirdly, the amalgamation on the surface of a nanozyme such as Hg2+ with Au. Fourthly, the deposition of HMIs on the nanozyme surface and the formation of multi-oxidation state complexes as in the case of Pb2+,41 which can lead to a “turn-on/off” mechanism (Fig. 3).
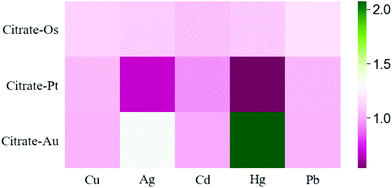 |
| Fig. 3 Heat map obtained from colorimetric response patterns of the three nanozymes against HMIs. The color bar represents (A/A0). A and A0 were the absorption of oxidized TMB in the presence and absence of HMIs in the nanozyme reaction systems, respectively. | |
Differentiation of heavy metal ions by a nanozyme sensor array
The three nanozymes were used to fabricate a nanozyme sensor array. First, we used the sensor array for the differentiation of three different concentrations of HMIs. The color change response patterns were analyzed by recording the A/A0 values. A and A0 describe the oxidized TMB absorption at 652 nm in the presence or absence of HMIs in the reaction systems, respectively. After that, the color change response pattern data were transferred to Python to generate 2D plots of linear discriminant analysis (LDA). Five HMIs were well-separated from each other into five different groups. As presented in Fig. 4A–C, the nanozyme array distinguished five HMIs with different concentrations (1.5, 2.5, and 5 μM) with an adequate separation ability confirmed the excellent distinguishing capability of HMIs in vast concentration ranges. After that, for the validation of the multiplex of the distinguishing capability of the sensor array, the mixture of various ratios of two HMIs Ag+ and Hg2+ was applied. As presented in Fig. 4D, the mixtures with various molar ratios were well-separated from each other into five different groups, confirming the feasible detection capability of the nanozyme sensor array for HMIs even with various molar ratios. It is important to note that in the case of using a single nanozyme, we cannot separate different HMIs (Ag+ and Hg2+ as examples, Fig. S4†). However, when we combined the three different nanozymes and collected the advantages of each of them (e.g., slightly enhancing in the case of citrate-Os, quenching in the case of citrate-Pt, and enhancing in the case of citrate-Au), HMIs can be successfully discriminated, due to their different characteristic responses as seen in Fig. S4.†
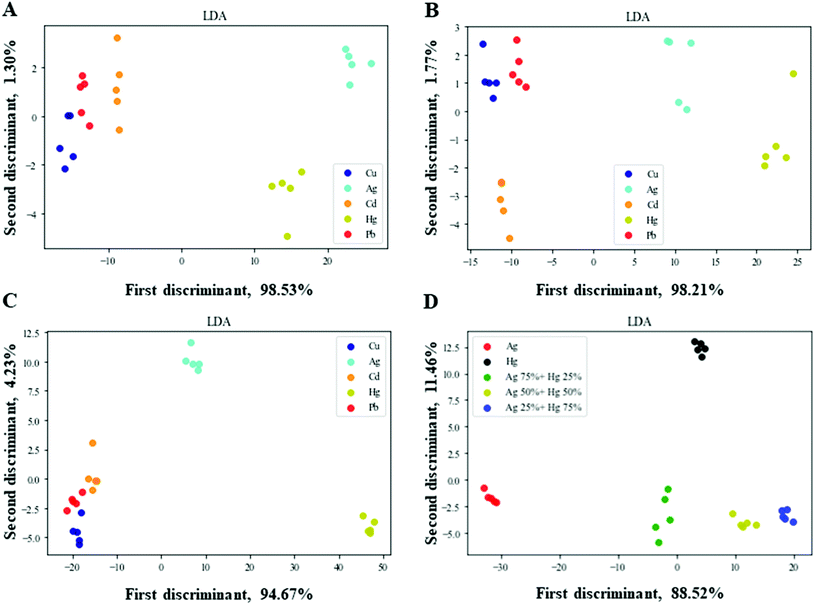 |
| Fig. 4 Nanozyme sensor array for HMI detection. Two-dimensional LDA score plots at different concentrations, (A) 1.5, (B) 2.5, (C) 5 μM of HMIs, and (D) Ag+ and Hg2+ at various molar ratios. | |
Semi-quantitative and quantitative determination of heavy metal ions
The capability of the nanozyme sensing array for the semi-quantitative determination of HMIs has been studied. For a nanozyme sensor array, distinguishing the different HMIs at various concentrations is an important topic, and overcoming the constraints of it is a focal point in the application potential of the nanozyme sensor array.60 As an example, the differentiation assays of Hg2+ with different concentrations have been studied. As shown in Fig. 5A, Hg2+ ions at different concentrations (1.5, 2.5, and 5 μM) were well-separated using LDA (other HMIs can be seen in Fig. S5†). Thus, the nanozyme sensor array showed excellent performance in the discrimination between HMIs at different concentrations.
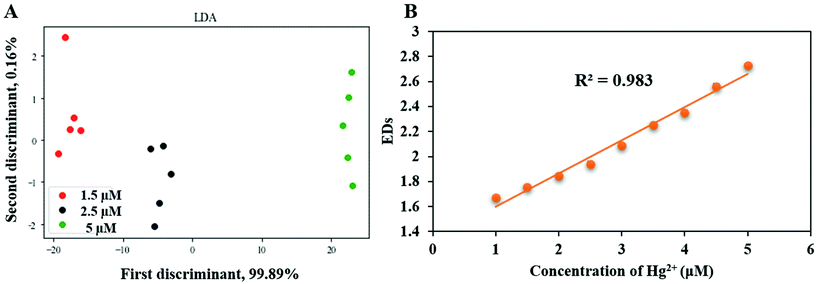 |
| Fig. 5 Nanozyme sensor array for HMI semi-quantification and quantification analyses. (A) 2D LDA score plot of Hg+ at various concentrations 1.5 μM, 2.5 μM, and 5 μM of HMIs. (B) Linear relationship of EDs of the nanozyme sensor array and concentration of Hg+ (μM). The error bars show the standard errors of the five independent experiments. | |
Besides the semi-quantitative detection, the capability of the nanozyme sensing array for quantitative detection has been studied as well. For this, a calibration graph was obtained by plotting the Euclidean distances (EDs) (Euclidean distance is the square root of the sum of the normalized squares of the A/A0 values) of the response vector of the nanozyme sensing array and the concentration of the HMIs. For this step, we selected Hg2+ at different concentrations, firstly as an example for other HMIs which an interested researcher can apply our method to other HMIs also. Secondly, due to its biological and environmental importance and because many studies have been published previously on the development of analytical techniques to detect Hg2+.7,55,61–63Fig. 5B shows an excellent linear relationship between EDs and the concentration of HMIs with a strong correlation coefficient (R2 = 0.98). Importantly, the LOD of Hg2+ was 81 nM, and was estimated according to the value of 3σ/slope, where σ is the standard deviation of the background of the colorimetric signal. Thus, our nanozyme sensor array can be used for semi-quantitative and quantitative detection of these HMIs.
Reproducibility of the nanozyme sensor array
For the reproducibility assessment of our developed nanozyme sensor array, the response of this sensor array toward the selected HMIs (five individual experiments where each one was replicated 5 times – 25 replicates in total) was recorded. The relative standard deviation (RSD) for the selected HMIs was obtained and listed in Table 1. The results show that the value of the RSD % was less than 5%. This suggests that the reproducibility of our nanozyme sensor array is acceptable for qualitative and quantitative analyses.
Table 1 Evaluation of the reproducibility of assay responses to five studied HMIs
HMIs |
Euclidean distances |
RSD (%) |
S 1 |
S 2 |
S 3 |
S 4 |
S 5 |
Cu2+ |
1.80 |
1.66 |
1.79 |
1.75 |
1.70 |
3.40 |
Ag+ |
1.76 |
1.68 |
1.78 |
1.81 |
1.74 |
2.79 |
Cd2+ |
1.79 |
1.67 |
1.77 |
1.72 |
1.67 |
3.18 |
Hg2+ |
2.09 |
2.02 |
2.12 |
2.09 |
1.95 |
3.30 |
Pb2+ |
1.81 |
1.67 |
1.74 |
1.71 |
1.67 |
3.28 |
Applicability of nanozyme sensor arrays
For further validation of the applicability of the nanozyme sensor array, we studied the practicability of distinguishing HMIs in real specimens. First, the response patterns of the selected HMIs in tap water were analyzed using LDA. As shown in Fig. 6A, the nanozyme sensor array showed a good separation ability for the five HMIs in tap water. Secondly, the color changes of the mixtures of Ag+ and Hg2+ with different ratios were measured and analyzed using LDA for the examination of the multiplex detection capability of the proposed nanozyme sensor array. As shown in Fig. 6B, the nanozyme sensor array exhibited a good separation ability and no overlapping for the five different concentrations in tap water (the same as in the case of DI water Fig. 3D). Our results revealed that our nanozyme sensor array successfully distinguished these HMIs in relatively complex media, specifying the capability of our developed nanozyme sensor array's practical application. Of interest, the same response manner as in Fig. S4† – when we used DI water – (slight enhancing in the case of citrate-Os, quenching in the case of citrate-Pt, and enhancing in the case of citrate-Au) can also be seen in Fig. S6† when we used tap water. In the same manner, SiYu lake water was used as a more complex example (Fig. S7†). This could clearly support the applicability and reliability of our developed nanozyme sensor array.
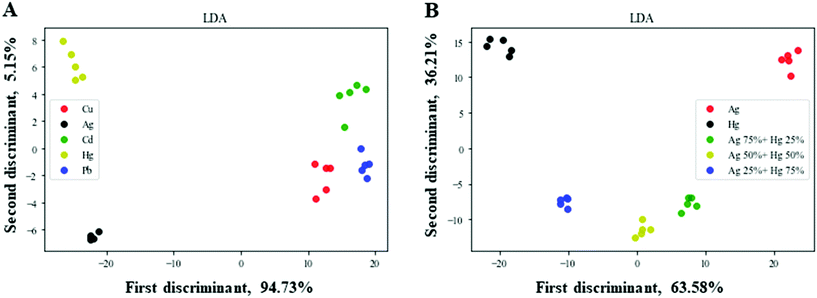 |
| Fig. 6 Nanozyme sensor array for HMI detection in tap water. Two-dimensional LDA score plots at, (A) 2.5 μM and (B) the mixtures of Ag+ and Hg2+ at various molar ratios. | |
Conclusion
In summary, a nanozyme sensor array consisting of three citrate-capped nanozymes with peroxidase-like activity was successfully constructed and designed to discriminate between five HMIs. The nanozymes’ catalytic activity was affected by the HMIs. Thus, the differential changes were monitored by the color change of the oxidized TMB, which gives a unique colorimetric pattern for every metal ion. So, the nanozyme sensor array showed a good separation capability towards the selected HMIs. Furthermore, the developed nanozyme sensor array was successfully used for the differentiation of the selected HMIs in an aqueous solution. Moreover, the developed nanozyme sensor array demonstrates a promising application for both semi-quantitative and quantitative analysis even at nanomolar concentrations. This study not only demonstrates an optical and sensitive approach for HMI discrimination but also increases the applicability of nanozymes in sensor arrays. However, further efforts are in high demand to construct more sensitive nanozyme sensing arrays for the detection of other toxic materials. Moreover, the enzymatic mimicry of nanozymes can be highly influenced by shape, size, and ligands. Therefore, further studies are required to prepare biosensor arrays using nanozymes with different sizes, shapes, and ligands.
Author contributions
H. A. A. N., idea, methodology, investigation, and writing; L. Y., and X. Y. G., reagent preparation; S. B. H., H. P. P., and H. H. D., idea; and W. C., idea, supervision, review and editing.
Conflicts of interest
No competing financial interest was declared.
Acknowledgements
The authors thankfully acknowledge the Program for Innovative Leading Talents in Fujian Province (2016B016), the Program for Innovative Research Team in Science and Technology in Fujian Province University (2018B033), and the Startup Fund for Scientific Research, Fujian Medical University (2018QH2016), and the financial support from the Joint Funds for the Innovation of Science and Technology, Fujian Province (2019Y9007).
Notes and references
- R. K. Sharma, M. Agrawal and F. Marshall, Ecotoxicol. Environ. Saf., 2007, 66, 258–266 CrossRef CAS PubMed.
- J. Briffa, E. Sinagra and R. Blundell, Heliyon, 2020, 6, e04691 CrossRef PubMed.
- P. B. Tchounwou, C. G. Yedjou, A. K. Patlolla and D. J. Sutton, Exper. Suppl., 2012, 101, 133–164 Search PubMed.
- L. Tan, Z. Chen, Y. Zhao, X. Wei, Y. Li, C. Zhang, X. Wei and X. Hu, Biosens. Bioelectron., 2016, 85, 414–421 CrossRef CAS PubMed.
- X. Zhou, J. Nie, J. Xu and B. Du, Colloid Polym. Sci., 2015, 293, 2101–2111 CrossRef CAS.
- L. Liu and H. Lin, Anal. Chem., 2014, 86, 8829–8834 CrossRef CAS PubMed.
- X.-P. Zhang, K.-Y. Huang, S.-B. He, H.-P. Peng, X.-H. Xia, W. Chen and H.-H. Deng, J. Hazard. Mater., 2021, 405, 124259 CrossRef CAS PubMed.
- G. Sener, L. Uzun and A. Denizli, ACS Appl. Mater. Interfaces, 2014, 6, 18395–18400 CrossRef CAS PubMed.
- Y. Safari, M. Karimaei, K. Sharafi, H. Arfaeinia, M. Moradi and N. Fattahi, J. Sci. Food Agric., 2018, 98, 2915–2924 CAS.
- H. Ebrahimi-Najafabadi, A. Pasdaran, R. R. Bezenjani and E. Bozorgzadeh, Food Chem., 2019, 289, 26–32 CrossRef CAS PubMed.
- S. L. Didukh-Shadrina, V. N. Losev, A. Samoilo, A. K. Trofimchuk and P. N. Nesterenko, Int. J. Anal. Chem., 2019, 2019, 1467631 Search PubMed.
- L. A. Hutton, G. D. O'Neil, T. L. Read, Z. J. Ayres, M. E. Newton and J. V. Macpherson, Anal. Chem., 2014, 86, 4566–4572 CrossRef CAS PubMed.
- F. B. Alkas, J. A. Shaban, A. A. Sukuroglu, M. A. Kurt, D. Battal and S. Saygi, Environ. Monit. Assess., 2017, 189, 1–8 CrossRef CAS PubMed.
- S.-B. He, L. Yang, M.-T. Lin, P. Balasubramanian, H.-P. Peng, Y. Kuang, H.-H. Deng and W. Chen, Biomed. Mater., 2020, 16, 032001 Search PubMed.
- L. Gao, J. Zhuang, L. Nie, J. Zhang, Y. Zhang, N. Gu, T. Wang, J. Feng, D. Yang and S. Perrett, Nat. Nanotechnol., 2007, 2, 577–583 CrossRef CAS PubMed.
- N. Singh, M. A. Savanur, S. Srivastava, P. D'Silva and G. Mugesh, Angew. Chem., Int. Ed., 2017, 56, 14267–14271 CrossRef CAS PubMed.
- Y. Zhang, F. Wang, C. Liu, Z. Wang, L. Kang, Y. Huang, K. Dong, J. Ren and X. Qu, ACS Nano, 2018, 12, 651–661 CrossRef CAS PubMed.
- X. Wang, Y. Hu and H. Wei, Inorg. Chem. Front., 2016, 3, 41–60 RSC.
- H. Wei and E. Wang, Anal. Chem., 2008, 80, 2250–2254 CrossRef CAS PubMed.
- K. Fan, C. Cao, Y. Pan, D. Lu, D. Yang, J. Feng, L. Song, M. Liang and X. Yan, Nat. Nanotechnol., 2012, 7, 459–464 CrossRef CAS PubMed.
- X. Wang, L. Qin, M. Zhou, Z. Lou and H. Wei, Anal. Chem., 2018, 90, 11696–11702 CrossRef CAS PubMed.
- J. Ko, N. Bhagwat, S. S. Yee, N. Ortiz, A. Sahmoud, T. Black, N. M. Aiello, L. McKenzie, M. O'Hara and C. Redlinger, ACS Nano, 2017, 11, 11182–11193 CrossRef CAS PubMed.
- W. Xu, C. Ren, C. L. Teoh, J. Peng, S. H. Gadre, H. W. Rhee, C. L. Lee and Y. T. Chang, Anal. Chem., 2014, 86, 8763–8769 CrossRef CAS PubMed.
- Z. Li, J. R. Askim and K. S. Suslick, Chem. Rev., 2018, 119, 231–292 CrossRef PubMed.
- Z. Pode, R. Peri-Naor, J. M. Georgeson, T. Ilani, V. Kiss, T. Unger, B. Markus, H. M. Barr, L. Motiei and D. Margulies, Nat. Nanotechnol., 2017, 12, 1161–1168 CrossRef CAS PubMed.
- S. Sun, K. Jiang, S. Qian, Y. Wang and H. Lin, Anal. Chem., 2017, 89, 5542–5548 CrossRef CAS PubMed.
- M. S. Hizir, N. M. Robertson, M. Balcioglu, E. Alp, M. Rana and M. V. Yigit, Chem. Sci., 2017, 8, 5735–5745 RSC.
- J. Karasinski, S. Andreescu, O. A. Sadik, B. Lavine and M. N. Vora, Anal. Chem., 2005, 77, 7941–7949 CrossRef CAS PubMed.
- S. N. Zulkifli, H. A. Rahim and W.-J. Lau, Sens. Actuators, B, 2018, 255, 2657–2689 CrossRef CAS PubMed.
- A. Mortari and L. Lorenzelli, Biosens. Bioelectron., 2014, 60, 8–21 CrossRef CAS PubMed.
- Y. Ma, Y. Li, K. Ma and Z. Wang, Sci. China: Chem., 2018, 61, 643–655 CrossRef CAS.
- S. He, L. Yang, P. Balasubramanian, S. Li, H. Peng, Y. Kuang, H. Deng and W. Chen, J. Mater. Chem. A, 2020, 8, 25226–25234 RSC.
- H.-H. Deng, G.-L. Hong, F.-L. Lin, A.-L. Liu, X.-H. Xia and W. Chen, Anal. Chim. Acta, 2016, 915, 74–80 CrossRef CAS PubMed.
- X. J. Chen, B. L. Sanchez-Gaytan, Z. Qian and S. J. Park, Wiley Interdiscip. Rev.: Nanomed. Nanobiotechnol., 2012, 4, 273–290 CAS.
- T. K. Sharma, R. Ramanathan, P. Weerathunge, M. Mohammadtaheri, H. K. Daima, R. Shukla and V. Bansal, Biosens. Bioelectron., 2014, 50, 15856–15859 CAS.
- M. Liu, H. Zhao, S. Chen, H. Yu and X. Quan, ACS Nano, 2012, 6, 3142–3151 CrossRef CAS PubMed.
- Y. Tao, Y. Lin, J. Ren and X. Qu, Biosens. Bioelectron., 2013, 42, 41–46 CrossRef CAS PubMed.
- Y. Zhu, J. Wu, L. Han, X. Wang, W. Li, H. Guo and H. Wei, Anal. Chem., 2020, 92, 7444–7452 CrossRef CAS PubMed.
- C.-I. Wang, C.-C. Huang, Y.-W. Lin, W.-T. Chen and H.-T. Chang, Anal. Chim. Acta, 2012, 745, 124–130 CrossRef CAS PubMed.
- G.-W. Wu, S.-B. He, H.-P. Peng, H.-H. Deng, A.-L. Liu, X.-H. Lin, X.-H. Xia and W. Chen, Anal. Chem., 2014, 86, 10955–10960 CrossRef CAS PubMed.
- B. Unnikrishnan, C.-W. Lien, H.-W. Chu and C.-C. Huang, J. Hazard. Mater., 2020, 401, 123397 CrossRef PubMed.
- B. Liu and J. Liu, Nano Res., 2017, 10, 1125–1148 CrossRef CAS.
- R. Zhu, Y. Zhou, X. L. Wang, L. P. Liang, Y. J. Long, Q. L. Wang, H. J. Zhang, X. X. Huang and H. Z. Zheng, Talanta, 2013, 117, 127–132 CrossRef CAS PubMed.
- D. Zhang, Z. Chen, H. Omar, L. Deng and N. M. Khashab, ACS Appl. Mater. Interfaces, 2015, 7, 4589–4594 CrossRef CAS PubMed.
- Y. J. Long, Y. F. Li, Y. Liu, J. J. Zheng, J. Tang and C. Z. Huang, Chem. Commun., 2011, 47, 11939–11941 RSC.
- C.-I. Wang, C.-C. Huang, Y.-W. Lin, W.-T. Chen, H.-T. Chen and J. A. c. a. Chang, Anal. Chim. Acta, 2012, 745, 124–130 CrossRef CAS PubMed.
- C.-L. Hsu, C.-W. Lien, C.-W. Wang, S. G. Harroun, C.-C. Huang and H.-T. Chang, Biosens. Bioelectron., 2016, 75, 181–187 CrossRef CAS PubMed.
- C.-L. Hsu, C.-W. Lien, S. G. Harroun, R. Ravindranath, H.-T. Chang, J.-Y. Mao and C.-C. Huang, Mater. Chem. Front., 2017, 1, 893–899 RSC.
- C.-F. Peng, Y.-Y. Zhang, L.-Y. Wang, Z.-Y. Jin and G. Shao, Anal. Methods, 2017, 9, 4363–4370 RSC.
- A. J. Kora and L. Rastogi, Sens. Actuators, B, 2018, 254, 690–700 CrossRef CAS.
- H. Deng, S. He, X. Lin, L. Yang, Z. Lin, R. Chen, H. Peng and W. Chen, Chin. Chem. Lett., 2019, 30, 1659–1662 CrossRef CAS.
- L. Zhang, G.-P. Yang, S.-J. Xiao, Q.-G. Tan, Q.-Q. Zheng, R.-P. Liang and J.-D. Qiu, Small, 2021, 2102944 CrossRef CAS PubMed.
- S.-H. Wen, X.-L. Zhong, Y.-D. Wu, R.-P. Liang, L. Zhang and J.-D. Qiu, Anal. Chem., 2019, 91, 6487–6497 CrossRef CAS PubMed.
- Y.-W. Wang, M. Wang, L. Wang, H. Xu, S. Tang, H.-H. Yang, L. Zhang and H. Song, Sensors, 2017, 17, 2521 CrossRef PubMed.
- W.-R. Cui, C.-R. Zhang, W. Jiang, R.-P. Liang, S.-H. Wen, D. Peng and J.-D. Qiu, ACS Sustainable Chem. Eng., 2019, 7, 9408–9415 CrossRef CAS.
- D. Jiang, D. Ni, Z. T. Rosenkrans, P. Huang, X. Yan and W. Cai, Chem. Soc. Rev., 2019, 48, 3683–3704 RSC.
- Y. Huang, J. Ren and X. Qu, Chem. Rev., 2019, 119, 4357–4412 CrossRef CAS PubMed.
- M. Liang and X. Yan, Acc. Chem. Res., 2019, 52, 2190–2200 CrossRef CAS PubMed.
- M. M. Bordbar, J. Tashkhourian, A. Tavassoli, E. Bahramali and B. Hemmateenejad, Sens. Actuators, B, 2020, 319, 128262 CrossRef CAS.
- H. Sharifi, J. Tashkhourian and B. Hemmateenejad, Sens. Actuators, B, 2021, 339, 129911 CrossRef CAS.
- L. Zhang, Y.-M. Zhang, R.-P. Liang and J.-D. Qiu, J. Phys. Chem. C, 2013, 117, 12352–12357 CrossRef CAS.
- Y.-M. Zhang, L. Zhang, R.-P. Liang and J.-D. Qiu, Chem. – Eur. J., 2013, 19, 6961–6965 CrossRef CAS PubMed.
- W. Gao, L. Zhang, R.-P. Liang and J.-D. Qiu, Chem. – Eur. J., 2015, 21, 15272–15279 CrossRef CAS PubMed.
Footnote |
† Electronic supplementary information (ESI) available. See DOI: 10.1039/d1an01895g |
|
This journal is © The Royal Society of Chemistry 2022 |