DOI:
10.1039/D1FO03003E
(Paper)
Food Funct., 2022,
13, 143-160
Dendrobium fimbriatum Hook polysaccharide ameliorates dextran-sodium-sulfate-induced colitis in mice via improving intestinal barrier function, modulating intestinal microbiota, and reducing oxidative stress and inflammatory responses†
Received
8th September 2021
, Accepted 8th November 2021
First published on 11th November 2021
Abstract
The ameliorative effect of Dendrobium fimbriatum polysaccharide (cDFPW1) on ulcerative colitis (UC) was investigated using a dextran-sodium-sulfate-induced (DSS-induced) mouse model in the present study. The results showed that cDFPW1 effectively improved colitis in mice by ameliorating weight loss, disease activity index (DAI) and colonic pathological damage, and by protecting the intestinal barrier function integrity. Moreover, cDFPW1 modulated the composition and metabolism of intestinal microbiota through enhancing Romboutsia, Lactobacillus and Odoribacter, and reducing Parasutterella, Burkholderia-Caballeronia-Paraburkholderia and Acinetobacter in colitis mice. Notably, cDFPW1 significantly restored the homeostasis of Th17/regulatory T (Treg) cells and the expression of specific cytokines. Western blotting of colon tissues showed that cDFPW1 markedly up-regulated the expression of Nrf2 and inhibited the phosphorylation of NF-κB signaling. These results indicated that cDFPW1 possesses the potential of improving UC and its effect on palliating colitis may be connected with the regulation of Nrf2/NF-κB signaling.
1. Introduction
Ulcerative colitis (UC) is a chronic disease that leads to inflammation and ulcers in the colon and rectum. The main symptoms of its attack include abdominal pain, diarrhea, and weight loss.1 The cause of UC is likely to be the combination of environmental factors and genetic susceptibility, which causes the disintegration of the intestinal epithelial barrier and the maladjustment of the immunoreaction to intestinal flora.2,3 Under the conditions of UC, the down-regulated expression of the tight junction protein will bring about an increase in the permeability of the intestine to microbial ligands and harmful metabolites, which ultimately triggers systemic inflammation.4 Meanwhile, inflammatory cytokines seem to play a critical role in controlling clinical symptoms related to the pathogenesis of UC.5 During the active stage of UC, proinflammatory cytokines further produce oxidative products to change the redox balance in the intestinal mucosa and induce redox sensitive transcription factors to aggravate inflammation and ultimately damage the intestinal barrier.6 Numerous studies in recent years have shown that the imbalance of intestinal microbes and their metabolites in patients with inflammatory bowel disease (IBD) can trigger an abnormal T cell immune response in individuals with susceptible genes, resulting in excessive activation of effector T cell subsets or deficiency of regulatory T cells (Treg), thereby causing a series of serious diseases such as gastrointestinal diseases, persistent immune disorders and metabolic syndrome.7–9
Wirtz et al. induced an animal model of acute colitis by administering DSS to study UC.10 This animal model mimics the clinical symptoms and morphology of UC patients, which contributes to designing a reasonable treatment plan for UC.11 The traditional methods for the treatment of UC are to inhibit inflammation responses through immunosuppressants, immunomodulators, and biological agents.12 However, the long-term use of them may cause substantial side-effects, for example increased susceptibility to secondary infections and cancer.13,14 As a result, it is significant to develop safe and valid therapeutic agents to prevent and mitigate UC.
Natural compounds are showing attractive potential in the prevention and intervention of UC, and polysaccharides have been paid more and more attention due to their significant efficacy and fewer side-effects.15,16 Increasing research suggested that polysaccharides, as prebiotics to regulate the gut microbiota, not only have good anti-inflammatory activity,17 but also can reach the large intestine safely and interact with the gut microbiota.18,19Dendrobium fimbriatum Hook is one of the Dendrobium species recorded in Chinese Pharmacopoeia with the functions of nourishing Yin, clearing heat, promoting saliva and protecting the stomach.20 As one of the principal active components, D. fimbriatum polysaccharides exhibit a variety of biological activities, such as immunoregulatory, hypoglycemic and antioxidative effects,21,22 and the structure of one homogeneous polysaccharide (cDFPW1) from its stems has been well characterized recently.20 The present study used DSS-induced colitis model mice to further investigate the improving effect of cDFPW1 on the pathological progress of UC through analyzing the changes in colitis symptoms, intestinal barrier function, intestinal microbes and their metabolites, immune responses, and oxidative damage as well as the related signaling pathways.
2. Materials and methods
2.1 Chemicals and reagents
D. fimbriatum stems were from Baise City, Guangxi Province. Dextran sulfate sodium (DSS) (Mw 36–50 kDa) was obtained from MP Biomedicals (Irvine, CA, USA). DEAE-52 cellulose, Sephadex G-75 and FITC-dextran (Mw 4 kDa) were obtained from Sigma-Aldrich (St Louis, MO, USA). Kits for the determination of myeloperoxidase (MPO), diamine oxidase (DAO), superoxide dismutase (SOD), malondialdehyde (MDA), glutathione peroxidase (GSH-Px), and catalase (CAT) were obtained from the Jiancheng Bioengineering Institute of Nanjing (Nanjing, China). A colorimetric D-lactate assay kit was purchased from AAT Bioquest (Sunnyvale, CA, USA). A chromogenic end-point tachypleus amebocyte lysate (TAL) assay kit was obtained from Chinese Horseshoe Crab Reagent Manufactory Co., Ltd (Xiamen, China). A RNA easy™ Animal RNA Isolation Kit was provided by Beyotime Biotechnology (Shanghai, China). A Revert Aid first strand cDNA synthesis kit was from Thermo Fisher Scientific (Waltham, MA, USA) and the iTaq™ Universal SYBR@ Green Supermix kit was obtained from Bio-Rad Laboratories (Hercules, CA, USA). Primers and a BCA protein assay kit were provided by Sangon Biotech Co., Ltd (Shanghai, China). A mouse cytokine array kit (QAM-TH17-1) was obtained from RayBiotech Inc. (Norcross, GA, USA). Immobilon-P polyvinylidene fluoride (PVDF) membranes (size: 0.45 μm) were obtained from Merck Millipore, Ltd (Billerica, MA, USA). Antibodies against ZO-1, Occludin, nuclear factor E2-related factor 2 (Nrf2), Keap-1 (kelch-like ECH-associated protein-1), IκB, phosphor-IκB, NF-κB p65, phosphor-NF-κB p65 and β-actin were purchased from Cell Signaling Technology (Cell Signaling Technology, Beverly, MA, USA). Horseradish peroxidase-conjugated goat anti-rabbit IgG was obtained from eBioscience (Beverly, MA, USA). Other antibodies for flow analysis included anti-CD16/32, anti-CD25-FITC and anti-Foxp3-APC antibodies from BioLegend (San Diego, CA, USA), anti-CD4-PE from BD Pharmingen (San Jose, CA, USA), and anti-CD4-PerCp-Cy5.5, anti-IFN-γ, anti-IL-4-APC and anti-IL-17A-PE from Elabscience Biotechnology Co. Ltd (Wuhan, China). An E.Z.N.A. Soil DNA kit was provided by Omega Bio-tek (Norcross, GA, USA). Acetic acid, propionic acid, n-butyric acid, n-valeric acid, i-butyric acid and i-valeric acid were obtained from Aladdin Reagent Int. (Shanghai, China). All other reagents were purchased from Sinopharm Chemical Reagent Co., Ltd (Shanghai, China).
2.2 Polysaccharide preparation
cDFPW1 was prepared from the stems of D. fimbriatum as our previous study.20 In short, the dried stem powder was pre-extracted with 95% ethanol (1
:
40, g
:
mL) at 25 °C for 24 h, and stirred then with distilled water at 80 °C for 2 h. The extract was precipitated with ethanol at a final concentration of 80% (v/v) for 24 h followed by protein removal using the Sevag method. The alcohol-insoluble substances were freeze-dried for 48 h to obtain crude polysaccharide, which was further eluted on a DEAE-Cellulose-52 column (1.6 cm × 60 cm) and a Sephadex G-75 column (1.6 cm × 80 cm) with pure water to give D. fimbriatum polysaccharide cDFPW1. The structural characteristics of cDFPW1 were analyzed by HPGPC, FT-IR, and methylation analysis as well as NMR analysis, which showed that cDFPW1 was a homogeneous polysaccharide with a relative molecular weight of 4.0 × 104 Da and consisted of T-linked Manp, 1,4-linked Glcp, 1,4-linked Manp, and 1,4,6-linked Glcp in a molar ratio of 1.3
:
7.1
:
1.0
:
1.9 (Fig. S1†).
2.3 Animal experiments and design
Six-week-old C57BL/6 male mice (No. 410983201100165078) were obtained from Skbex Biotechnology Co. Ltd (Henan, China) and kept in an environment without pathogen under the condition of a 12 h light–dark cycle at 25 ± 2 °C. Mice were provided with distilled water and food ad libitum. All animal procedures were performed in accordance with the Regulations for Care and Use of Laboratory Animals of the People's Republic of China and experiments were approved by the Animal Ethics Committee of Hefei University of Technology. After one week of acclimation, the mice were randomly divided into five groups (n = 20). The experimental procedure is shown in Fig. 1A. Group I (Control group) was administered distilled water for 21 days. Group II (DSS) was administered distilled water for the first 14 days, and then was fed with 3% (w/v) DSS in distilled water from day 14 to day 21. Group III (L-cDFPW1), Group IV (M-cDFPW1) and Group V (H-cDFPW1) were given oral administration with different doses of cDFPW1 (50 mg kg−1 day−1, 100 mg kg−1 day−1, and 200 mg kg−1 day−1, respectively) once per day for 21 days, along with the oral administration of 3% (w/v) DSS in distilled water from day 14 to day 21 for 7 days. For all groups, the halves of the mice were euthanized on the 14th day to observe the effects of cDFPW1 on the normal mice, and the remaining mice were euthanized on the 21th day to detect the improved effect of cDFPW1 on the colitis mice.
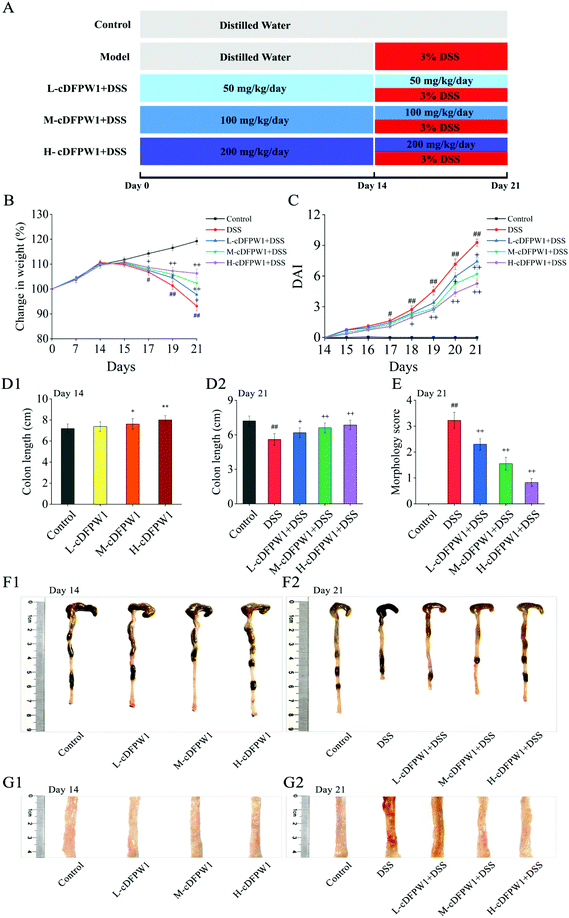 |
| Fig. 1 cDFPW1 attenuated the colitis symptoms in mice. Experimental design (A), changes in body weight (B), DAI score (C), colon length (D1 and D2), morphology score (E), representative image of colon tissue in each group (F1 and F2), and representative section of colon tissue in each group (G1 and G2). Data are presented as mean ± SEM (n = 10). *p < 0.05 and **p < 0.01 vs. control group on day 14. +p < 0.05 and ++p < 0.01 vs. DSS-induced model group. #p < 0.05 and ##p < 0.01 vs. control group on day 21. | |
2.4 Assessment of colitis
An electronic analytical balance was used to measure and record the body weights of mice on day 0, 7, 14 and 21, respectively. During DSS treatment, the changes of the disease activity index (DAI) of mice were recorded according to the literature's method10 (Table S1†). The entire colon was removed from each dissected mouse for the measurement of its length. The degree of mucosal damage of colons was scored with reference to the literature's method.23 For transmission electron microscopy (TEM) analysis, the small pieces of colon tissue specimens were placed rapidly into glutaraldehyde at 25 ± 2 °C for 2 h and then fixed in osmium tetroxide. The specimens were dehydrated in ethanol and acetone with degrading concentrations, embedded in epon, and dried in an oven at 60 °C for 24 h. After cutting with a glass knife and diamond knife successively, the thin sections were placed on copper grids, impregnated with uranyl acetate and lead citrate, and then scanned through a Tecnai G2 Spirit transmission electron microscope (FEI Co., Hillsboro, OR, USA). For hematoxylin and eosin (H&E) analysis, the colon tissues were collected, dehydrated, embedded, sectioned and stained for observation and pathological scoring of colitis by referring to the method described previously24 (Table S2†). The number of goblet cells was studied by PAS staining.25 Neutrophil infiltration was assessed by Ly6G+ staining.26
2.5 Determination of MPO, D-lactic acid, DAO and endotoxin
Clean colon tissue of 100 mg was added to 1 mL tissue lysis buffer to homogenize and then centrifuged for 20 min (12
000g, 4 °C) to obtain the supernatant. The MPO activity of tissue was measured using a myeloperoxidase assay kit following the manufacturer's instruction. Blood was collected and serum was separated by centrifugation for 15 min (3500g, 4 °C). The contents of endotoxin, DAO and D-lactic acid in serum were tested using the corresponding ELISA kits.
2.6
In vivo intestinal permeability assay
The intestinal permeability of mice was evaluated by FITC-dextran.27 The mice received intragastric administration of FITC-dextran to track intestinal permeability at 60 mg per 100 g body weight. The serum was harvested after 4 h of intragastric administration, and the concentration of FITC-dextran in serum was detected using a Synergy H1 microplate reader (BioTek, Winooski, VT, USA).
2.7 Quantitative real-time PCR
Total RNA of the colon tissues was extracted and purified in accordance with the manufacturer's instructions by using a RNAeasy™ Animal RNA Isolation kit. The concentration of RNA was detected using a NanoDrop 2000 UV-vis spectrophotometer (Thermo Scientific, Wilmington, DE, USA). Subsequently, cDNA was synthesized and amplified through using the Revertaid First Strand cDNA Synthesis kit, iTaq™ Universal SYBR@ Green Supermix kit and specific primers. RT-PCR was performed using a BIO-RAD MyIQ2 real-time PCR System (Hercules, CA, USA), and the conditions of amplification were as follows: an initial denaturation of 1 min at 94 °C, followed by 40 cycles of 95 °C for 10 s, annealing at 60 °C for 15 s and extending at 72 °C for 6 s. The relative mRNA expression of Occludin and ZO-1 was calculated by the 2−ΔΔCt method. Specific primers for target genes are shown in Table S3.† The glyceraldehyde-3-phosphate dehydrogenase (GAPDH) was used as the internal control for normalization.
2.8 Western blot analysis
Western blot was performed according to the method we described previously.28 Protein samples were prepared, subjected to 8–12% SDS-PAGE, and then transferred to the PVDF membrane, which was probed with the corresponding primary antibodies against Occludin, ZO-1, Nrf2, Keap-1, NF-κB p65, phosphor-NF-κB p65, IκB, phosphor-IκB, and β-actin (all at 1
:
1000 dilution) at 4 °C overnight, and then incubated with 1
:
5000 for goat anti-rabbit IgG-HRP at 25 ± 2 °C for 2 h. The stained membranes were photographed on ImageQuant LAS 4000 mini (GE Healthcare, NJ, USA) and quantified through employing the Alpha View SA 3.3.0.0 software (San Jose, CA, USA).
2.9 Short-chain fatty acid analysis
The SCFA contents were determined according to the approaches previously reported.29 The feces were added to twice the volume of ultrapure water and vortexed to obtain homogenate. The supernatant following centrifugation for 10 min (12
000g, 4 °C) was determined through an Agilent gas chromatograph (Agilent Technologies Inc., USA) equipped with a silica capillary column (DB-Wax, J&W 30 m × 0.25 mm I.D.).
2.10 Intestinal microflora analysis
Microbial DNA was extracted from colon contents using the E.Z.N.A.® Soil DNA kit following the manufacturer's protocol. The concentration and purity of DNA were measured using a NanoDrop 2000 UV-vis spectrophotometer (Thermo Scientific, Wilmington, DE, USA). The V3–V4 region of the 16S ribosomal RNA (rRNA) gene was amplified by PCR with the primer 338 F (5′ACTCCTACGGGAGGCAGCAG-3′) and 806 R (5′-GGACTACHVGGGTWTCTAAT-3′) according to the previous protocol.30 Subsequently, sequencing was implemented by using the Illumina Miseq sequencing technology (Illumina, San Diego, CA, USA) and further processed with the free online platform of Majorbio Cloud Platform (http://www.majorbio.com).
2.11 Flow cytometry analysis
Lamina propria cells (LPCs) were prepared by consulting the method of Xie.29 In brief, the intestinal cavity was cut into 0.5 cm fragments after rinsing the contents with HBSS. Then, the fragments were immersed in HBSS digestive solution containing 5 mM EDTA and 4% serum and digested at 37 °C on a shaker with 250 rpm for 20 min to release the epithelial cells. Afterwards, LPCs were isolated from the intestine pieces through the treatment of RPMI1640 medium digestion solution containing 4% FBS, collagenase-D (0.5 mg mL−1), DNase I (0.5 mg mL−1) and Dispase (1 mg mL−1) for 20 min at 37 °C. The concentration of LPCs was adjusted to 1 × 106 cells per mL with PBS-5% FBS and incubated with anti-CD4-PerCp-Cy5.5, anti-IFN-γ-FITC, anti-IL-4-APC and anti-IL-17A-PE to analyze Th1, Th2 and Th17 cells, and with anti-CD4-PE, anti-CD25-FITC and anti-Foxp3-APC to analyze regulatory T (Treg) cells using a 14-color BD LSR Fortessa flow cytometer (BD Biosciences, San Jose, CA, USA).
2.12 Cytokine assays
The colon tissue of 100 mg was homogenized with cell lysis buffer and protease inhibitor cocktail on ice for 30 min with a shake per 5 min to obtain tissue homogenate, which was centrifuged at 16
000g and 4 °C for 20 min to obtain the supernatant. The BCA protein assay kit was used to determine the protein concentration in the supernatant. Cytokines in the supernatant samples for each mouse in each group were analyzed using a mouse cytokine array kit (QAM-TH17-1) according to the protocol provided by the manufacturer. After visualizing the signals using the InnoScan 300 Microarray Scanner (Innopsys, Parcd'ActivitésActivestre, Carbonne, France) equipped with a Cy3 wavelength (green channel, excitation 532 nm), the results were calculated using the RayBio Analysis Tool Excel sheet.
2.13 Evaluation of oxidative stress
SOD, MDA, GSH-Px and CAT activities in the colon tissue were detected using a Bio-Rad model 680 Microplate Reader (Hercules, CA, USA) according to the instruction of the commercial kits.
2.14 Data analysis
Most of the data and figures were statistically analyzed and made using SPSS 19.0 statistics software (SPSS Inc., Chicago, IL, USA) and Origin 8.0, respectively. For multiple groups, one-way ANOVA followed by Duncan's test was performed for analyzing the statistical differences. For the data of gut microbiome, we used the online platform of the Majorbio Cloud. Alpha diversity analysis was performed by the Wilcoxon rank-sum test. The PCoA plots were produced with weighted UniFrac distances. Kruskal–Wallis rank sum tests were performed to analyze species difference among groups, and then Tukey Kramer post-hoc tests were performed to compare between each two groups. All results were represented as mean ± SEM. p < 0.05 or p < 0.01 was considered significant between groups.
3. Results
3.1 cDFPW1 alleviated the colitis symptoms
In order to investigate the relieving effects of oral cDFPW1 on colitis, mice were first treated with distilled water or cDFPW1 of different doses for 14 days, and then treated with distilled water, DSS, and cDFPW1 + DSS for another 7 days, as designed in Fig. 1A. Compared with the control group, there was no significant difference in body weight and colon tissue mucosal structure after oral administration of cDFPW1 (50, 100 and 200 mg kg−1) for 14 days (Fig. 1B, C and G1), but the cDFPW1 in the middle and high dose groups increased the colon length of mice (Fig. 1D1 and F1). After 21 days, the DSS treatment alone significantly caused body weight loss (Fig. 1B), DAI score increases (Fig. 1C) and colon length shortness (Fig. 1D2 and F2) in model mice. Meanwhile, the morphological score was also significantly increased, and the appearance of colonic mucosa was rough and uneven, showing the fine granule accompanied by reddish-brown bleeding and mucosal shedding (Fig. 1E and G2). However, cDFPW1 supplementation effectively improved the trend of body weight loss, inhibited the elevation of DAI score, prevented the bleeding and shortening of colon, decreased the morphology score, and obviously alleviated the DSS-induced colitis symptoms. These results indicated that cDFPW1 supplementation may help to prevent the occurrence and development of UC.
3.2 cDFPW1 ameliorated the colitis histopathological features
The ultrastructure of the microvilli (Mv) and tight junctions (TJs) of intestinal epithelium in colon were observed by TEM. After intragastric administration of cDFPW1 for 14 days, the Mv of epithelial cells were arranged neatly, the tips were visible, and TJs (shown by the arrows) were intact in all groups (Fig. 2A1). After 21 days, the Mv of colon were sparse and shortened, the TJs were destroyed, and the intercellular space was expanded from DSS treatment, but cDFPW1 supplementation weakened the destroyed ultrastructure in UC (Fig. 2A2). As compared to the control, HE staining showed no significant changes in the histopathological structure along with the infiltration of neutrophils (Ly6G+) in the mice given with cDFPW1 alone, while the number of goblet cells was observed to increase in a dose-dependent manner (Fig. 2B1–D1). It means that cDFPW1 will not only cause no damage to the intestine, but may also play an important role in maintaining the integrity of the intestinal mucosa. In the model group, there were severe mucosal damage, deformation of crypts and loss of goblet cells in colon tissue accompanied by neutrophil Ly6G+ infiltration from DSS treatment. In contrast, these DSS-induced histopathological changes were significantly improved after cDFPW1 intervention (Fig. 2B2–D2 and Fig. S2†). These results confirmed that cDFPW1 supplementation had a protective effect on the intestinal tract and reduced DSS-induced colon tissue damage.
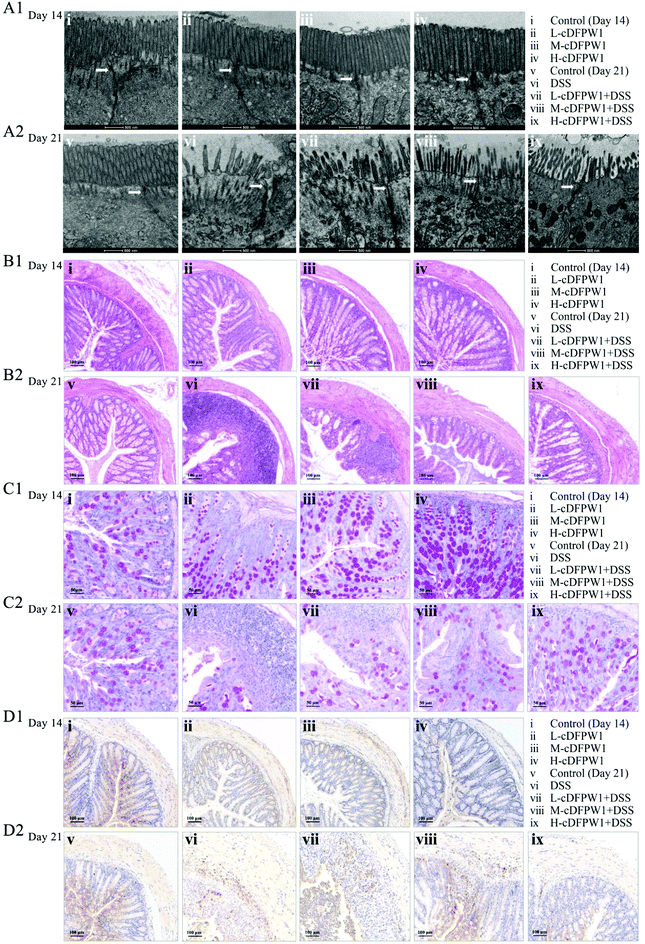 |
| Fig. 2 cDFPW1 attenuated the colon tissue damage in DSS-induced colitis mice. TEM observation of intestinal epithelial tissues (scale bar, 500 nm) and the arrow points to TJs (A1 and A2), hematoxylin and eosin (H&E) staining of the colon (scale bar, 100 μm) (B1 and B2), PAS staining of the colon (scale bar, 50 μm) (C1 and C2), and immunohistochemical staining of Ly6G+ positive cells in colon tissues (scale bar, 100 μm) (D1 and D2). | |
3.3 cDFPW1 protected the integrity of intestinal barrier function
After supplementing with cDFPW1 for 14 days, the protein and mRNA expression levels of Occludin and ZO-1 in the colon tissue of normal mice were significantly upregulated in a dose-dependent manner (Fig. 3A1–C1). In the meantime, the levels of endotoxin, DAO and D-lactic acid in serum and MPO content in colon tissue were down-regulated effectively by oral cDFPW1 (Fig. 3D1–G1). DSS administration from day 14 significantly decreased the expression level of TJ proteins Occludin and ZO-1 (Fig. 3A2–C2), which was accompanied by the obvious increase both in endotoxin, DAO, and D-lactic acid contents of the serum and in MPO levels of colon tissue (Fig. 3D2–G2). However, all these DSS-induced changes in mice could gradually return to the normal level by cDFPW1 administration. It is worth noting that the FITC-dextran permeability experiment showed that cDFPW1 has no significant effect on the intestinal mucosal permeability of normal mice (Fig. 3H1), but it could decelerate the intestinal barrier dysfunction in DSS-induced mice (Fig. 3H2). These results potentially indicated that cDFPW1 contributed to enhance the structural integrity and barrier function of the intestinal mucosa, and prevent the intestinal barrier damage caused by colitis mice.
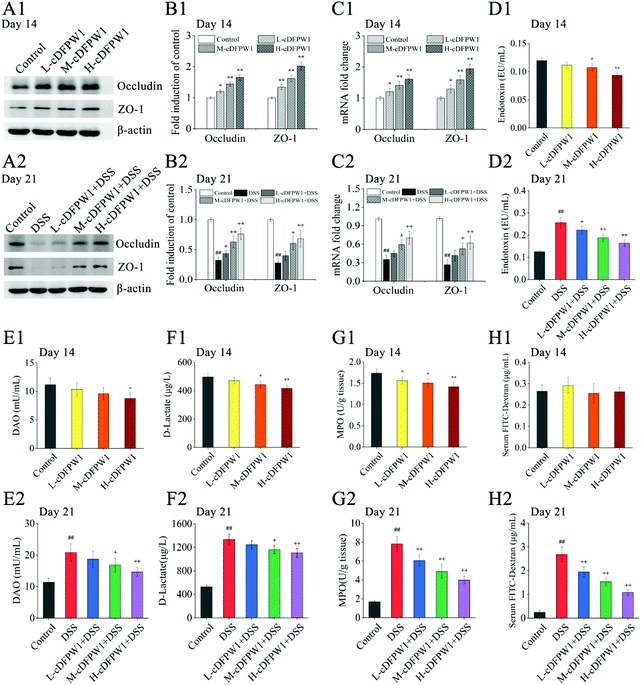 |
| Fig. 3 cDFPW1 strengthened colon epithelial barrier integrity in DSS-induced colitis mice. Western blot of Occludin and ZO-1 (A1 and A2), statistical analysis of western blot (B1 and B2), RT-qPCR analysis of Occludin and ZO-1 (C1 and C2), serum endotoxin (D1 and D2), serum DAO (E1 and E2), serum D-lactate (F1 and F2). MPO in colon tissue (G1 and G2), and serum FITC-dextran (4 kDa) (H1 and H2). Data are presented as mean ± SEM (n = 3). *p < 0.05 and **p < 0.01 vs. control group on day 14. +p < 0.05 and ++p < 0.01 vs. DSS-induced model group. #p < 0.05 and ##p < 0.01 vs. control group on day 21. | |
3.4 cDFPW1 promoted the production of short-chain fatty acids
Analysis of SCFAs in the feces of mice showed that the levels of acetic acid, propionic acid and n-butyric acid were significantly increased by oral cDFPW1 for 14 days as compared with the control group, while the contents of i-butyric acid, n-valeric acid and i-valeric acid had no obvious difference between groups (Fig. 4A1–F1). After 21 days, the levels of acetic acid, propionic acid, n-butyric acid, i-butyric acid and i-valeric acid in the feces of the DSS model group were significantly lower than those in the control group (p < 0.05). However, the decreased levels of acetic acid, propionic acid, and n-butyric acid by DSS could be significantly increased by cDFPW1 intervention (Fig. 4A2–F2). Additionally, the concentration of acetic acid in the H-cDFPW1 + DSS group on day 21 was higher than that in the control group (Fig. 4A2). The results above suggested that cDFPW1 supplementation could regulate the production of SCFAs.
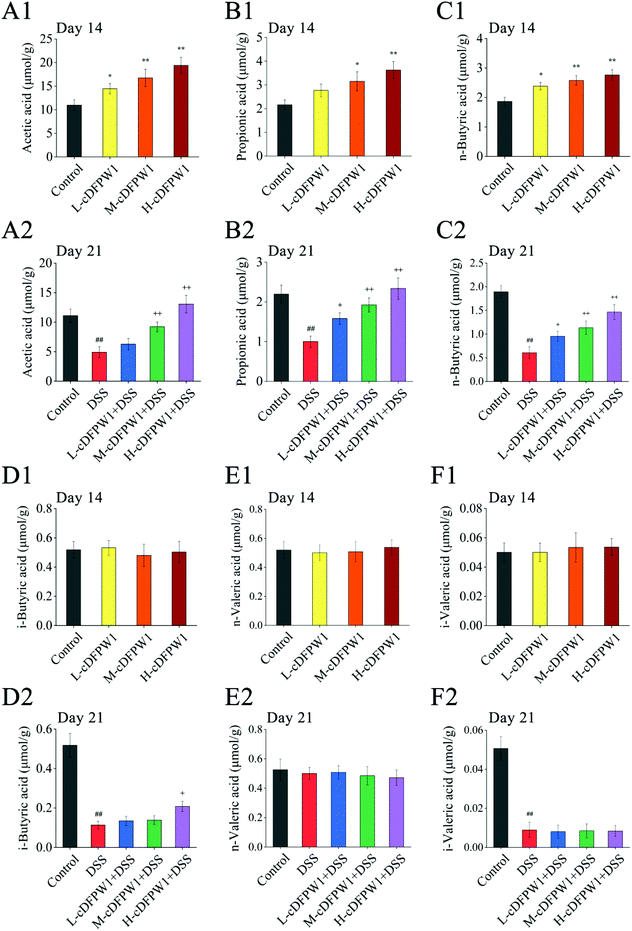 |
| Fig. 4 cDFPW1 regulated the amount of SCFAs in feces from DSS-induced colitis mice. Acetic acid (A1 and A2), propionic acid (B1 and B2), n-butyric acid (C1 and C2), i-butyric acid (D1 and D2), n-valeric acid (E1 and E2) and i-valeric acid (F1 and F2). Data are presented as mean ± SEM (n = 3). *p < 0.05 and **p < 0.01 vs. control group on day 14. +p < 0.05 and ++p < 0.01 vs. DSS-induced model group. #p < 0.05 and ##p < 0.01 vs. control group on day 21. | |
3.5 cDFPW1 modulated the intestinal microbiota in colitis mice
The α-diversity of intestinal microbes was evaluated by the index of Simpson, Shannon and Chao. After oral administration of cDFPW1 for 14 days, there was no significant difference between the control, L-cDFPW1, M-cDFPW1 and H-cDFPW1 groups (Fig. 5A1–C1). The total number of OTUs and overlapping OTUs in each group can be visually displayed by the Venn diagram. The results showed that there were 24, 40, 29 and 38 unique OTUs in the control group, L-cDFPW1, M-cDFPW1 and H-cDFPW1, respectively (Fig. 5D1). The principal coordinate analysis (PCoA) of Weighted UniFrac distance reflected the β-diversity of intestinal microflora between different groups. It is observed that the structures of the intestinal microflora of oral cDFPW1 were partially superimposed as compared to the control group, and showed a tendency to move to the second quadrant as the dose of cDFPW1 increased (Fig. 5E1). After 21 days, compared with the control group, DSS-treatment decreased the diversity of the gut microbiome structure, while cDFPW1 supplementation could reverse the results, and the H-cDFPW1 + DSS group was the most outstanding among all groups (Fig. 5A2–C2). As shown in Fig. 5D2, there were 19, 16, 18, 23 and 26 unique OTUs in the control, DSS, L-cDFPW1 + DSS, M-cDFPW1 + DSS and H-cDFPW1 + DSS, respectively. The intestinal microflora of mice in the control group and DSS group could be well distinguished. As the dose of cDFPW1 increased, the intestinal microflora of colitis mice exhibited a tendency to move to the control group (Fig. 5E2). The results manifested that cDFPW1 could prevent the changes of the intestinal microflora structure induced by DSS.
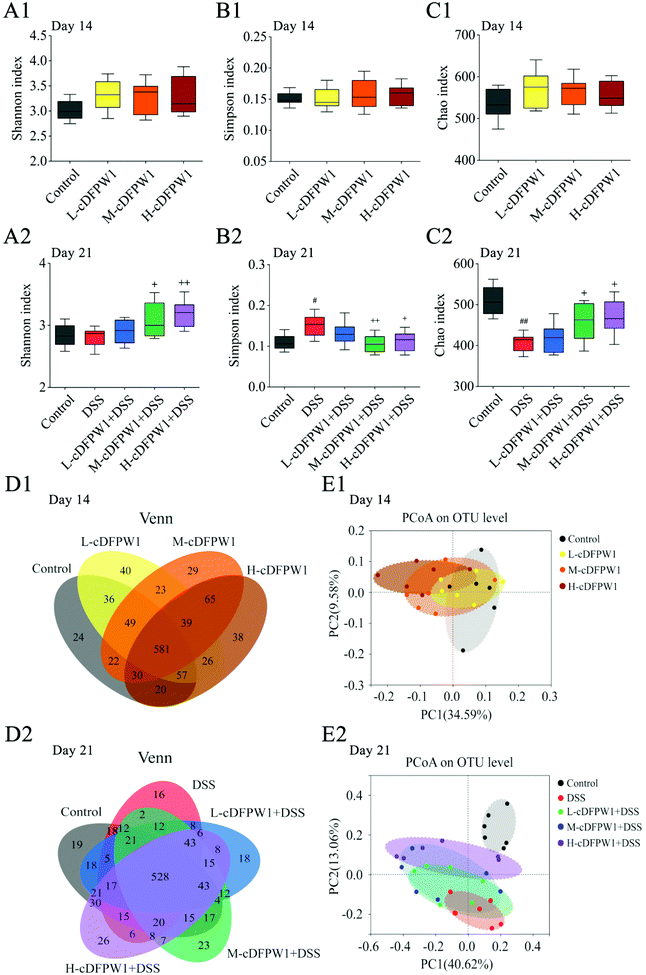 |
| Fig. 5 cDFPW1 regulated the intestinal microflora structure from DSS-induced colitis mice. Shannon index (A1 and A2), Simpson index (B1 and B2), Chao index (C1 and C2), Venn diagrams of OTUs (D1 and D2), and β-diversity assessed by PCoA analysis based on weighted UniFrac distances (E1 and E2). Data are presented as mean ± SEM (n = 6). *p < 0.05 and **p < 0.01 vs. control group on day 14. +p < 0.05 and ++p < 0.01 vs. DSS-induced model group. #p < 0.05 and ##p < 0.01 vs. control group on day 21. | |
To further statistically support the effects of cDFPW1 on the structure and composition of the gut microbiota, the Kruskal–Wallis H test followed by Tukey Kramer post-hoc tests was examined. As shown in Fig. 6A1, there were seven major phyla in the gut microbial communities at the first 14 days. Firmicutes and Verrucomicrobiota abundance increased but Bacteroidota and Campilobacterota abundance decreased in the cDFPW1 group as compared with the control group. At the genus level, it was revealed that as compared with the control group, cDFPW1 treatment remarkably increased the abundance of Lactobacillus, Ileibacterium, Allobaculum and Akkermansia. Meanwhile, the abundance of Lachnospiraceae_NK4A136_group, unclassified_f__Lachnospiraceae, Bacteroides and Helicobacter was significantly reduced (Fig. 6B1 and Fig. S3†). LEfSe used linear discriminant analysis (LDA) to reveal the biomarkers and dominant flora in each group (Fig. 6C1). Among all flora, Akkermansia was the dominant genus in the L-cDFPW1 group, and Lactobacillus was enriched in the M-cDFPW1 group, while Ileibacterium and Alistipes were the most abundant genus in the H-cDFPW1 group (Fig. 6D1). After 21 days, the histogram of the gut microbial community structure displayed the microbial species and their relative abundance at the phylum level (Fig. 6A2). Compared with the DSS group, Firmicutes and Verrucomicrobiota abundances were up-regulated, while Campilobacterot and Proteobacteria abundances were down-regulated by oral cDFPW1. In Fig. 6B2, the relative abundance of intestinal microbiota in the mice of five groups showed differences at the genus level. The relative abundance of Lachnospiraceae_NK4A136_group, Turicibacter, Bacteroides, Helicobacter, Parabacteroides in DSS-induced mice was notably improved, while supplementation of cDFPW1 reduced the relative abundance. Meanwhile, cDFPW1 treatment significantly increased the relative abundance of norank_f__Muribaculaceae, Ileibacterium, Romboutsia, Lactobacillus, Allobaculum, norank_f__norank_o__Clostridia_UCG-014 and Odoribacter (Fig. 6B2 and Fig. S4†). Dominant communities of 10, 17, 3, 5 and 7 taxa were found in the control, DSS, L-cDFPW1 + DSS, M-cDFPW1 + DSS and H-cDFPW1 + DSS groups, respectively (Fig. 6C2 and D2). The abovementioned results demonstrated that the Proteobacteria Phylum (Parasutterella, Burkholderia-Caballeronia-Paraburkholderia and Acinetobacter) were dominant bacteria in the model mice, while Romboutsia (L-cDFPW1 + DSS), Akkermansia (M-cDFPW1 + DSS), Odoribacter and Lactobacillus (H-cDFPW1 + DSS) were identified as dominant bacteria in DSS-induced mice treated with cDFPW1.
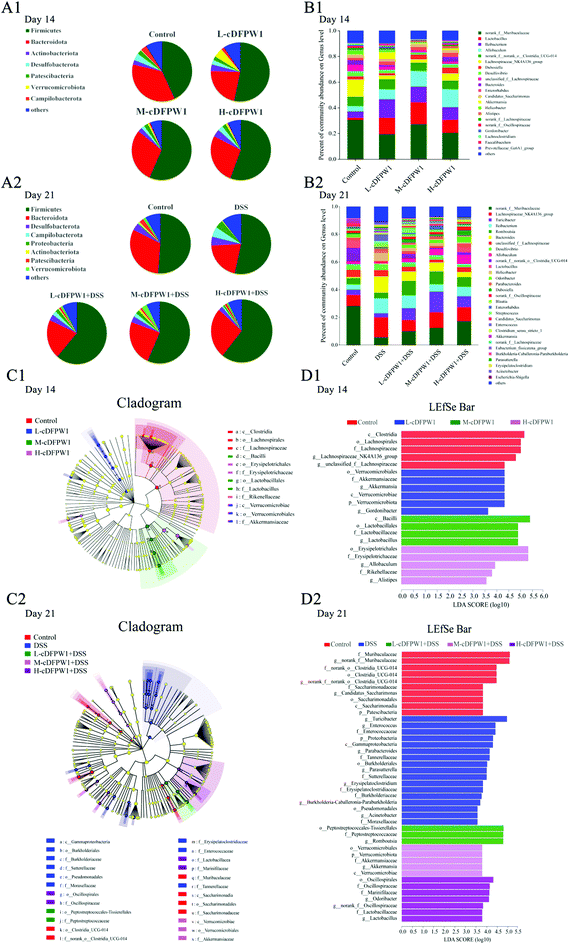 |
| Fig. 6 cDFPW1 reshaped the specific intestinal microflora from DSS-induced colitis mice. Microbiota compositions at the phylum level (A1 and A2), microbiota compositions at the genus level (B1 and B2), cladogram (C1 and C2), and distribution histogram based on a log LDA score above 3.5 (D1 and D2). Data are presented as mean ± SEM (n = 6). *p < 0.05 and **p < 0.01 vs. control group on day 14. +p < 0.05 and ++p < 0.01 vs. DSS-induced model group. #p < 0.05 and ##p < 0.01 vs. control group on day 21. | |
3.6 cDFPW1 recovered the balance of Th17/Treg cells in DSS-induced colitis mice
Phenotypic analyses of T cells in colonic LPCs were performed by flow cytometry. After oral administration of cDFPW1 for 14 days, no significant effect was found on the proportion of Th1, Th2, Th17 and Treg cells as compared with the control group (Fig. S5†). After 21 days, although cDFPW1 supplementation had no significant influence on the DSS-changed proportion of Th1 and Th2 cells (Fig. 7A and B), the proportion of Th17 cells was significantly reduced and the proportion of Treg cells was increased (Fig. 7C, D and E). Fig. 7F showed that with cDFPW1 administration of low, middle and high dosages, the ratio of Th17 cells to Treg cells in DSS-indued colitis mice could be decreased from 2.29 to 1.44, 0.81 and 0.47, respectively. These results suggested that cDFPW1 supplementation could improve the severity of DSS-induced colitis inflammation by restoring the immune homeostasis of Th17 and Treg cells in DSS-induced colitis mice.
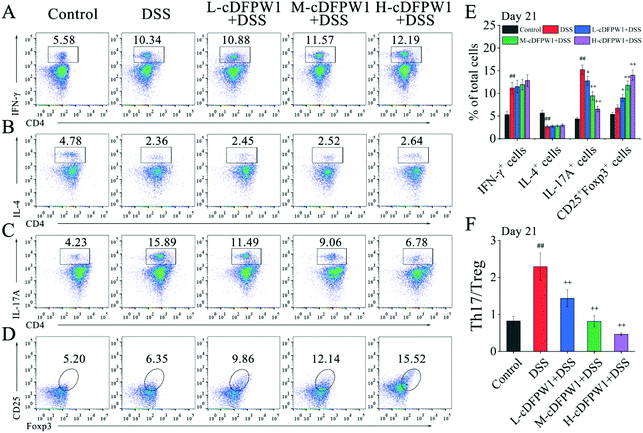 |
| Fig. 7 cDFPW1 restored colonic Th17 and Treg immune balance. Percentages of Th1 cells (A), Th2 cells (B), Th17 cells (C) and Treg cells (D) in colonic LPCs were detected by using flow cytometry assay. Percentage of T-cell subpopulations gated on CD4+ T cells (E), and ratio of Th17/Treg cells (F). Data are presented as mean ± SEM (n = 3). +p < 0.05 and ++p < 0.01 vs. DSS-induced model group. #p < 0.05 and ##p < 0.01 vs. control group on day 21. | |
3.7 cDFPW1 affected the expression of inflammation-related cytokines in DSS-induced colitis mice
Microarrays were applied to detect the changes of cytokine profiles in colon tissues. As compared with the control group, the expression levels of 8 cytokines after oral administration of cDFPW1 for 14 days were up-regulated, including IL-1β, IL-4, IL-5, IL-10, IL-12, IL-13, IL-22 and TNF-α (Fig. S6†). After 21 days, the pro-inflammatory cytokines IL-1β, IL-6, IL-17A, IL-17F, IL-21 and IL-23 were dramatically reduced in the colon tissue of colitis mice after cDFPW1 intervention, while the expression levels of IL-5, IL-10, IL-22, IFN-γ, TNF-α and TGFβ1 were up-regulated (Fig. 8A–L). These data manifested that supplementation of cDFPW1 significantly alters the cytokine profile in DSS-induced colitis mice, and its palliation effect on UC could be correlated with the regulation of specific inflammatory factors.
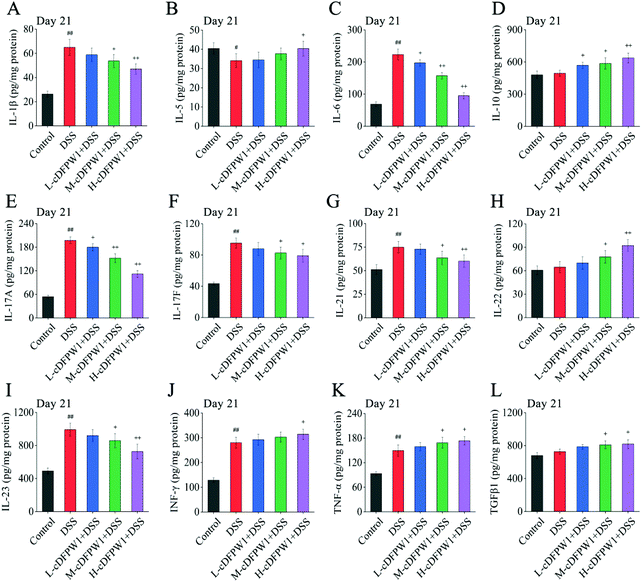 |
| Fig. 8 cDFPW1 regulated inflammatory cytokines from DSS-induced colitis mice. IL-1β (A), IL-5 (B), IL-6 (C), IL-10 (D), IL-17A (E), IL-17F (F), IL-21 (G), IL-22 (H), IL-23 (I), IFN-γ (J), TNF-α (K), and TGFβ1 (L). Data are presented as mean ± SEM (n = 4). +p < 0.05 and ++p < 0.01 vs. DSS-induced model group. #p < 0.05 and ##p < 0.01 vs. control group on day 21. | |
3.8 cDFPW1 resisted the oxidative stress in DSS-induced colitis mice
The oxidative stress in the colon tissue of mice was evaluated after supplementing with cDFPW1 for 14 days. It was found that cDFPW1 increased the activities of SOD, CAT and GSH-Px and reduced the concentration of MDA in a dose-dependent manner (Fig. 9A1–D1). After 21 days, the DSS challenge caused a decrease in the activities of SOD, CAT and GSH-Px and an increase in the MDA concentration as compared with the control group. However, cDFPW1 intervention prevented excessive oxidation stress in colitis mice, and the H-cDFPW1 + DSS group had the most significant effect (Fig. 9A2–D2). These data suggested that the alleviation of UC by cDFPW1 was related to the reduction in oxidative stress of colon tissue.
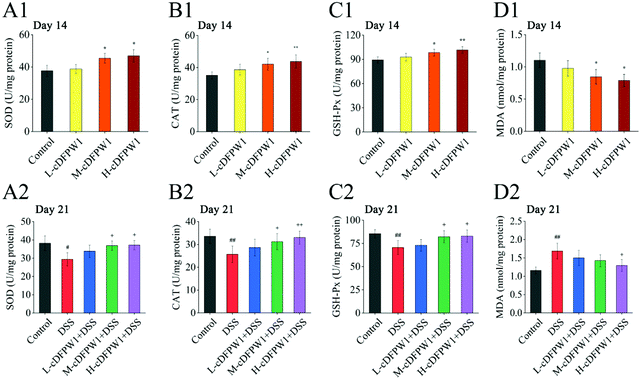 |
| Fig. 9 cDFPW1 inhibited oxidative-stress in DSS-induced colitis mice. SOD (A1 and A2), CAT (B1 and B2), GSH-Px (C1 and C2), and MDA (D1 and D2). Data are presented as mean ± SEM (n = 4). *p < 0.05 and **p < 0.01 vs. control group on day 14. +p < 0.05 and ++p < 0.01 vs. DSS-induced model group. #p < 0.05 and ##p < 0.01 vs. control group on day 21. | |
3.9 cDFPW1 regulated the Nrf2/NF-κB signaling pathways in colitis mice
Since the nuclear transcription factor Nrf2 and NF-κB signaling pathways play important roles in oxidative stress and inflammatory response, respectively, we detected these signaling pathways by western blotting. After oral administration of cDFPW1 for 14 days, the expression of nuclear Nrf2 was significantly up-regulated and Keap-1 was down-regulated acutely in the colon tissues of mice (Fig. 10A1 and B1). In the meantime, it was observed that the levels of nuclear NF-κB p65 and phosphorylation of IκB also showed an up-regulation trend (Fig. 10C1 and D1). This indicated that cDFPW1 may participate in the intestinal oxidative and inflammatory responses, and play an important role in maintaining intestinal homeostasis. After 21 days, the level of nuclear Nrf2 in the colon tissues of mice in the DSS group was significantly down-regulated and the expression level of Keap-1 was markedly up-regulated as compared with the control group, indicating that the antioxidative signaling pathways in model mice were significantly inhibited. Furthermore, the levels of nuclear NF-κB P65 and phosphorylation of IκB were increased significantly in the DSS treatment group. However, these phenomena were reversed after cDFPW1 intervention (Fig. 10A2–D2). The obtained results showed that the effect of cDFPW1 supplementation on colitis may be attributed to the activation of the Nrf2 antioxidative signaling pathway and the inhibition of the NF-κB inflammatory signaling pathway.
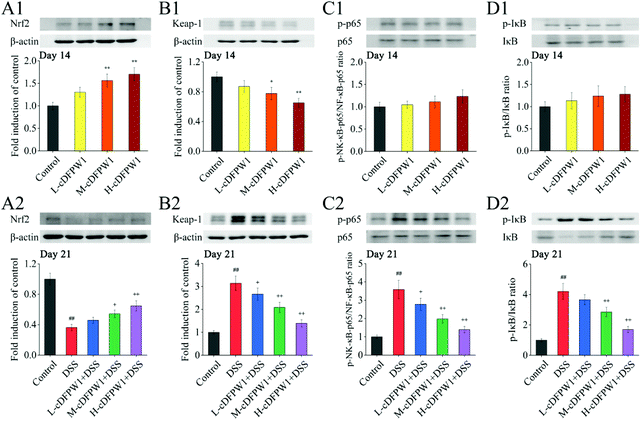 |
| Fig. 10 cDFPW1 regulated antioxidative and inflammatory signaling pathways in DSS-induced colitis mice. Nrf2 pathway (A1, A2, B1, and B2) and NF-κB pathway (C1, C2, D1, and D2). Data are presented as mean ± SEM (n = 3). *p < 0.05 and **p < 0.01 vs. control group on day 14. +p < 0.05 and ++p < 0.01 vs. DSS-induced model group. #p < 0.05 and ##p < 0.01 vs. control group on day 21. | |
4. Discussion
The development of UC is accompanied by a series of inflammation-related pathological changes in the colon,31 manifested as weight loss, diarrhea, bloody stools, ulcer and shortening of the colon length. The findings of the present study indicated that cDFPW1 significantly improved the symptoms of UC. The impaired intestinal mucosal barrier function is closely linked to the occurrence of UC,4,32 and was showed by sparse and shortened microvilli, expanded intercellular space, severe mucosal damage, loss of goblet cells, deformation of crypts and hyper-infiltration of neutrophils in our study. Goblet cells are the main line of defense of the intestinal mucosal barrier through secreting mucus proteins to resist the invasion of harmful substances.29 However, the supplement of cDFPW1 prevented intestinal mucosal damage, restored the mucosal ultrastructure, and increased the number of goblet cells in colitis mice in a dose-dependent manner. Similarly, the reduction of TJ proteins can lead to the increase of intestinal permeability, the translocation of bacteria and the increase of endotoxin, D-lactic acid and DAO in serum, which further aggravate the inflammatory response.29,33 In the present study, cDFPW1 intervention effectively enhanced the expression of occludin and ZO-1 and restored colonic TJs, which had positive effects on maintaining the integrity of the intestinal epithelial structure, thereby preventing the occurrence of colitis.
The intestinal flora affects the function of intestinal epithelial cells and the immune response of the host, which is essential for maintaining intestinal homeostasis, especially in IBD.34–36 As demonstrated in the present study, oral administration of cDFPW1 for 14 days increased the abundance of Lactobacillus, Ileibacterium, and Allobaculum in Firmicutes and the abundance of Akkermansia in Verrucomicrobiota. Lactobacillus affects the colonic barrier function by preventing cytokine-induced intestinal epithelial cell apoptosis and increasing the expression level of Muc2 mRNA in intestinal epithelial cells.37 Although the role of Ileibacterium in the host has not been well described, some studies have shown that the effects of oral catechins, flavonol glycosides and amino acids in preventing UC are positively correlated with Ileibacterium and Akkermansia.36Allobaculum is a potential probiotic that can produce butyrate.38Lachnospiraceae_NK4A136_group, unclassified_f__Lachnospiraceae, Bacteroides, and Helicobacter are considered to be relatively abundant flora in IBD patients or mouse colitis models,39–41 but cDFPW1 supplementation reduced their relative abundance, suggesting that the intake of this polysaccharide might cause changes in intestinal flora and intestinal metabolites, thereby playing a potentially positive role under host stress conditions. It has been reported that the development of UC is usually accompanied by a decrease in the biodiversity of intestinal microbes, which can be distinguished by a loss of beneficial bacteria and an increase in the abundance of harmful microorganisms.42 Lefse analysis results further showed that Turicibacter, Enterococcus, Parabacteroides, Parasutterella, Erysipelatoclostridium and Acinetobacter were mainly enriched in the DSS group, and they were closely related to the progression of colitis.40,43,44 Crohn's disease has been reported to have a high infection rate of Helicobacteraceae.41 Our data showed that cDFPW1 treatment could reduce the abundance of Helicobacteraceae in colitis mice. Meanwhile, Romboutsia, Lactobacillus and Odoribacter are believed to be beneficial for the production of intestinal microbiota metabolites SCFAs, and cDFPW1 supplementation significantly increased their relative abundance to prevent and inhibit inflammatory response,45,46 suggesting the role of cDFPW1 in maintaining the stability of the intestinal microenvironment.47 Therefore, cDFPW1 might exert an anti-inflammatory effect by regulating specific intestinal microbiota and their metabolites.
In the intestinal immune system, cytokines play an important role in the regulation of intestinal barrier function and the communication between cells.48 Our results showed that oral administration of cDFPW1 for 14 days increased the secretion of typical Th1-associated cytokines (IL-12 and IFN-γ) and the expression of inflammatory cytokines (IL-1β and TNF-α) to prime immune response,49,50 the up-regulation of Th2-related cytokines (IL-4, IL-5, and IL-13) to promote humoral immune response and the development of mucosal IgA response,29,51 and the release of IL-22 to activate intestinal stem cell proliferation and tissue regeneration for maintaining the intestinal epithelial cell integrity.52,53 These data mean that cDFPW1 has the function of regulating the intestinal mucosal immune response that is beneficial for the enhancement of host defense mechanisms. Recent studies have shown that the increased expression of Th17 cell-related cytokines IL-17A, IL-6 and IL-23 in UC disease patients is positively related to disease activity.54 In contrast to Th17 cells, Treg cells can inhibit intestinal inflammation caused by abnormal immune responses to autoantigens and symbiotic bacteria.55 Treg cells in the lamina propria of the colon specifically express the transcription factor Foxp3, which can effectively inhibit autoimmunity and prevent tissue damage by secreting the anti-inflammatory factor IL-10.54,55 It is worth mentioning that cDFPW1 could significantly adjust the balance state of Th17/Treg cells to improve the deterioration of colitis in the DSS treatment group, which was specifically manifested as a decrease in Th17 cells (CD4+ IL-17A+) and an increase in Treg cells (CD4+ CD25+ Foxp3+) along with the reduced secretion of IL-6, IL-17A, IL-17F, IL-21 and IL-23 as well as the increased expression of IL-10 and TGF-β1. In addition, the secretion of TGF-β can inhibit the inflammatory response of intestinal bacterial antigens to maintain the function of the epithelial barrier by regulating the expression levels of tight junction proteins and adhesion molecules,56,57 the secretion of IL-5, IL-22, IFN-γ and TNF-α, which may contribute to the activation of the self-repair system under intestinal injury, and the appropriate secretion of IL-1β can protect mice from intestinal infections by promoting the phagocytosis and elimination of bacteria in mononuclear phagocytes58,59 and regulating tight junction protein expression.60 Hence, it is recognized that the supplement of cDFPW1 may strengthen the self-defense ability of the intestines, regulate the function of the body's immune dynamic balance, and eventually reduce the severity of colitis.
A significant increase in the level of oxidative stress, which is one of the key factors in the pathogenesis of UC, can result in the loss of colonic mucosal barrier function and substantial reduction in TJs.61 In the current study, DSS-induced abnormal levels of anti-oxidative and pro-oxidative factors were recovered by cDFPW1 treatment. Meanwhile, cDFPW1 enhanced the expression level of Nrf2 and inhibited the phosphorylation of NF-κB signaling. Nrf2 and Keap1 are important proteins involved in the oxidative stress response in tissues, and the changes in their expression levels can reflect the oxidative stress state in colon tissues.62 In general, Nrf2 and NF-κB are inseparable during anti-inflammatory and antioxidative processes. Under normal conditions, NF-κB is usually combined with its inhibitory protein IκB and stored in the cytoplasm in an inactive form. When the body undergoes an inflammatory response, IκB kinase (IKK) can induce phosphorylation and degradation release of IκB, lead to the activation and nuclear translocation of NF-κB, upregulate the gene expression of inflammatory cytokines, and further induce the production of oxygen free radicals and cause tissue damage.63 Similarly, under the conditions of oxidative stress, Nrf2 separates from Keap-1 and transfers into the nucleus to bind to the antioxidative response element (ARE), leading to the activation of antioxidative enzymes including SOD, CAT and HO-1. Activated Nrf2 attenuates inflammatory signal transduction by inhibiting the entry of NF-κB into the nucleus, and NF-κB in turn directly inhibits the Nrf2 pathway at the RNA level.64 In fact, NF-κB and Nrf2 can competitively combine with CREB-binding protein (CBP) for transcription of their respective target genes. However, over-expressed p65 can preferentially bind to CBP to prevent Nrf2 transcriptional activity, leading to increased oxidative stress and aggravated UC symptoms.65 The agents that activate the Nrf2 signaling pathway and inhibit the NF-κB signaling pathway, such as Troxerutin and licochalcone A, have been shown to be effective in treating UC.62,64 In contrast, Nrf2−/− mice showed stronger signs of NF-κB activation in response to inflammatory stimulation as compared with the wild type mice,66 and Nrf2-regulated antioxidant enzymes such as SOD have been shown to regulate the signal transduction of NF-κB.67 Obviously, the crosstalk between Nrf2 and NF-κB signaling pathways might be an effective way to improve UC, and the data from the present study might indicate that Nrf2/NF-κB signaling is involved in cDFPW1-mediated protection against UC.
5. Conclusion
The present study demonstrated that Dendrobium fimbriatum Hook polysaccharide (cDFPW1) has the ability of alleviating UC through strengthening the intestinal barrier integrity, reshaping the structure composition of intestinal microbes and metabolites, recovering the balance of Th17/Treg cells, regulating the expression of specific cytokines, and inhibiting oxidative stress damage, and is associated with the Nrf2/NF-κB signal transducing pathways. Although the synergistic process involving Nrf2 and NF-κB signaling needed to be further explored, the great potential of cDFPW1 for alleviating UC would provide promising treatment strategies for the prevention of UC.
Author contributions
Conceptualization: Jian-Ping Luo; methodology, formal analysis, investigation and writing the original draft: Yu-Jing Wang; writing—review and editing: Yu-Jing Wang, Qiang-Ming Li, Xue-Qiang Zha and Jian-Ping Luo; project administration: Jian-Ping Luo. All authors have read and agreed to the published version of the manuscript.
Conflicts of interest
There are no conflicts of interest to declare.
Acknowledgements
This work was supported by the Project for Science and Technology Research Plan from Anhui Province of China (No. 18030801112).
References
- B. Khor, A. Gardet and R. J. Xavier, Genetics and pathogenesis of inflammatory bowel disease, Nature, 2011, 474, 307–317 CrossRef CAS.
- A. Kaser, S. Zeissig and R. S. Blumberg, Inflammatory bowel disease, Annu. Rev. Immunol., 2010, 28, 573–621 CrossRef CAS.
- G. P. Ramos and K. A. Papadakis, Mechanisms of Disease: Inflammatory Bowel Diseases, Mayo Clin. Proc., 2019, 94(1), 155–165 CrossRef CAS PubMed.
- Y. Belkaid and T. W. Hand, Role of the microbiota in immunity and inflammation, Cell, 2014, 157, 121–141 CrossRef CAS.
- M. F. Neurath, Cytokines in inflammatory bowel disease, Nat. Rev. Immunol., 2014, 14, 329–342 CrossRef CAS.
- F. Biasi, G. Leonarduzzi, P. I. Oteiza and G. Poli, Inflammatory bowel disease: mechanisms, redox considerations, and therapeutic targets, Antioxid. Redox Signaling, 2013, 19(14), 1711–1747 CrossRef CAS PubMed.
- R. Caruso, B. C. Lo and G. Nunez, Host-microbiota interactions in inflammatory bowel disease, Nature, 2020, 20, 411–426 CAS.
- S. V. Lynch and O. Pedersen, The human intestinal microbiome in health and disease, N. Engl. J. Med., 2016, 375(24), 2369–2379 CrossRef CAS.
- V. Tremaroli and F. Backhed, Functional interactions between the gut microbiota and host metabolism, Nature, 2012, 489, 242–249 CrossRef CAS.
- S. Wirtz, V. Popp, M. Kindermann, K. Gerlach, B. Weigmann and S. Fichtner-Feigl, Chemically induced mouse models of acute and chronic intestinal inflammation, Nat. Protoc., 2017, 12(7), 1295–1309 CrossRef CAS.
- P. K. Randhawa, K. Singh, N. Singh and A. S. Jaggi, A review on chemical-induced inflammatory bowel disease models in rodents, Korean J. Physiol. Pharmacol., 2014, 18(4), 279–288 CrossRef CAS PubMed.
- L. S. Celiberto, F. A. Graef, G. R. Healey, E. S. Bosman, K. Jacobson, L. M. Sly and B. A. Vallance, Inflammatory bowel disease and immunonutrition: novel therapeutic approaches through modulation of diet and the gut microbiome, Immunology, 2018, 155(1), 36–52 CrossRef CAS PubMed.
- S. Ben-Horin, R. Mao and M. Chen, Optimizing biologic treatment in IBD: objective measures, but when, how and how often?, BMC Gastroenterol., 2015, 15(1), 178 CrossRef PubMed.
- S. Renna, M. Cottone and A. Orlando, Optimization of the treatment with immunosuppressants and biologics in inflammatory bowel disease, World J. Gastroenterol., 2014, 20(29), 9675–9690 CrossRef PubMed.
- D. Lee, L. Albenberg, C. Compher, R. Baldassano, D. Piccoli, J. D. Lewis and G. D. Wu, Diet in the pathogenesis and treatment of inflammatory bowel diseases, Gastroenterology, 2015, 148(6), 1087–1106 CrossRef CAS PubMed.
- J. Liang, S. X. Chen, J. H. Chen, J. Z. Lin, Q. P. Xiong, Y. Q. Yang, J. Yuan, L. Zhou, S. Z. Hou, S. J. Li, S. Huang and X. P. Lai, Therapeutic roles of polysaccharides from Dendrobium Officinaleon colitis and its underlying mechanisms, Carbohydr. Polym., 2018, 185, 159–168 CrossRef CAS PubMed.
- L. J. Zhang, X. J. Huang, X. D. Shi, H. H. Chen, S. W. Cui and S. P. Nie, Protective effect of three glucomannans from different plants against DSS induced colitis in female BALB/c mice, Food Funct., 2019, 10(4), 1928–1939 RSC.
- L. F. Li, H. Yao, X. J. Li, Q. W. Zhang, X. Y. Wu, T. Wong, H. M. Zheng, H. Fung, B. X. Yang, D. Ma, C. Leung, G. Zhang, Z. Bian, A. Lu and Q. B. Han, Destiny of Dendrobium Officinale polysaccharide after oral administration: indigestible and non-absorbing, ends in modulating gut microbiota, J. Agric. Food Chem., 2019, 67(21), 5968–5977 CrossRef CAS.
- N. T. Porter and E. C. Martens, The critical roles of polysaccharides in gut microbial ecology and physiology, Annu. Rev. Microbiol., 2017, 71, 349–369 CrossRef CAS PubMed.
- Y. J. Wang, D. L. Wan, Q. M. Li, X. Q. Zha and J. P. Luo, Structural characteristics and immunostimulatory activities of a new polysaccharide from Dendrobium fimbriatum Hook, Food Funct., 2021, 12(7), 3057–3068 RSC.
- Q. Zhang, J. Li, M. Luo, G. Y. Xie, W. W. Zeng, Y. X. Wu, Y. H. Zhu, X. L. Yang and A. Y. Guo, Systematic Transcriptome and Regulatory Network Analyses Reveal the Hypoglycemic Mechanism of Dendrobium fimbriatum, Mol. Ther.–Nucleic Acids, 2020, 19, 1–14 CrossRef CAS.
- A. X. Luo and Y. J. Fan, In vitro Antioxidant of a Water-Soluble Polysaccharide from Dendrobium fimhriatum Hook.var.oculatum Hook, Int. J. Mol. Sci., 2011, 12(6), 4068–4079 CrossRef CAS.
- J. E. Ghia, P. Blennerhassett, H. Kumarondiveeran, E. F. Verdu and S. M. Collins, The vagus nerve: A tonic inhibitory influence associated with inflammatory bowel disease in a murine model, Gastroenterology, 2006, 131(4), 1122–1130 CrossRef.
- R. M. Stillie and A. W. Stadnyk, Role of TNF receptors, TNFR1 and TNFR2, in dextran sodium sulfate-induced colitis, Inflammatory Bowel Dis., 2010, 15(10), 1515–1525 CrossRef PubMed.
- Y. Chen, B. Yang, R. P. Ross, Y. Jin, C. Stanton, J. X. Zhao, H. Zhang and W. Chen, Orally administered CLA ameliorates DSS-induced colitis in mice via intestinal barrier improvement, oxidative stress reduction, inflammatory cytokine and gut microbiota modulation, J. Agric. Food Chem., 2019, 67(48), 13282–13298 CrossRef CAS PubMed.
- H. Y. Jeon, S. W. Ham, J. K. Kim, X. Jin, S. Y. Lee, Y. J. Shin, C. Y. Choi, J. K. Sa, S. H. Kim, T. Chun, X. Jin, D. H. Nam and H. Kim, Ly6G + inflammatory cells enable the conversion of cancer cells to cancer stem cells in an irradiated glioblastoma model, Cell Death Differ., 2019, 26(10), 2139–2156 CrossRef CAS PubMed.
- R. Singh, S. Chandrashekharappa, S. R. Bodduluri, B. V. Baby, B. Hegde, N. G. Kotla, A. A. Hiwale, T. Saiyed, P. Patel, M. Vijay-Kumar, M. G. I. Langille, G. M. Douglas, X. Cheng, E. C. Rouchka, S. J. Waigel, G. W. Dryden, H. Alatassi, H. G. Zhang, B. Haribabu, P. K. Vemula and V. R. Jala, Enhancement of the gut barrier integrity by a microbial metabolite through the Nrf2 pathway, Nat. Commun., 2019, 10(1), 89 CrossRef PubMed.
- H. Y. Wang, Q. M. Li, N. J. Yu, W. D. Chen, X. Q. Zha, D. L. Wu, L. H. Pan, J. Duan and J. P. Luo,
Dendrobium huoshanense polysaccharide regulates hepatic glucose homeostasis and pancreatic β-cell function in type 2 diabetic mice, Carbohydr. Polym., 2019, 211, 39–48 CrossRef CAS.
- S. Z. Xie, B. Liu, H. Y. Ye, Q. M. Li, L. H. Pan, X. Q. Zha, J. Liu, J. Duan and J. P. Luo,
Dendrobium huoshanense polysaccharide regionally regulates intestinal mucosal barrier function and intestinal microbiota in mice, Carbohydr. Polym., 2019, 206, 149–162 CrossRef CAS.
- X. Wang, G. Sun, T. Feng, J. Zhang, X. Huang, T. Wang, Z. Xie, X. Chu, J. Yang, H. Wang, S. Chang, Y. Gong, L. Ruan, G. Zhang, S. Yan, W. Lian, C. Du, D. Yang, Q. Zhang, F. Lin, J. Liu, H. Zhang, C. Ge, S. Xiao, J. Ding and M. Geng, Sodium oligomannate therapeutically remodels gut microbiota and suppresses gut bacterial amino acids-shaped neuroinflammation to inhibit Alzheimer's disease progression, Cell Res, 2019, 29(7), 1–17 CrossRef.
- A. Dashdorj, K. R. Jyothi, S. Lim, A. Jo, M. N. Nguyen, J. Ha, K. S. Yoon, H. J. Kim, J. H. Park, M. P. Murphy and S. S. Kim, Mitochondria-targeted antioxidant MitoQ ameliorates experimental mouse colitis by suppressing NLRP3 inflammasome-mediated inflammatory cytokines, BMC Med., 2013, 11(1), 178 CrossRef CAS PubMed.
- B. Pawłowska and B. M. Sobieszczańska, Intestinal epithelial barrier: The target for pathogenic Escherichia coli, Adv. Clin. Exp. Med., 2017, 26(9), 1437–1445 CrossRef.
- C. Yin, G. D. Noratto, X. Z. Fan, Z. Y. Chen, F. Yao, D. F. Shi and H. Gao, The impact of mushroom polysaccharides on gut microbiota and its beneficial effects to host: a review, Carbohydr. Polym., 2020, 250, 116942 CrossRef CAS PubMed.
- G. Gorkiewicz and A. Moschen, Gut microbiome: a new player in gastrointestinal disease, Virchows Arch., 2018, 472(1), 159–172 CrossRef CAS.
- L. W. Peterson and D. Artis, Intestinal epithelial cells: regulators of barrier function and immune homeostasis, Nat. Rev. Immunol., 2014, 14, 141–153 CrossRef CAS PubMed.
- Y. Liu, L. Y. Luo, Y. K. Luo, J. Zhang, X. H. Wang, K. Sun and L. Zeng, The prebiotic properties of green and dark tea contribute to protective effects in chemical-induced colitis in mice: a fecal microbiota transplantation study, J. Agric. Food Chem., 2020, 68(23), 6368–6380 CrossRef CAS.
- M. Wu, P. Li, Y. Y. An, J. Ren, D. Yan, J. Z. Cui, D. Li, M. Li, M. Y. Wang and G. S. Zhong, Phloretin ameliorates dextran sulfate sodium-induced ulcerative colitis in mice by regulating the gut microbiota, Pharmacol. Res., 2019, 150, 104489 CrossRef CAS PubMed.
- E. Herrmann, W. Young, D. Rosendale, V. Reichert-Grimm, C. U. Riedel, R. Conrad and M. Egert, RNA-based stable isotope probing suggests allobaculum spp. as particularly active glucose assimilators in a complex murine microbiota cultured in vitro, BioMed Res. Int., 2017 DOI:10.1155/2017/1829685.
- M. N. Quraishi, M. Sergeant, G. Kay, T. Iqbal, J. Chan, C. Constantinidou, P. Trivedi, J. Ferguson, D. H. Adams, M. Pallen and G. M. Hirschfield, The gut-adherent microbiota of PSC–IBD is distinct to that of IBD, Gut, 2017, 66(2), 386–388 CrossRef.
- P. Wan, Y. J. Peng, G. J. Chen, M. H. Xie, Z. Q. Dai, K. Y. Huang, W. Dong, X. X. Zeng and Y. Sun, Modulation of gut microbiota by Ilex kudingcha improves dextran sulfate sodium-induced colitis, Food Res. Int., 2019, 126, 108595 CrossRef CAS PubMed.
- A. L. Li, W. W. Ni, Q. M. Zhang, Y. Li, X. Zhang, H. Y. Wu, P. Du, J. C. Hou and Y. Zhang, Effect of cinnamon essential oil on gut microbiota in the mouse model of dextran sodium sulfate–induced colitis, Microbiol. Immunol., 2019, 64(1), 23–32 CrossRef.
- G. Pickert, S. Wirtz, J. Matzner, M. Ashfaq-Khan, R. Heck, S. Rosigkeit, D. Thies, R. Surabattula, D. Ehmann, J. Wehkamp, M. Aslam, G. W. He, A. Weigert, F. Foerster, L. Klotz, J. Frick, C. Becker, E. Bockamp and D. Schuppan, Wheat consumption aggravates colitis in mice via amylase trypsin inhibitor-mediated dysbiosis, Gastroenterology, 2020, 159(1), 257–272 CrossRef CAS PubMed.
- Z. Q. Zha, Y. Lv, H. L. Tang, T. T. Li, Y. H. Miao, J. W. Cheng, G. Q. Wang, Y. Y. Tan, Y. Zhu, X. Xing, K. Ding, Y. Wang and H. P. Yin, An orally administered butyrate-releasing xylan derivative reduces inflammation in dextran sulphate sodium-induced murine colitis, Int. J. Biol. Macromol., 2019, 156, 1217–1233 CrossRef PubMed.
- W. Yu, X. Su, W. Chen, X. Tian, K. Zhang, G. Guo, L. X. Zhou, T. Zeng and B. Han, Three types of gut bacteria collaborating to improve Kuijie’an enema treat DSS-induced colitis in mice, Biomed. Pharmacother., 2019, 113, 108751 CrossRef PubMed.
- M. Y. Lin, M. R. de Zoete, J. P. M. van Putten and K. Strijbis, Redirection of epithelial immune responses by short-chain fatty acids through inhibition of histone deacetylases, Front. Immunol., 2015, 6, 554 Search PubMed.
- Y. J. Liu, B. Tang, F. C. Wang, L. Tang, Y. Y. Lei, Y. Luo, S. J. Huang, M. Yang, L. Y. Wu, W. Wang, S. Liu, S. M. Yang and X. Y. Zhao, Parthenolide ameliorates colon inflammation through regulating Treg/Th17 balance in a gut microbiota-dependent manner, Theranostics, 2020, 10(12), 5225–5241 CrossRef CAS.
- P. Goncalves, J. R. Araujo and J. P. D. Santo, A Cross-Talk Between Microbiota-Derived Short-Chain Fatty Acids and the Host Mucosal Immune System Regulates Intestinal Homeostasis and Inflammatory Bowel Disease, Inflammatory Bowel Dis., 2018, 24(3), 558–572 CrossRef PubMed.
- C. L. Xu, R. Sun, X. J. Qiao, C. C. Xu, X. Y. Shang and W. N. Niu, Protective effect of glutamine on intestinal injury and bacterial community in rats exposed to hypobaric hypoxia environment, World J. Gastroenterol., 2014, 20(16), 4662–4674 CrossRef CAS.
- V. A. K. Rathinam and F. K. Chan, Inflammasome, Inflammation and Tissue Homeostasis, Trends Mol. Med., 2018, 24(3), 304–318 CrossRef CAS.
- B. Burkholder, R. Y. Huang, R. Burgess, S. H. Luo, V. S. Jones, W. J. Zhang, Z. Q. Lv, C. Y. Gao, B. L. Wang, Y. M. Zhang and R. P. Huang, Tumor-induced perturbations of cytokines and immune cell networks, Biochim. Biophys. Acta, 2014, 1845(2), 182–201 CAS.
- H. W. Guo, C. X. Yun, G. H. Hou, J. Du, X. Huang, Y. Lu, E. T. Keller, J. Zhang and J. G. Deng, Mangiferin attenuates TH1/TH2 cytokine imbalance in an ovalbumin-induced asthmatic mouse model, PLoS One, 2014, 9(6), e100394 CrossRef PubMed.
- C. A. Lindemans, M. Calafiore, A. M. Mertelsmann, M. H. O’Connor, J. A. Dudakov, R. R. Jenq, E. Velardi, L. F. Young, O. M. Smith, G. Lawrence, J. A. Ivanov, Y. Y. Fu, S. Takashima, G. Hua, M. L. Martin, K. P. O’Rourke, Y. H. Lo, M. Mokry, M. Romera-Hernandez, T. Cupedo, L. E. Dow, E. E. Nieuwenhuis, N. F. Shroyer, C. Liu, R. Klesnick, M. R. M. van den Brink and A. M. Hanash, Interleukin-22 promotes intestinal-stem-cell-mediated epithelial regeneration, Nature, 2015, 528, 560–564 CrossRef CAS PubMed.
- P. Aparicio-Domingo, M. Romera-Hernandez, J. J. Karrich, F. Cornelissen, N. Papazian, D. J. Lindenbergh-Kortleve, J. A. Butler, L. Boon, M. C. Coles, J. N. Samsom and T. Cupedo, Type 3 innate lymphoid cells maintain intestinal epithelial stem cells after tissue damage, J. Exp. Med., 2015, 212(11), 1783–1791 CrossRef CAS PubMed.
- L. Y. Zhang, Y. J. Zhang, W. W. Zhong, C. X. Di, X. L. Lin and Z. W. Xia, Heme oxygenase-1 ameliorates dextran sulfate sodium-induced acute murine colitis by regulating Th17/Treg cell balance, J. Biol. Chem., 2014, 289(39), 26847–26858 CrossRef CAS.
- A. Ueno, H. Jijon, R. Chan, K. Ford, C. Hirota, G. G. Kaplan, P. L. Beck, M. Iacucci, M. F. Gasia, H. W. Barkema, R. Panaccione and S. Ghosh, Increased prevalence of circulating novel IL-17 secreting Foxp3 expressing CD4+ T cells and defective suppressive function of circulating Foxp3+ regulatory cells support plasticity between Th17 and regulatory T cells in inflammatory bowel disease patients, Inflammatory Bowel Dis., 2013, 19(12), 2522–2534 CrossRef PubMed.
- A. D. Sabatino, K. M. Pickard, D. Rampton, L. Kruidenier, L. Rovedatti, N. A. B. Leakey, G. R. Corazza, G. Monteleone and T. T. MacDonald, Blockade of transforming growth factor beta upregulates T-box transcription factor T-bet, and increases T helper cell type 1 cytokine and matrix metalloproteinase-3 production in the human gut mucosa, Gut, 2008, 57(5), 605–612 CrossRef PubMed.
- P. Biancheri, A. D. Sabatino, G. R. Corazza and T. T. Macdonald, Proteases and the gut barrier, Cell Tissue Res., 2013, 351(2), 269–280 CrossRef CAS PubMed.
- M. Alipour, Y. F. Lou, D. Zimmerman, M. W. Bording-Jorgensen, C. Sergi, J. J. Liu and E. Wine, A balanced IL-1βactivity is required for host response to Citrobacter rodentium infection, PLoS One, 2013, 8(12), e80656 CrossRef PubMed.
- M. Hasegawa, N. Kamada, Y. Z. Jiao, M. Z. Liu, G. Núñez and N. Inohara, Protective role of commensals against Clostridium difficile infection via an IL-1β-mediated positive-feedback loop, J. Immunol., 2012, 189(6), 3085–3091 CrossRef CAS.
- M. Rawat, M. Nighot, R. Al-Sadi, Y. Gupta, D. Viszwapriya, G. Yochum, W. Koltun and T. Y. Ma, IL1B increases intestinal tight junction permeability by up-regulation of MIR200C-3p, which degrades Occludin mRNA, Gastroenterology, 2020, 159(4), 1375–1389 CrossRef CAS PubMed.
- G. S. Seo, W. Y. Jiang, P. H. Park, D. H. Sohn, J. H. Cheon and S. H. Lee, Hirsutenone reduces deterioration of tight junction proteins through EGFR/AKT and ERK1/2 pathway both converging to HO-1 induction, Biochem. Pharmacol., 2014, 90(2), 115–125 CrossRef CAS PubMed.
- X. Q. Wang, Y. Z. Gao, L. Wang, D. Yang, W. Bu, L. S. Gou, J. J. Huang, X. Y. Duan, Y. Pan, S. Y. Cao, Z. X. Gao, C. Cheng, Z. J. Feng, J. Xie and R. Q. Yao, Troxerutin improves dextran sulfate sodium-induced ulcerative colitis in mice, J. Agric. Food Chem., 2021, 69(9), 2729–2744 CrossRef CAS PubMed.
- S. K. Ramakrishnan, H. B. Zhang, X. Y. Ma, I. Jung, A. J. Schwartz, D. Triner, S. N. Devenport, N. K. Das, X. Xue, M. Y. Zeng, Y. L. Hu, R. M. Mortensen, J. K. Greenson, M. Cascalho, C. E. Wobus, J. A. Colacino, G. Nunez, L. Y. Rui and Y. M. Shah, Intestinal
non-canonical NF-κB signaling shapes the local and systemic immune response, Nat. Commun., 2019, 10, 660 CrossRef CAS PubMed.
- S. Saber, R. M. Khalil, W. S. Abdo, D. Nassif and E. El-Ahwany, Olmesartan ameliorates chemically-induced ulcerative colitis in rats via modulating NF-κB and Nrf-2/HO-1 signaling crosstalk, Toxicol. Appl. Pharmacol., 2018, 364, 120–132 CrossRef PubMed.
- G. H. Liu, J. Qu and X. Shen, NF-κB/p65 antagonizes Nrf2-ARE pathway by depriving CBP from Nrf2 and facilitating recruitment of HDAC3 to MafK, Biochim. Biophys. Acta, 2008, 1783(5), 713–727 CrossRef CAS PubMed.
- R. K. Thimmulappa, H. Lee, T. Rangasamy, S. P. Reddy, M. Yamamoto, T. W. Kensler and S. Biswal, Nrf2 is a critical regulator of the innate immune response and survival during experimental sepsis, J. Clin. Invest., 2006, 116(4), 984–995 CrossRef CAS PubMed.
- A. E. Frakes, L. Ferraiuolo, A. M. Haidet-Phillips, L. Schmelzer, L. Braun, C. J. Miranda, K. J. Ladner, A. K. Bevan, K. D. Foust, J. P. Godbout, P. G. Popovich, D. C. Guttridge and B. K. Kaspar, Microglia induce motor neuron death via the classical NF-κB pathway in amyotrophic lateral sclerosis, Neuron, 2014, 81(5), 1009–1023 CrossRef CAS PubMed.
Footnote |
† Electronic supplementary information (ESI) available. See DOI: 10.1039/d1fo03003e |
|
This journal is © The Royal Society of Chemistry 2022 |