DOI:
10.1039/D1FO02795F
(Paper)
Food Funct., 2022,
13, 131-142
The enzymatic synthesis of EPA-rich medium- and long-chain triacylglycerol improves the digestion behavior of MCFA and EPA: evidence on in vitro digestion
Received
25th August 2021
, Accepted 17th November 2021
First published on 18th November 2021
Abstract
Medium-chain triglyceride (MCT) and eicosapentaenoic acid (EPA) have been widely applied in nutritional supplementation. However, when administered individually or mixed, they were unable to maximize their nutritional value. Hence, EPA-rich medium- and long-chain triacylglycerol (MLCT) was synthesized from MCT and EPA-rich fish oil (FO) by enzymatic transesterification. The fatty acids in triglyceride (TAG) were rearranged which resulted in significant changes in TAG profiles compared to the physical mixture of MCT and FO (PM). EPA-containing MML (MML, MLM and LMM) and LLM (LLM, LML and MLL) type TAGs account for 70.21%. The fate of different oils (MCT, FO, PM, and MLCT) across the gastrointestinal tract was subsequently simulated using an in vitro digestion model. The results showed that the physical and structural characteristics of different oils during digestion depended upon the oil type and the microenvironment they were in. After 120 min of small intestine digestion, the degree of hydrolysis for MLCT was higher than that for the other three oils. The final FFA release level was in the following order: MLCT (102.79%) > MCT (95.20%) > PM (85.81%) > FO (74.18%). This can be attributed to the composition and positional distribution of fatty acids in TAGs. What's more, LCFAs (EPA) in MLCT mainly existed in the form of sn-2 MAG, which was conducive to their subsequent absorption and transport. These results may aid in the future rational design of structural lipids, thereby regulating lipid digestion and maximizing the nutritional value of oils.
1. Introduction
Malnutrition is highly prevalent in severely ill patients or postoperative patients, such as patients with cancer or after liver resection.1,2 Malnutrition is often accompanied by abnormal energy metabolism and can lead to a decline in body weight and systemic immune function, which in turn increase the chances of other infections, mortality, and treatment related complications.3 Therefore, appropriate nutritional supplementation is essential to improve health outcomes of patients with malnutrition.
Some nutritional lipids as important sources of nutrients and energy have been extensively used to improve the nutritional status of malnourished patients, especially for those who also have disorders of lipid metabolism.2
Medium-chain triglyceride (MCT) is an excellent substrate to provide a rapid way to obtain energy under the conditions of metabolic demand. MCT exhibits low dependence on bile salt and pancreatin, and it can be easily cleaved by pancreatic lipase to yield glycerol and medium-chain fatty acids (MCFAs) in the small intestine. MCFAs travel through the epithelium directly to the portal vein, and then transport to the liver as free fatty acids (FFAs).4 In the deficiency of pancreatic lipase, a large fraction of MCT can be absorbed as triacylglycerol, whereas LCT is not absorbed. Therefore, MCT has a wide range of applications in nutritional therapy based on its rapid digestion and energy supplement.5 However, MCT also has several obvious shortcomings. From a nutritional point of view, MCT is not able to provide sufficient LCFAs that is essential to deliver essential fatty acids to maintain the normal physiological function of the body. Furthermore, the rapid metabolism of MCT leads to unstable energy supply and generation of ketone bodies.6
The importance of supplementation of omega-3 polyunsaturated fatty acids (PUFAs) in nutritional intervention of patients is self-evident, as they can suppress inflammatory response and modulate key metabolic functions under certain conditions, and in turn improve the appetite and nutritional state of patients.7,8 In recent years, dietary eicosapentaenoic acid (EPA) has received much attention for its indirect effects on clinical treatment. It can reduce drug side effects and complications.9 In addition, dietary EPA supplementation has potential benefits on muscle mass and body weight preservation.10,11 Thus, dietary EPA supplementation is a particularly relevant strategy to be implemented for targeted nutritional treatment of malnourished patients. As is well known, a critical factor that influences the physiological responses of fatty acids and their metabolic fate is the speed of their intestinal digestion, which further affects their subsequent absorption and may affect their nutritional properties.12,13 However, EPA has a highly curved structure and a double bond close to the carboxyl group, which result in a lower hydrolysis rate and reduced bioavailability of EPA.14 Therefore, how to improve the digestive efficiency of EPA is an important and challenging problem that needs to be addressed.
To better provide patients with lipid nutrition, physical mixtures of MCT and fish oil (PM) were the most commonly used. However, the physical blend of MCT and fish oil neither slowed down the energy-providing rate of MCT nor improved the absorption of LCFAs, because each of the individual triacylglycerols maintained its original absorption rate. Moreover, the LCFAs at the primary positions of randomized triacylglycerols also had a slower hydrolysis rate. An effective solution is the manufacture and use of a medium- and long-chain triglyceride (MLCT) containing both LCFAs and MCFAs in a glycerol backbone, which can maximize the potential for effective functioning of each fatty acid.15 In fact, MLCT is more digestible and absorbable due to its unique structure, especially MLM-type structured TAGs, the sn-1,3 MCFAs which are used to provide immediate energy, and sn-2 LCFAs which are used to provide some essential nutrients to the body.4 In addition, the safety and functionality of MLCT related products have been recognized successively in Europe, United States and Japan.2 In this study, an EPA-rich MLCT was designed and synthesized by biocatalytic technology from MCT and EPA-rich fish oil. Gas chromatography (GC) and ultra-performance liquid chromatography/quadrupole time-of-flight tandem mass spectrometry (UPLC-Q-TOF-MS) were employed to analyze the fatty acid composition and TAG profiles of four different oils (MCT, FO, PM and MLCT). Moreover, the potential gastrointestinal fate of these four oils was examined under standardized simulated gastrointestinal conditions, associated with physical and structural features, the degree of lipid hydrolysis and lipid composition analysis to determine whether an EPA-rich MLCT is more suitable for nutritional therapy. Ultimately, we aimed to provide a basic understanding of differences in digestive characteristics of different oils, which might facilitate the rational design of structural lipids, and further control lipid digestion and improve the applicability in nutritional therapy for malnourished patients.
2. Materials and methods
2.1 Materials and chemicals
EPA-rich fish oil was sponsored by Seawit Ltd (Qingdao, China). Medium chain triglyceride was obtained from Youchuang Industry Co., Ltd (Shanghai, China). Lipozyme RM IM was obtained from Novozymes Biotechnology Co., Ltd (Beijing, China). Porcine pancreatic lipase (type II, Sigma catalog number L-3126, 100–400 units per mg), methyl nonadecanoate (C19:0), and standard FAME mixture (Supelco 37 Component FAME Mix) were obtained from Sigma-Aldrich Co. (Shanghai, China). Mucin from porcine stomach was purchased from Husbio Technology Co., Ltd (Shanghai, China). Tween 20, Nile red and all the HPLC-grade solvents were obtained from J&K Scientific Ltd (Shanghai, China). Bile salt (pig, 69005361-25 g) and other chemical reagents of analytical grade were obtained from Sinopharm Chemical Reagent Co. Ltd (Shanghai, China). All solutions and emulsions were prepared with ultrapure water (Millipore, Bedford, USA).
2.2 Enzymic synthesis, purification, and identification of MLCT
2.2.1 Enzymic synthesis and purification.
Enzymatic synthesis of MLCT was modified based on methods previously reported by our laboratory.16 At the outset of our studies, the effects of the reaction conditions (substrate ratio, temperature, enzyme load and reaction time) were studied in order to optimize the yield of the MLCT and EPA content. The optimized reaction conditions were as follows: the transesterification reaction of EPA-rich fish oil and MCT (weight ratio 1
:
3) was performed with Lipozyme RM IM (8% of the total mass of the substrate) at 60 °C for 6 h in a 100 mL reactor flask. A filter membrane was used to remove the lipase when the reaction was completed. The synthetic product obtained from the transesterification reaction was further purified by molecular distillation (KDL 1, Alzenau-Hoerstein, Germany). Molecular distillation was carried out at the evaporation temperature of 200 °C, and the rest of the operating parameters were as follows: the rotation speed was set to 120 rpm, the feed temperature was set to 65 °C, the feed flow rate was set to 2 mL min−1, the vacuum level was set to 10−3 mbar and the condenser temperature was set to 30 °C.17 The purified oil was stored in −20 °C for further experiments.
2.2.2 Identification of lipid composition.
2.2.2.1 Fatty acid composition analysis.
The fatty acid composition of oils was determined by GC. The specific detection conditions were according to a previous study from our laboratory.16 The preparation procedure of FAMEs is briefly described as follows.18 The oil (25 mg) was dissolved in 0.5 mL of KOH-CH3OH (2 mol L−1) and 1.5 mL of hexane. After 1 min of oscillation, an appropriate amount of Na2SO4 was added to remove water. The obtained organic phase was filtered with a filter (pore size, 0.22 μm) for GC analysis. Individual fatty acids were identified by comparing the relative retention times of FAME peaks of the samples with those of the FAME standards, and expressed as the percentage (%) of FAME.
The analysis of sn-2 FA composition mainly referred to the method described by Qi et al.18 Briefly, 70 mg of oil was first mixed together with the working fluid and hydrolyzed in a shaking water bath (80 agitations per min) at 37 °C for 6 min, and 2-mono-acylglycerol was achieved. The working fluid consisted of 1 M Tris-HCl buffer (Tris adjusted to pH 7.6 with HCl; 7 mL), 0.05% (wt/vol) bile salts (1.75 mL), 2.2% (wt/vol) CaCl2 (0.7 mL) and 70 mg of pancreatic lipase. Then, diethyl ether (2 mL) was added and mixed well. After the separation of phases, the upper organic phase was collected and evaporated to around 300 μL under a nitrogen stream. The hydrolysis products were separated by thin-layer chromatography (TLC) on silica gel plates using hexane/diethyl ether/acetic acid (50
:
50
:
1, vol/vol/vol). The band corresponding to sn-2 mono-acylglycerol (MAG) was detected by iodine for color development, scraped off, and then extracted, methylated and analyzed via GC as described above.
2.2.2.2 TAG profile analysis.
TAG profile analysis was conducted using ultra-performance liquid chromatography/quadrupole time-of-flight tandem mass spectrometry (Waters, Milford, MA, USA). The specific experimental procedures were performed as described in a previously published work from our lab.19 The Waters MassLynx V4.1 software was used to process and analyze the MS data (Waters, Milford, MA, USA).
2.3 Preparation of digestion emulsion
The original oil-in-water emulsion consisted of 10 wt% oil phase and 90 wt% aqueous phase. The aqueous phase was prepared by dispersing 1.0 wt% Tween 20 into 99.0 wt% 5 mM phosphate buffered saline (PBS, pH 7.0) and stored overnight at 4 °C to ensure adequate hydration. The oil phase was added to the aqueous phase, followed by homogenizing with a high-speed Ultra-Turrax blender (T25, IKA, Germany) at 19
200 rpm for 2 min to form a crude emulsion, and then transferred into a high-pressure homogenizer (HPH2000/4-SH5, GEA, Germany) at 60/620 bar (first-stage pressure/second-stage pressure) for 5 cycles. The original oil-in-water emulsion was diluted 5-fold in PBS and used for the in vitro digestion experiment. The gastrointestinal fate of the emulsion was simulated in an in vitro static simulated gastrointestinal digestion model consisting of mouth, stomach, and intestinal phases.
2.4 In vitro digestion
Mouth stage: Simulated artificial saliva stock solution (SASS: 27.28 mM NaCl, 4.10 mM NH4NO3, 4.67 mM KH2PO4, 2.71 mM KCl, 0.95 mM C6H5K3O7, 0.11 mM C5H3N4O3Na, 3.30 mM CH4N2O, 1.30 mM C3H5O3Na) was prepared referring to a previously published method.20 A 60 mL aliquot of the emulsions containing 2 wt% oil phase was put into a 200 mL glass container, and 60 mL of SASS containing 1.8 g of mucin was added and mixed thoroughly. The pH of the mixture was adjusted to 6.8, and then incubated for 2 min at 37 °C and 100 rpm in a shaking incubator (Incubator Shaker, THZ-412, Jing Hong Instrument, Shanghai, China).
Stomach stage: Simulated gastric fluid (SGF: 34.22 mM NaCl, 226.11 mM HCl) was prepared. 75 mL of the digestion product from the mouth stage was then mixed with an equal volume of SGF, subsequent to adjusting the pH to 2.5 with 1 M and 0.1 M HCl. Then the mixture was shaken continuously at 37 °C for 2 h at 100 rpm.
Small intestine stage: Simulated intestinal fluid (SIF: 3.75 M NaCl, 249.49 mM CaCl2·2H2O) was prepared. 60 mL of the digestion product from the stomach stage was poured into a glass container connected to a 37 °C circulating water bath. The pH of product was adjusted to 7.0 with NaOH, and then 7 mL of bile salts (pH 7.0, 53.57 g L−1) was added and the pH was readjusted to 7.0 again. After that, 5 mL of pancreatic lipase solution (1.6 mg mL−1, freshly prepared) was mixed with the sample and the automatic titration program was initiated immediately. During this process, TAGs were hydrolyzed to a complex mixture of diacylglycerols (DAGs), monoacylglycerols (MAGs), and free fatty acids (FFAs) by pancreatic lipase. The real-time released FFAs were monitored with a pH-stat automatic titration titrator (Hanon T-960, Jinan, China), and the consumed volume of 0.1 M NaOH (in mL) to maintain the sample pH at 7.00 ± 0.05 was recorded with a software program.21 The digestion curves were reported as FFA released (%) values versus small intestinal digestion time (min). The percentage of FFAs released during digestion was calculated using formula (1):
|  | (1) |
where
VNaOH (L) is the consumed volume of NaOH solution in titration,
mNaOH (0.1 mM) is the molarity of the NaOH solution,
WLipid (g) is the total mass of lipid added initially in digestion system, and
MLipid (g mol
−1) is the mean molecular weight of the oils (MCT: 504.37 g mol
−1; FO: 957.46 g mol
−1; PM: 644.23 g mol
−1; MLCT: 779.18 g mol
−1). All the simulated digestion fluids were prewarmed at 37 °C for 10 minutes before each digestion phase.
2.5 Particle size and ζ-potential characterization
Mean particle size and ζ-potential of the four different emulsions were measured immediately after they were produced by high-pressure homogenization. Digestion products were taken immediately after each stage of digestion for measurement. Particle size analyses were measured using the Microtrac S3500 laser scattering particle size analyzer (Microtrac, USA). The mean particle sizes of emulsion droplets were expressed as the surface-weighted mean diameter, D[3,2] (μm). The particle charge (ζ-potential) measurements were performed by the microelectrophoretic method using a NanoBrook Omni zeta potential analyzer (Brookhaven Instruments, USA) referring to the previous method of Zou et al.22 All the measurements were performed in triplicate at 25 °C, and the mean values are reported.
2.6 Microstructure observation
The microstructure changes of samples at the different stages of in vitro digestion were observed by inverted fluorescent microscopy (Nikon ECLIPSE TS100/100-F, Japan). 1 mL of each sample solution stained with 20 μL of Nile red solution (0.02%, wt/vol, in ethanol) at 4 °C in the dark for 30 min before microscopy images were taken.
2.7 Lipid analysis of the digestion products
Lipids were extracted from the collected digestion products using ultrasonic extraction with chloroform–methanol (2
:
1, vol/vol) for 10 min to analyze the composition of the lipids.
2.7.1 Lipid composition analysis.
A high-performance liquid chromatography system equipped with a refractive index detector (HPLC-RID) (Waters Corp., Milford, MA) was employed to analyze the lipid composition of oil extracts, which included FFAs, MAGs, DAGs and TAGs. The specific experimental procedures of HPLC and data processing were conducted with reference to previously published paper from our lab.17 The percentage of lipolysis degree during digestion was calculated according to formula (2). | 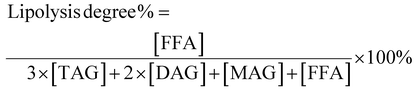 | (2) |
where [FFA], [TAG], [DAG], and [MAG] are the concentrations (mol L−1) of FFAs, TAGs, DAGs, and MAGs obtained by hydrolysis, respectively.
2.7.2 FFA content analysis.
FFAs released were separated by TLC using hexane: diethyl ether: acetic acid (70
:
30
:
2, vol/vol/vol) as the developing solvents. The band corresponding to the FFAs was collected and extracted with diethyl ether (two times; 5 mL). The solvents were then evaporated and methylation of the residue samples (25 mg each) was performed using BF3-CH3OH (1
:
3, vol/vol) at 70 °C for 5 min after the addition of methyl nonadecanoate (5 mg ml−1) as internal standards.23,24 Afterward, FAMEs were extracted with 1 mL hexane, filtered, and transferred to a GC vial ready for GC analysis using the method described in section 2.2.3.1.
2.8 Statistical analysis
All of the experiments were performed with 3 replicates. All the experimental data were expressed as the mean ± standard deviation (SD) and were analyzed using the SPSS 22.0 software (IBM, Armonk, NY, USA). One-way analysis of variance (ANOVA) with Duncan's test was used to determine the statistical significance. The statistical significance of a difference was declared when p < 0.05.
3. Results and discussion
Operating under the previously optimized reaction conditions, MLCT containing both MCFAs and LCFAs was obtained in 63.6% yield. Then the purity of triglyceride content was above 96% after molecular distillation.
3.1 Fatty acid composition and TAG profile of the experimental oils
The composition of total FA and sn-2 position FA in four experimental oils (MCT, FO, PM and MLCT) is summarized in Table 1. The major fatty acids of MCT were octanoic acid (C8:0) and decanoic acid (C10:0) (52.97% and 44.53%, respectively), while the major fatty acids of FO were EPA (C20:5) and DHA (C22:6) (62.92% and 18.48%, respectively). The total fatty acid composition of PM was consistent with that of purified MLCT (p > 0.05). The predominant fatty acids in both oils were C8:0, C10:0, C20:5 and C22:6 (17.88% vs. 17.76%, 19.02% vs. 19.31%, 36.08% vs. 35.92% and 10.79% vs. 10.95%, respectively). It is noteworthy that there was a significant difference (p < 0.05) between the sn-2 position FA composition of PM and MLCT. The relative contents of LCFAs existed at the sn-2 position of PM and MLCT were 56.31% and 70.65%, respectively (p < 0.05), and MCFAs were 43.69% and 29.35%, respectively (p < 0.05). These results demonstrated that enzymatic transesterification by Lipozyme RM IM has some influence on the FA distribution of the oils. This is mainly because of this enzyme having a certain positional specificity.25 The positional distribution of fatty acids in TAG is of importance in fatty acid metabolism.12 In particular, TAG molecules containing LCFAs at the sn-2 position and MCFAs primarily at the sn-1,3 positions are conducive to the hydrolysis of TAGs by pancreatic lipase.13
Table 1 The fatty acid compositions of different oils (MCT, FO, PM and MLCT) expressed in relative percentagea
Fatty acid (% of total fatty acids) |
MCT |
FO |
PM (MCT/FO = 1 : 1.2) |
MLCT |
Total |
sn-2 |
sn-1,3b |
Total |
sn-2 |
sn-1,3 |
Total |
sn-2 |
sn-1,3 |
Total |
sn-2 |
sn-1,3 |
MCT: medium-chain triglyceride; FO: EPA-rich fish oil; PM: the physical mixture the MCT and FO; MLCT was synthesized with Lipozyme RM IM of 8 wt% and substrate at 60 °C for reaction time of 6 h. SFA: total saturated fatty acids; UFA: total unsaturated fatty acids; different lower case letters (a–d) indicate significant difference (p < 0.05) in total fatty acids among four different oils. Different capital letters (A–D) denote significant difference (p < 0.05) in sn-2 position fatty acid among four different oils. Different capital letters (E–H) denote significant difference (p < 0.05) in sn-1,3 position fatty acids among four different oils.
FA composition at sn-1,3 positions was calculated as (3 × total FA − sn-2 FA)/2.
nd, not detected.
|
C 8:0 |
52.97 ± 1.19a |
28.27 ± 0.85A |
65.32 ± 0.92E |
0.12 ± 0.01c |
ndc |
— |
17.88 ± 1.27b |
13.87 ± 0.98B |
19.86 ± 0.35G |
17.76 ± 0.58b |
3.91 ± 0.12C |
24.69 ± 0.68F |
C 10:0 |
44.53 ± 0.88a |
65.79 ± 1.28A |
33.9 ± 0.76E |
0.11 ± 0.02c |
nd |
— |
19.02 ± 0.40b |
28.62 ± 1.28B |
14.22 ± 0.28G |
19.31 ± 0.35b |
22.29 ± 0.50C |
17.82 ± 0.25F |
C 20:5 (n-3 EPA) |
nd |
Nd |
— |
62.92 ± 0.18a |
49.8 ± 0.71A |
69.48 ± 0.76E |
36.18 ± 0.26b |
21.21 ± 0.73B |
43.67 ± 1.01F |
35.92 ± 0.32b |
26.93 ± 0.94C |
40.41 ± 0.86G |
C 22:6 (n-3 DHA) |
nd |
Nd |
— |
18.63 ± 0.17a |
11.8 ± 0.76A |
22.05 ± 0.35E |
10.79 ± 0.17b |
4.30 ± 0.64B |
14.04 ± 0.22F |
10.95 ± 0.45b |
7.40 ± 0.30C |
12.73 ± 0.36G |
Others |
2.5 ± 0.03c |
5.94 ± 0.84C |
0.78 ± 0.00G |
18.22 ± 0.04a |
38.4 ± 1.12A |
8.13 ± 0.08E |
16.13 ± 0.42b |
32.00 ± 0.59B |
8.20 ± 0.16E |
16.06 ± 0.05b |
39.47 ± 1.04A |
4.35 ± 0.14F |
Total SFA |
99.91 ± 1.23a |
99.9 ± 1.28A |
99.92 ± 1.12E |
13.8 ± 0.23c |
22.74 ± 1.12D |
9.33 ± 0.05H |
51.74 ± 1.13b |
67.51 ± 1.46B |
43.86 ± 0.56G |
52.98 ± 1.36b |
60.12 ± 1.85C |
49.41 ± 1.05F |
Total UFA |
0.09 ± 0.00c |
0.1 ± 0.97D |
0.08 ± 0.01H |
86.20 ± 1.36a |
77.26 ± 2.21A |
90.67 ± 1.05E |
48.26 ± 1.95b |
32.49 ± 0.89C |
56.14 ± 1.02F |
47.02 ± 1.39b |
39.88 ± 1.02B |
50.59 ± 1.32G |
Total MCFA |
98.61 ± 1.03a |
98.03 ± 0.78A |
98.9 ± 1.04E |
0.53 ± 0.03c |
4.51 ± 0.13D |
0.14 ± 0.01H |
38.8 ± 1.26b |
43.69 ± 1.61B |
36.54 ± 0.73G |
39.86 ± 1.05b |
29.35 ± 0.94C |
45.12 ± 1.12F |
Total LCFA |
1.39 ± 0.02c |
1.97 ± 0.65D |
1.1 ± 0.03H |
99.47 ± 2.42a |
95.49 ± 2.34A |
99.86 ± 1.33E |
61.2 ± 1.29b |
56.31 ± 0.98C |
63.46 ± 1.46F |
60.14 ± 1.42b |
70.65 ± 1.48B |
54.88 ± 1.00G |
As can be seen from Table 2, the major TAGs in MCT were CyCyCy, CaCyCy, CaCaCy and CaCaCa (15.74%, 33.13%, 31.64% and 19.49%, respectively), while EPAEPAEPA, EPAEPADHA, OEPADHA and OPEPA were the major TAGs in FO (45.03%, 38.64%, 2.45% and 2.42%, respectively), which accounted for around 90% of the total TAGs. Though the overall fatty acid composition of PM and MLCT was similar, the TAG molecular species and relative content had clear differences. The TAG molecular species in PM are composed of those in MCT and FO, mainly including CaCyCy, CaCaCy, EPAEPAEPA and EPAEPADHA (14.00%, 15.80%, 21.64% and 19.41%, respectively). Interestingly, a different profile emerged in MLCT. Some less abundant TAGs presented in PM were not detected in MLCT, while some new TAGs (e.g. OLaLa, CyCyEPA and CaCaEPA) were obtained in MLCT by the transesterification reaction. The synthesized product MLCT contained four major groups of TAGs, MMM, LLM (LLM, LML and MLL), MML (MML, MLM and LMM) and LLL (Fig. 1), of which the LLM group (32.81%) and MML group (43.89%) accounted for 76.7%. It is worth mentioning that EPA-containing LLM and MML group accounted for 70.21%, where the top six TAGs were CaCyEPA (15.64%), CaCaEPA (12.56%), CyCyEPA (9.89%), CyEPAEPA (7.64%), CaEPAEPA (7.63%) and CyEPADHA (6.35%). Enzyme-catalyzed transesterification of MCT and FO significantly increased the MML and MLL type TAG content in TAG profiles while significantly decreased the LLL and MMM type TAG content. This demonstrated that the FA rearrangements have occurred in TAG, which may lead to substantial changes in lipid functionality.
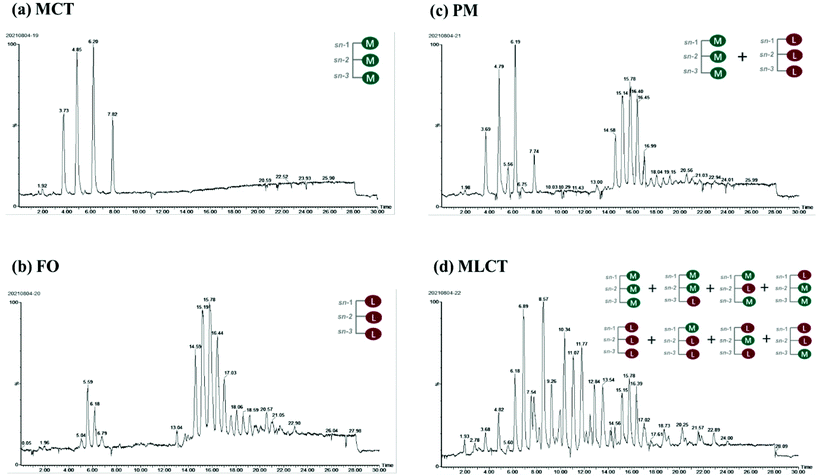 |
| Fig. 1 Total ion current chromatogram of TAGs in four different oils obtained by Q-TOF-MS. M: middle chain fatty acid; L: long chain fatty acid. | |
Table 2 TAG profiles and relative percent (%) of four experimental oils
Triglycerides profiles (%) |
TAG species |
MCT |
FO |
PM (MCT/FO) |
MLCT |
Cy, C8:0; Ca, C10:0; La, C12:0; M, C14:0; P,C16:0; Po, C16:1; O, C18:1; L, C18:2; EPA, C20:5; DPA, C22:5; DHA, C22:6. Values are means ± SD, n = 3. Different superscript lowercase letters indicate significant differences (p < 0.05) in a row. nd, not detected. |
Cy–Cy–Cy |
15.74 ± 0.03a,a |
ndb |
6.77 ± 0.05b |
0.45 ± 0.01c |
Ca–Cy–Cy |
33.13 ± 0.02a |
nd |
14.00 ± 0.04b |
1.69 ± 0.00c |
Ca–Ca–Cy |
31.64 ± 0.00a |
nd |
15.8 ± 0.11b |
3.80 ± 0.02c |
Ca–Ca–Ca |
19.49 ± 0.02a |
nd |
5.10 ± 0.04b |
1.10 ± 0.01c |
O–La–La |
nd |
nd |
nd |
1.36 ± 0.00 |
L–L–La |
nd |
nd |
nd |
0.38 ± 0.02 |
O–M–La |
nd |
nd |
nd |
1.22 ± 0.01 |
O–P–La |
nd |
nd |
nd |
1.31 ± 0.02 |
O–P–M |
nd |
nd |
nd |
0.95 ± 0.00 |
O–P–P |
nd |
nd |
nd |
0.57 ± 0.01 |
Cy–Cy–EPA |
nd |
nd |
nd |
9.89 ± 0.12 |
Ca–Cy–EPA |
nd |
nd |
nd |
15.64 ± 0.04 |
Ca–Cy–DHA |
nd |
nd |
nd |
5.80 ± 0.04 |
Ca–Ca–EPA |
nd |
nd |
nd |
12.56 ± 0.01 |
Cy–EPA–EPA |
nd |
nd |
nd |
7.64 ± 0.03 |
Cy–EPA–DHA |
nd |
nd |
nd |
6.35 ± 0.05 |
Ca–EPA–EPA |
nd |
nd |
nd |
7.63 ± 0.03 |
Cy–EPA–DPA |
nd |
nd |
nd |
5.66 ± 0.01 |
Ca–EPA–DHA |
nd |
nd |
nd |
4.10 ± 0.00 |
Cy–P–EPA |
nd |
nd |
nd |
0.74 ± 0.01 |
Ca–DHA–DHA |
nd |
nd |
nd |
0.69 ± 0.00 |
MOPO |
nd |
0.60 ± 0.01 |
nd |
nd |
MPOEPA |
nd |
0.62 ± 0.01 |
nd |
nd |
OOPO |
nd |
0.60 ± 0.02 |
nd |
nd |
OOP |
nd |
2.08 ± 0.02a |
1.30 ± 0.01b |
nd |
OPL |
nd |
0.65 ± 0.01a |
0.34 ± 0.01b |
nd |
OPEPA |
nd |
2.42 ± 0.02a |
0.95 ± 0.02b |
nd |
OEPAEPA |
nd |
1.74 ± 0.03a |
1.36 ± 0.01b |
nd |
OEPADHA |
nd |
2.45 ± 0.04a |
0.94 ± 0.01b |
nd |
EPAEPAARA |
nd |
1.40 ± 0.01b |
2.44 ± 0.03a |
nd |
EPA–EPA–EPA |
nd |
45.03 ± 0.01a |
21.64 ± 0.02b |
3.62 ± 0.03c |
EPA–EPA–DHA |
nd |
38.64 ± 0.01a |
19.41 ± 0.03b |
4.50 ± 0.05c |
EPA–DHA–DHA |
nd |
1.59 ± 0.03c |
2.30 ± 0.00b |
2.35 ± 0.02a |
EPA–EPA–DPA |
nd |
1.39 ± 0.02b |
6.75 ± 0.01a |
nd |
DHA–EPA–DPA |
nd |
0.79 ± 0.01b |
0.90 ± 0.01a |
nd |
3.2 Changes in physical and structural features of oil emulsions during digestion
The prepared lipid emulsions were passed through an in vitro digestion model consisting of mouth, stomach, and intestinal phases. After each digestion step, the particle size, ζ-potential and the microstructure were measured. These physical and structural characteristics might help to explain the differences in the stability and lipolysis degree during digestion.
Initial samples: Particle size measurements of the lipid emulsions were performed immediately after they were prepared by high-pressure homogenization. Fish oil emulsion exhibited the largest average particle size (0.43 μm), and the other emulsions had relatively small initial mean particle diameters ranging from 0.16 to 0.32 μm (Fig. 2A). The microscopy images indicated that all the initial emulsions were dispersed uniformly and no agglomeration phenomenon occurred (Fig. 2C). These results suggested that the droplet particle sizing with MCT as an oil phase could be smaller compared with FO due to its lower viscosity. Our results were consistent with previous studies that have also reported using LCT instead of MCT resulted in larger droplets by homogenization at higher pressures.26,27 Another possibility may be that the emulsifying ability of Tween 20 to different oils phase may be different.
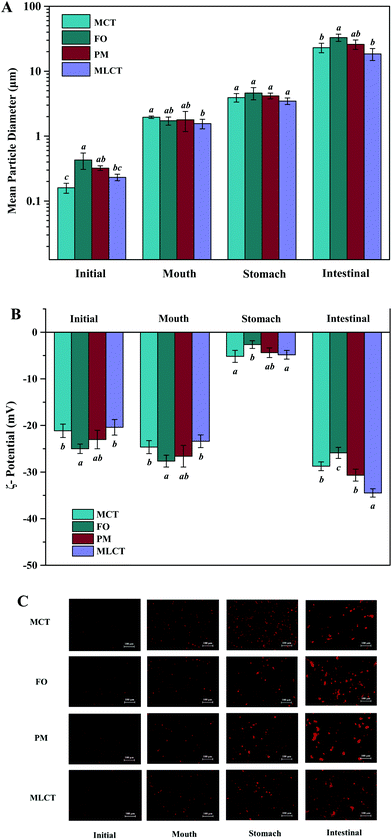 |
| Fig. 2 Influence of oil type on mean particle size (A), ζ-potential (B) and morphology changes (C, the scale bars = 100 μm, eyepiece: 10×, objective lens: 20×) of emulsion samples before and during in vitro simulated digestion (mouth, stomach and small intestine stage). Bars represent the standard deviation of the mean. Different lowercase letters above the bars indicate significant differences (p < 0.05) from different emulsions within the same digestion phase. | |
In the initial emulsions with different oil phases, all the droplets had moderately high negative surface potential (−24.99 to −20.41 mV) (Fig. 2B), which might be caused by some anionic impurities in the Tween 20 surfactant. Non-ionic emulsifier could provide stability by steric repulsion, which is a strong short-range interaction.28 It should be noted that the droplets of different initial emulsions have different electrical charges. This could be due to the presence of impurities in the oil phase. For instance, some cationic or anionic impurities in the oil phase, such as FFAs and mineral ions, were absorbed on the oil–water interface and affect the overall interfacial charge.29 FO used in this study contained significant amounts of free fatty acids, so the surface negative charge density of FO emulsion is higher than that of the other emulsions. Again, TAG profiles and fatty acid compositions were also the reason for the differences among ζ-potential of four oil emulsions.30
Mouth stage: After 2 min of mouth digestion, the mean diameter of particles was observed to have appreciable increase (Fig. 2A), which was supported by the fluorescence microscopy images at this stage (Fig. 2C), indicating that extensive droplet aggregation had occurred. One reason for this phenomenon might be that the addition of porcine stomach mucin promoted bridging or depletion flocculation within the mouth.31 Furthermore, the addition of a large number of salts (Na+, K+, Cl−, PO43−, etc.) also had a detrimental effect on the physical stability, thereby leading to particle flocculation.32 There was also an appreciable increase in negative charge on the droplet surfaces after exposure to the artificial saliva (Fig. 2B), which could be either due to the anionic mucin molecules adsorbed onto the surface of oil droplets, or simply due to the light scattering effect caused by the anionic mucin molecules.33,34
Stomach stage: After the 2 h stomach digestion period, the mean particle diameters were increased for all the emulsion systems (D[3,2] = 3.93, 4.60, 4.17, and 3.47 μm for the MCT, FO, PM and MLCT, respectively, Fig. 2A). In terms of surface potentials, a significant decrease of the negative charge on the droplets occurred after they were incubated in the artificial gastric juice (Fig. 2B), which can be attributed to a reduced pH (pH 2.5) and an increased ionic strength in the gastric environment. In low pH environments, the protonation of anionic groups resulted in the neutralization of negative charge in the solution. In addition, the high ionic strength gastric juice could reduce the negative charges through the electrostatic shielding effect. The lipid droplets may come closer due to a reduced number of the surface charge, thus decreasing their stability against aggregation. This was confirmed by the results of the microscopy images (Fig. 2C). Interestingly, we noted that no positive charge on the droplets was detected under the highly acidic conditions in the stomach, which indicated that more anions were adsorbed on their surface. Another reason could be that Tween 20 is a non-ionic surfactant, which is essentially insensitive to pH variations in the stomach phase, thereby showing higher emulsion stability in the stomach compared to other emulsifiers.34
Small intestine stage: After the simulated small intestine digestion, pancreatic lipases hydrolyze TAGs into FFAs, DAGs and MAGs.34 As a result, there were considerable variations in the structure, composition, and properties of the lipid droplets. Meanwhile, the occurrence flocculation could further change the droplet size and stability.35 The particles in the small intestine stage had the largest mean particle diameter for all four oil systems throughout the simulated digestion process, D[3,2] = 23.07, 32.99, 25.91, and 18.45 μm, respectively (Fig. 2A), which indicated that extensive droplet aggregation had occurred in this stage. The particle size of FO and PM is larger than that of MCT and MLCT, and we speculated that the reason for this phenomenon is large quantities of LCT may form more insoluble calcium soaps. The microstructure of the emulsions also indicated that a large number of droplet aggregations and floccule precipitations occurred in the small intestine stage (Fig. 2C). As the digestion progresses, part of the lipid particles was aggregated together and further clustered into a larger flocculation. These changes may be caused by a variety of reasons, including lipid hydrolysis, droplet flocculation and aggregation, or generation of insoluble deposits. In addition, aggregation may be facilitated by the interactions among the droplets and other components of the small intestinal fluids, such as bile salts and mineral ions.
The lipid droplets in this stage again had more negative surface charge (Fig. 2B), which can be explained by multiple factors.21 First, a large amount of FFAs released during lipid digestion would contribute to the increased net charge of the digestive products. Second, the concentration of negative charge of the surfactant molecules increases with increasing pH. Third, FFAs, anionic phospholipids, and bile acids adsorbed onto the droplet surfaces could result in an increased negative charge. As a consequence, the negative charge on the surface of lipid droplets after the small intestine digestion would increase compared to other digestion stages.36 In the PM oil system, the zeta potential (−30.66 mV) was significantly lower than that of the MCT and FO system (−28.74 and −25.88 mV, respectively). This was because the digestion products from both MCT and FO were included in the PM composite system, and more FFAs were presented, thereby providing more negatively charged molecules to the particles in the system. However, the absolute value of zeta potential of MLCT (−34.48 mV) after digestion was significantly higher than that of PM (p < 0.05). This phenomenon may be interpreted as PM releasing more LCFAs than MLCT during digestion.36 During the digestion, LCFAs tended to accumulate at the oil–water interface, thus leading to a great number of long-chain fatty acid salts becoming enriched at the interface of lipid droplets.37 This eventually resulted in a decrease in the absolute value of zeta potential of PM after digestion and larger oil droplets were observed using a microscope (Fig. 2C).
3.3 Comparison of the digestion properties of four oils during in vitro digestion
3.3.1 Lipolysis degree and lipid composition.
The degree of oil hydrolysis was expressed in terms of FFA content via a step-by-step in vitro digestion process (Fig. 3A). A low lipolysis rate was observed during the mouth and gastric phase due to a lack of gastric lipase. Thus, after 2 min of mouth digestion and 120 min of stomach digestion, the lipolysis degree of the four oils was only 3.42%, 4.61%, 5.10% and 4.92%, respectively. The small intestine is the primary site of lipid digestion, where the four oils are rapidly lipolysed under the action of bile salts and pancreatic lipase. However, there were also some variations between the four oils. MLCT eventually reached a maximum lipolysis rate for about 59.65%, which was remarkably higher than that of the other three oils (p < 0.05). This could be readily explained by the results of fatty acid composition and distribution in Table 1. For MLCT, MCFAs were predominantly distributed in the sn-1,3 positions, and LCFAs mainly existed at the sn-2 position in the TAG structure enhancing the hydrolysis of lipids. In contrast, fish oil contained substantial quantities of long chain omega-3 polyunsaturated fatty acids at the sn-1,3 position, which caused a higher steric hindrance and thus to the lowest hydrolysis rate (35.12%). These results were in accordance with previous reports that demonstrated fatty acid chain length, unsaturation and positional distribution may affect the rate and extent of triglyceride lipolysis.38,39 In addition, some studies have shown that the degree of lipolysis is closely related to the particle size.40 The larger the initial particle size of the emulsion, the lower the micelle formation rate of the digested products during the digestion process, which will lead to the decrease of the hydrolysis rate of the oil.41 This is also one of the reasons why fish oil has the lowest hydrolysis rate.
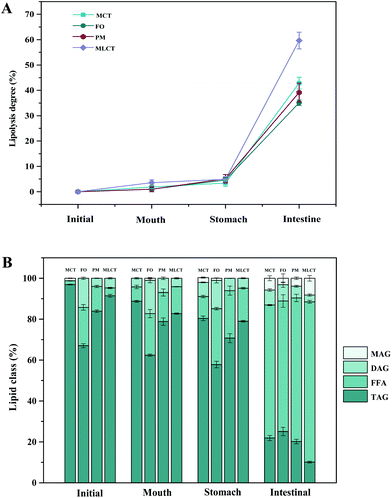 |
| Fig. 3 Lipolysis degree in four oils (A) and lipid composition (B) of four oils digestion products (area percentage) at various stages of in vitro digestion. | |
During in vitro simulated digestion, the changes of lipid class composition of the four different oils were shown in Fig. 3B. In an overall perspective, the changes in lipid class composition of the experimental oils were related to the evolution of lipolysis. In lipid digestion products, the content of TAGs decreased, while the content of FFAs and MAGs increased with lipolysis time. Interestingly, the DAG content increased first and then decreased. Because there was no addition of gastric lipase, only a small amount of these oils is hydrolyzed to lipolysis products after entering the mouth and stomach digestion phase compared with the initial emulsion, and most of them still existed in the form of triglycerides. Thereafter, pancreatic lipase converts TAGs and DAGs into MAGs and FFAs during the intestinal digestion, again leading to the lipolysis product accumulation. Results also suggested that the maximum FFA content was recorded for MLCT (74.55%) after hydrolysis, followed by MCT (63.15%), PM (58.1%), and minimum for FO (46.06%) (Fig. 3B). FO and PM contained large amounts of n-3 PUFAs, which were characterized by highly curved conformation that might have impeded the hydrolyzing effect of lipase. By comparing the contents of DAGs and MAGs in the digestive products of the four oils, it can be found that the DAG content from low to high was MLCT, PM, MCT and FO. Nevertheless, the MAG content was in the following order from low to high: FO < PM < MCT < MLCT. These discrepancies were associated with the specific hydrolytic action of pancreatic lipase. Pancreatic lipase has a high hydrolysis effect on the MCFAs at the sn-1 and sn-3 positions of TAG, but a relatively low hydrolysis effect on the LCFAs at the same position of TAG.13 For MLCT, the highest content of FFAs and MAGs may be due to the relatively sufficient hydrolysis of the sn-1,3 position fatty acids, and LCFAs at the sn-2 position was retained in sn-2 MAG.
3.3.2 FFA release level.
During simulated digestion in the small intestine, a pH-stat was used to monitor the extent and rate of lipid digestion of the four oil systems. The overall trends of digestion curves were similar among the four samples. Within the first several minutes, free fatty acids were released rapidly, followed by a more gradual release, and finally entered a stable stage. These results indicated that the lipase molecules rapidly adhered to the lipid droplet surfaces and began to degrade triacylglycerol molecules. However, the accurate shape of the release rate and release time curves for fatty acid was dictated by the composition of oil phase in the emulsions. As shown in Fig. 4, after 120 min of small intestine digestion, the final FFA release level of the four different lipid phases was in the following order: MLCT (102.79%) > MCT (95.20%) > PM (85.81%) > FO (74.18%). Many studies have confirmed that the digestion process is slightly faster and more extensively in the samples that contain MCT compared with those containing LCT.30,42 This could be because medium chain free fatty acids were dispersed in an aqueous solution more easily than long chain free fatty acids, and could be rapidly removed from the surface of droplets, thereby keeping the lipase to continue performing its effector function.37 On the other hand, long chain free fatty acids might accumulate on the emulsion droplets surface, inhibiting the ability of lipase to further digest the remaining TAGs.43
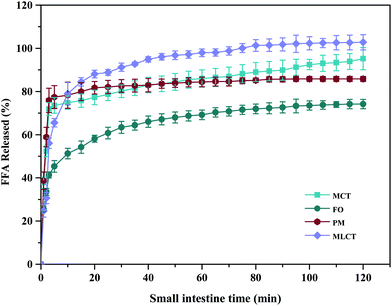 |
| Fig. 4 The calculated percentage of free fatty acids (FFAs) released from emulsions including different oils measured in pH-stat during small intestine digestion. Bars represent the standard deviation of the mean. | |
In addition, because of the steric hindrance effects, the cis-double bonds in polyunsaturated alkenyl chains were hydrolyzed by lipase in a relatively difficult manner.44 Consistently, previous studies had revealed that although pancreatic lipase had the sn-1,3 positional specificity toward the fatty acid triglyceride, the specificity to n-3 PUFAs, especially EPA and DHA, is lower compared with the activity toward other fatty acids when located at the sn-1,3 positions.12,45 In our results, FO contained large quantities of LCFAs, leading to a slower and lesser extent of FFA release. The lower release of free fatty acids resulted in more undigested lipids. However, it is important to note that although MLCT also contained large quantities of LCFAs, the highest FFA release rate was observed. This phenomenon was explained by the fact that after enzymatic transesterification, the LCFAs were more located at the sn-2 position, while the MCFAs were more located at the sn-1,3 position. This has been confirmed in the analysis of sn-2 fatty acids in this work and other researchers have also reported similar results.27 Another reason could be large amounts of MCFA released from MCT might accumulate on the surface of pancreatic lipase, limiting the contact between pancreatic lipase and substrates and resulting in limited MCT lipolysis, which may lead to the lipolysis degree of MCT emulsion being significantly less than that of MLCT.12
3.3.3 The changes of free fatty acid content during digestion.
To further illustrate the lipolysis process of different oil samples, the content of the released FFAs at various stages of digestion was analyzed (Fig. 5). High amounts of MCFAs (C8:0 and C10:0) were released from MCT, which can promote lipid metabolism because these FAs are easily absorbed by the gastrointestinal tract. In addition, octanoic acid (C8:0) was hydrolyzed faster than decanoic acid (C10:0) during the digestion (Fig. 5a). Large amounts of free EPA were present in the initial FO emulsion. However, the concentration of EPA and DPA increased at a slower pace due to the quick hydrolysis of other LCFAs in the first 20 minutes of the small intestine digestion stage. Then, the concentration of EPA and DHA significantly increased (p < 0.05) under the action of pancreatic lipase, which was mainly because EPA is the major component in FO, accounting for about 60% (Fig. 5b). We focused on the comparison of the released FFAs from PM and MLCT. Although the overall fatty acid compositions of these two oils were similar, a noticeable difference of the released FFA profiles was observed during digestion (p < 0.05). Compared with PM, MLCT released greater amount of MCFAs and much less LCFAs (especially EPA) during digestion, which is because MCFAs in MLCT were mainly located at the sn-1,3 positions (Fig. 5c and d). These MCFAs could provide fast energy for the body. In contrast, EPA and other LCFAs at the sn-2 position were absorbed in the form of sn-2 MAG, which pass through cell membranes more easily than the free form of LCFAs.15,46 These results implied that the EPA-rich MLCT synthesized in this study not only had a high degree of lipolysis, but also might promote EPA absorption potential. However, the properties of EPA-rich MLCT have been modified through the enzymatic interesterification of MCT and FO. Thus, it is necessary to re-evaluate its safety before EPA-rich MLCT can be used as functional oils for humans.
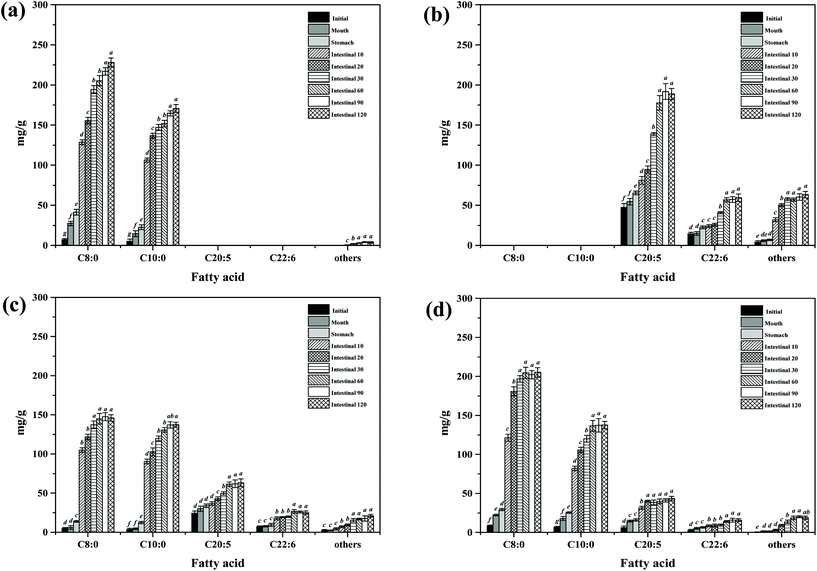 |
| Fig. 5 FFA contents (mg g−1) of different lipid emulsions at various stages of digestion. (a) MCT group; (b) FO group; (c) PM group; (d) MLCT group. Bars represent the standard deviation of the mean, and different lowercase letters above the bars indicate significant differences (p < 0.05) of the same fatty acid at various stages of in vitro digestion. | |
3 Conclusions
In the present study, EPA-rich MLCT was synthesized by enzymatic transesterification, in which the newly synthesized TAG species (MLL and MML) accounted for around 75% of the total TAGs. The distribution of fatty acids in triglycerides indicated that transesterification reactions catalyzed by the immobilized lipase Lipozyme RM IM could increase the relative content of LCFAs at the sn-2 position to 70.65% and reduce the relative content of MCFAs (29.35%) at the sn-2 position, which could lead to substantial changes in lipid functionality. According to the results of an in vitro digestion model, the physical and structural characteristics of different lipid emulsions (MCT, FO, PM and MLCT) were significantly different under the action of pancreatic lipase and bile salts. In addition, MLCT emulsions had a significantly higher hydrolysis degree than the other oils, which indicated that the release behaviour was related not only to the fatty acid species, but also to the position of the fatty acid in the triglyceride. LCFAs (EPA) in MLCT mainly existed in the form of sn-2 MAG, which was conducive to their subsequent absorption and transport. Although it remains impossible to perfectly simulate physiological conditions, the present study can provide some useful information for understanding the digestion process of different oils and offer a valuable reference for further structural design of functional lipids.
Author contributions
Yandan Wang: methodology, data collection and analysis, writing – original draft. Minjie Cao: data collection. Ruijie Liu: revision of the manuscript. Ming Chang: revision of the manuscript. Wei Wei: revision of the manuscript. Qingzhe Jin: supervision, reviewing and editing. Xingguo Wang: conceptualization, validation, supervision.
Conflicts of interest
All authors have declared no conflicts of interest.
Acknowledgements
This work was supported by the National First-Class Discipline Program of Food Science and Technology (JUFSTR20180202) and the National Natural Science Foundation of China (Grant No. 31972037).
References
- M. A. E. de van der Schueren, A. Laviano, H. Blanchard, M. Jourdan, J. Arends and V. E. Baracos, Systematic review and meta-analysis of the evidence for oral nutritional intervention on nutritional and clinical outcomes during chemo(radio)therapy: current evidence and guidance for design of future trials, Ann. Oncol., 2018, 29, 1141–1153 CrossRef CAS.
- Y. D. Wang, T. Zhang, R. J. Liu, M. Chang, W. Wei, Q. Z. Jin and X. G. Wang, New perspective toward nutritional support for malnourished cancer patients: Role of lipids, Compr. Rev. Food Sci. Food Saf., 2021, 20, 1381–1421 CrossRef CAS PubMed.
- N. Schmidt, G. Moller, L. Baeksgaard, K. Osterlind, K. D. Stark, L. Lauritzen and J. R. Andersen, Fish oil supplementation in cancer patients. Capsules or nutritional drink supplements? A controlled study of compliance, Clin. Nutr. ESPEN, 2020, 35, 63–68 CrossRef PubMed.
- Y. Y. Lee, T. K. Tang, E. S. Chan, E. T. Phuah, O. M. Lai, C. P. Tan, Y. Wang, N. A. Ab Karim, N. H. Mat Dian and J. S. Tan, Medium chain triglyceride and medium-and long chain triglyceride: metabolism, production, health impacts and its applications - a review, Crit. Rev. Food Sci. Nutr., 2021, 1–17 Search PubMed.
- Y. Y. Lee, T. K. Tang and O. M. Lai, Health benefits, enzymatic production, and application of medium- and long-chain triacylglycerol (MLCT) in food industries: a review, J. Food Sci., 2012, 77, 137–144 CrossRef.
- M. Zhu and X. Li, Meta-analysis of structured triglyceride versus other lipid emulsions for parenteral nutrition, Nutrition, 2013, 29, 833–840 CrossRef CAS PubMed.
- E. Dierge, E. Debock, C. Guilbaud, C. Corbet, E. Mignolet, L. Mignard, E. Bastien, C. Dessy, Y. Larondelle and O. Feron, Peroxidation of n-3 and n-6 polyunsaturated fatty acids in the acidic tumor environment leads to ferroptosis-mediated anticancer effects, Cell Metab., 2021, 33, 1701–1715 CrossRef CAS.
- K. Stanislaw, Omega-3 fatty acids in modern parenteral nutrition: A review of the current evidence, J. Clin. Med., 2016, 5(3), 34 CrossRef PubMed.
- R. Gorjao, C. M. M. Dos Santos, T. D. A. Serdan, V. L. S. Diniz, T. C. Alba-Loureiro, M. F. Cury-Boaventura, E. Hatanaka, A. C. Levada-Pires, F. T. Sato, T. C. Pithon-Curi, L. C. Fernandes, R. Curi and S. M. Hirabara, New insights on the regulation of cancer cachexia by N-3 polyunsaturated fatty acids, Pharmacol. Ther., 2019, 196, 117–134 CrossRef CAS PubMed.
- R. A. Murphy, M. Mourtzakis, Q. S. Chu, V. E. Baracos, T. Reiman and V. C. Mazurak, Supplementation with fish oil increases first-line chemotherapy efficacy in patients with advanced nonsmall cell lung cancer, Cancer, 2011, 117, 3774–3780 CrossRef CAS.
- G. Pappalardo, A. Almeida and P. Ravasco, Eicosapentaenoic acid in cancer improves body composition and modulates metabolism, Nutrition, 2015, 31, 549–555 CrossRef CAS.
- H. L. Mu and T. Porsgaard, The metabolism of structured triacylglycerols, Prog. Lipid Res., 2005, 44, 430–448 CrossRef CAS.
- H. L. Mu, The digestion of dietary triacylglycerols, Prog. Lipid Res., 2004, 43, 105–133 CrossRef CAS.
- M. C. Michalski, C. Genot, C. Gayet, C. Lopez, F. Fine, F. Joffre, J. L. Vendeuvre, J. Bouvier, J. M. Chardigny and K. Raynal-Ljutovac, Multiscale structures of lipids in foods as parameters affecting fatty acid bioavailability and lipid metabolism, Prog. Lipid Res., 2013, 52, 354–373 CrossRef CAS.
- C. Liu, A. Chen, L. Xu, T. Wang, R. Zhang, J. Xu, Y. Yu, K. Nie, L. Deng and F. Wang, Synthesis of middle-long-middle structured intralipids by biological catalysis and the evaluation of intralipids’ protective effect on liver injury rats, Food Sci. Nutr., 2021, 9, 2381–2389 CrossRef CAS.
- T. L. Yuan, W. Wei, X. G. Wang and Q. Z. Jin, Biosynthesis of structured lipids enriched with medium and long-chain triacylglycerols for human milk fat substitute, LWT—Food Sci. Technol., 2020, 128, 109255 CrossRef CAS.
- Y. Zhang, X. S. Wang, D. Xie, S. Zou, Q. Z. Jin and X. G. Wang, Synthesis and concentration of 2-monoacylglycerols rich in polyunsaturated fatty acids, Food Chem., 2018, 250, 60–66 CrossRef CAS PubMed.
- C. Qi, J. Sun, Y. Xia, R. Q. Yu, W. Wei, J. Y. Xiang, Q. Z. Jin, H. Xiao and X. G. Wang, Fatty Acid Profile and the sn-2 Position Distribution in Triacylglycerols of Breast Milk during Different Lactation Stages, J. Agric. Food Chem., 2018, 66, 3118–3126 CrossRef CAS PubMed.
- T. Zhang, G. Lou, G. J. Tao, R. J. Liu, M. Chang, Q. Z. Jin and X. G. Wang, Composition and structure of single cell oil produced by Schizochytrium limacinum SR31, J. Am. Oil Chem. Soc., 2016, 93, 1337–1346 CrossRef CAS.
- L. Liang, C. Qi, X. Wang, Q. Jin and D. J. McClements, Influence of Homogenization and Thermal Processing on the Gastrointestinal Fate of Bovine Milk Fat: In Vitro Digestion Study, J. Agric. Food Chem., 2017, 65, 11109–11117 CrossRef CAS PubMed.
- B. Ozturk, S. Argin, M. Ozilgen and D. J. McClements, Nanoemulsion delivery systems for oil-soluble vitamins: Influence of carrier oil type on lipid digestion and vitamin D3 bioaccessibility, Food Chem., 2015, 187, 499–506 CrossRef CAS PubMed.
- X. Q. Zou, Z. Guo, J. H. Huang, Q. Z. Jin, L. Z. Cheong, X. G. Wang and X. B. Xu, Human milk fat globules from different stages of lactation: a lipid composition analysis and microstructure characterization, J. Agric. Food Chem., 2012, 60, 7158–7167 CrossRef CAS PubMed.
- T. L. Yuan, Z. W. Geng, X. Y. Dai, X. H. Zhang, W. Wei, X. G. Wang and Q. Z. Jin, Triacylglycerol containing medium-chain fatty acids: Comparison of human milk and infant formulas on lipolysis during In vitro digestion, J. Agric. Food Chem., 2020, 68, 4187–4195 CrossRef CAS PubMed.
- Z. Y. Liu, Y. Y. Hu, M. T. Zhao, H. K. Xie, X. P. Hu, X. C. Ma, J. H. Zhang, Y. H. Bai and D. Y. Zhou, Formation and disappearance of aldehydes during simulated gastrointestinal digestion of fried clams, Food Funct., 2020, 11, 3483–3492 RSC.
- X. Q. Zou, L. Ye, X. He, S. Wu, H. Zhang and Q. Z. Jin, Preparation of DHA-rich medium- and long-chain triacylglycerols by lipase-catalyzed acidolysis of microbial oil from Schizochytrium sp. with medium-chain fatty acids, Appl. Biochem. Biotechnol., 2020, 191, 1294–1314 CrossRef CAS PubMed.
- K. Yao, D. J. McClements, J. Xiang, Z. Zhang, Y. Cao, H. Xiao and X. Liu, Improvement of carotenoid bioaccessibility from spinach by co-ingesting with excipient nanoemulsions: impact of the oil phase composition, Food Funct., 2019, 10, 5302–5311 RSC.
- X. Liu, J. Bi, H. Xiao and D. J. McClements, Increasing carotenoid bioaccessibility from yellow peppers using excipient emulsions: Impact of lipid type and thermal processing, J. Agric. Food Chem., 2015, 63, 8534–8543 CrossRef CAS.
- A. Ashkar, A. Sosnik and M. Davidovich-Pinhas, Structured edible lipid-based particle systems for oral drug-delivery, Biotechnol. Adv., 2021, 107789 CrossRef PubMed.
- R. Zhang, Z. Zhang, H. Zhang, E. A. Decker and D. J. McClements, Influence of lipid type on gastrointestinal fate of oil-in-water emulsions: In vitro digestion study, Food Res. Int., 2015, 75, 71–78 CrossRef CAS.
- Z. Ye, C. Cao, Y. F. Liu, P. R. Cao and Q. Li, Triglyceride structure modulates gastrointestinal digestion fates of lipids: A comparative study between typical edible oils and triglycerides using fully designed in vitro digestion model, J. Agric. Food Chem., 2018, 66, 6227–6238 CrossRef CAS PubMed.
- C. Ritzoulis, S. Siasios, K. D. Melikidou, C. Koukiotis, C. Vasiliadou and S. Lolakos, Interactions between pig gastric mucin and sodium caseinate in solutions and in emulsions, Food Hydrocolloids, 2012, 29, 382–388 CrossRef CAS.
- P. J. Wilde and B. S. Chu, Interfacial & colloidal aspects of lipid digestion, Adv. Colloid Interface Sci., 2011, 165, 14–22 CrossRef CAS.
- M. Yao, Z. Li, D. J. McClements, Z. Tang and H. Xiao, Design of nanoemulsion-based delivery systems to enhance intestinal lymphatic transport of lipophilic food bioactives: Influence of oil type, Food Chem., 2020, 317, 126229 CrossRef CAS.
- Z. Y. Xia, Y. H. Han, H. J. Du, D. J. McClements, Z. H. Tang and H. Xiao, Exploring the effects of carrier oil type on in vitro bioavailability of β-carotene: A cell culture study of carotenoid-enriched nanoemulsions, LWT—Food Sci. Technol., 2020, 134, 110224 CrossRef CAS.
- G. A. van Aken, Relating food emulsion structure and composition to the way it is Pprocessed in the gastrointestinal tract and physiological responses: What are the opportunities?, Food Biophys., 2010, 5, 258–283 CrossRef.
- J. Z. Wang, C. K. Wu, C. H. Yan, H. Chen, S. You, S. Sheng, F. A. Wu and J. Wang, Nutritional targeting modification of silkworm pupae oil catalyzed by a smart hydrogel immobilized lipase, Food Funct., 2021, 12, 6240–6253 RSC.
- H. H. de Abreu-Martins, M. Artiga-Artigas, R. Hilsdorf Piccoli, O. Martin-Belloso and L. Salvia-Trujillo, The lipid type affects the in vitro digestibility and beta-carotene bioaccessibility of liquid or solid lipid nanoparticles, Food Chem., 2020, 311, 126024 CrossRef.
- Q. Guo, A. Ye, N. Bellissimo, H. Singh and D. Rousseau, Modulating fat digestion through food structure design, Prog. Lipid Res., 2017, 68, 109–118 CrossRef CAS.
- A. L. Schoener, R. Zhang, S. Lv, J. Weiss and D. J. McClements, Fabrication of plant-based vitamin D3-fortified nanoemulsions: influence of carrier oil type on vitamin bioaccessibility, Food Funct., 2019, 10, 1826–1835 RSC.
- L. Liang, X. Y. Zhang, X. G. Wang, Q. Z. Jin and D. J. McClements, Influence of dairy emulsifier type and lipid droplet size on gastrointestinal fate of model emulsions: In vitro digestion study, J. Agric. Food Chem., 2018, 66, 9761–9769 CrossRef CAS PubMed.
- D. J. McClements, E. A. Decker and Y. Park, Controlling lipid bioavailability through physicochemical and structural approaches, Crit. Rev. Food Sci. Nutr., 2009, 49, 48–67 CrossRef.
- Y. Li, M. Hu and D. J. McClements, Factors affecting lipase digestibility of emulsified lipids using an in vitro digestion model: Proposal for a standardised pH-stat method, Food Chem., 2011, 126, 498–505 CrossRef CAS.
- R. Devraj, H. D. Williams, D. B. Warren, A. Mullertz, C. J. Porter and C. W. Pouton, In vitro digestion testing of lipid-based delivery systems: calcium ions combine with fatty acids liberated from triglyceride rich lipid solutions to form soaps and reduce the solubilization capacity of colloidal digestion products, Int. J. Pharm., 2013, 441, 323–333 CrossRef CAS PubMed.
- D. A. Gray, G. Payne, D. J. McClements, E. A. Decker and M. Lad, Oxidative stability of echium plantagineum seed oil bodies, Eur. J. Lipid Sci. Technol., 2010, 112, 741–749 CrossRef CAS.
- X. Zhu, A. Ye, T. Verrier and H. Singh, Free fatty acid profiles of emulsified lipids during in vitro digestion with pancreatic lipase, Food Chem., 2013, 139, 398–404 CrossRef CAS PubMed.
- B. H. Kim and C. C. Akoh, Recent research trends on the enzymatic synthesis of structured lipids, J. Food Sci., 2015, 80, 1713–1724 CrossRef PubMed.
|
This journal is © The Royal Society of Chemistry 2022 |