Enhanced doping efficiency and thermoelectric performance of diketopyrrolopyrrole-based conjugated polymers with extended thiophene donors†
Received
28th August 2020
, Accepted 23rd November 2020
First published on 24th November 2020
Abstract
The design of polymer backbones suitable for improving doping efficiency can provide a new direction in enhancing charge transport and thermoelectric performance. However, their optimizations are still unclear. Herein, the synthesis of a new diketopyrrolopyrrole (DPP)-based conjugated polymer with eight thiophene groups in a repeat unit, EHT6-20DPP, was reported for the improvement of thermoelectric properties. Compared to other DPP-based polymers with a different number of thiophene groups, EHT6-20DPP exhibits higher doping efficiency when doped with a p-type dopant, FeCl3, owing to its higher number of thiophene groups as electron donating units. The optimum electrical conductivity and power factor of the doped EHT6-20DPP were found to be 93.28 S cm−1 and 56.73 μW m−1 K−2, which are higher than the reference DPP-based polymer with three thiophene groups in a repeat unit. The relationship between the thermoelectric properties is demonstrated using a charge transport model, suggesting that doped EHT6-20DPP has good charge transport properties in terms of polymer backbone engineering. The results of the present work could provide insights into the optimal polymer backbone design for excellent thermoelectric properties.
Introduction
As global energy use has significantly increased with the development of various electronic products, there has been great interest in energy harvesting research, utilizing wasted energy from industry or human society, converting it to electricity.1 Energy harvesting research is important for maintaining the safety, security, and sustainability of the energy supply.2 Thermoelectric energy harvesters are one of these energy harvesting technologies that convert heat into electrical energy through the Seebeck effect; thus, wasted heat or body temperature is reused more efficiently.3 Currently, although commercialized thermoelectric modules are mainly composed of inorganic materials, there is a fundamental limitation in their application for next-generation electronics, such as flexible or wearable devices, owing to their generally heavy, brittle, expensive, and complicated fabrication processes.4–8 To overcome these limitations, organic, lightweight, flexible, cost-effective, and easily processable thermoelectric materials have garnered significant attention.9–13 Although organic thermoelectric materials have lower thermoelectric performance than conventional inorganic counterparts, they are capable of energy harvesting even at low temperatures (near room temperature), which could satisfy the requirements for wearable electronic devices, electronic skins, and smart sensors operating with low power sources.14–16
Among organic materials, conjugated polymers have recently attracted a lot of attention owing to their high electrical conductivity and solution processability at low temperatures.9,17 Various efforts have been made to enhance the thermoelectric performance of conjugated polymers, which are more focused on enhancing the electrical conductivities because the thermal conductivities of organic materials are basically low.18,19 For the organic materials used in TE studies, the power factor (S2σ) consisting of the relationship between the Seebeck coefficient (S) and electrical conductivity (σ) is mainly represented as the thermoelectric efficiency, instead of the dimensionless figure of merit (ZT), usually expressed as ZT = S2σT/κ, where T and κ are the absolute temperature and thermal conductivity, respectively.20 The power factor of conjugated polymers can be improved after (1) the molecular design of repeat units, (2) structural modification in polymer thin films, and (3) molecular doping with chemical agents, such as acids or small molecules.21–24 These methods control the charge carrier concentration and its transport.21–24 Yan et al. reported an n-type donor–acceptor conjugated polymer containing a pyrazine group in an acceptor unit, showing a σ and power factor that are 20 and 6 times higher, respectively, than that of the polymer backbone converted to thiophene instead of pyrazine.23 In other words, an optimal design of the repeat backbone unit in conjugated polymers is required to increase the doping efficiency and induce favorable crystalline morphology for charge carrier transport. This implies that the selection of effective electron-donating and electron-withdrawing units should be carefully considered to maximize thermoelectric performance in conjugated polymers. The strong electron acceptors in conjugated polymers based on diketopyrrolopyrrole (DPP) have been noticed in organic thermoelectric research owing to their excellent charge transport properties attributed to their high planar structure and electron/hole mobility.23–26 Therefore, a clever strategy to enhance electrical conductivity and thermoelectric performance needs to be suggested by introducing suitable electron-donor structure pairing with the DPP acceptor.
In this study, we reported a novel design of a DPP-based conjugated polymer, EHT6-20DPP (3,3′′′′′-bis(2-ethylhexyl)-2,2′:5′,2′′:5′′,2′′′:5′′′,2′′′′:5′′′′,2′′′′′-sexithiophene-co-2,5-bis(2-octyldodecyl)-3,6-bis(thiophen-2-yl)-2,5-dihydropyrrolo[3,4-c]pyrrole-1,4-dione) comprising eight thiophene groups as electron donating units effectively p-doped by the FeCl3 dopant. The doping efficiency, and electrical, morphological, and thermoelectric properties of the EHT6-20DPP conjugated polymer were investigated in comparison with a typical poly(diketopyrrolopyrrole-terthiophene), PDPP3T with three thiophene groups in a repeat unit. EHT6-20DPP exhibited a maximum σ of 93.28 S cm−1 upon doping with FeCl3, showing 2–3 orders of difference compared to that of PDPP3T even with the similar saturation field-effect mobilities between their transistors in the undoped state. The significant improvement of σ of the doped conjugated polymer contributed to a rise in the power factor of up to 56.7 μW m−1 K−2. In addition, the obtained optical, morphological, and structural properties of the new DPP-based conjugated polymer suggested that the higher thermoelectric performance of EHT6-20DPP was attributable to the high doping efficiency of the extended donating units with eight thiophene groups.
Experimental section
Materials and sample preparation
EHT6-20DPP was synthesized following the procedures described in detail in the ESI.† Poly{2,2-[(2,5-bis(2-hexyldecyl)-3,6-dioxo-2,3,5,6-tetrahydropyrrolo[3,4-c]pyrrole-1,4-diyl)dithiophene]-5,5-diyl-alt-thiophen-2,5-diyl} (PDPP3T) was purchased from Ossila. FeCl3 (97%), chloroform (anhydrous, 99%), acetonitrile (anhydrous, 99.8%), and Triton X-100 were purchased from Sigma-Aldrich. All chemicals were used as received without further purification. The polymers were dissolved in chloroform at a concentration of 10 mg mL−1. The prepared solutions were vigorously stirred for 1 h. The FeCl3 dopant was dissolved in acetonitrile and prepared with specific molarities. The 2 cm × 2 cm glass substrates were cleaned by sequential bath sonication in deionized (DI) water with 1 vol% Triton X-100, acetone, and absolute ethanol. The substrates were then dried and treated with UV–ozone for 20 min. To fabricate the polymer thin film, the prepared polymer solutions were spin-coated onto glass substrates at 1000 rpm for 1 min and then annealed for 10 min on a hot plate to evaporate residual solvents. For the doping process, FeCl3 solutions were sequentially spin-coated onto the as-cast polymer films at 2000 rpm for 20 s. Finally, 100 nm thick Au electrodes were deposited on the polymer films by thermal evaporation using a shadow mask. To prepare the samples for the two-dimensional grazing incident wide-angle X-ray diffraction (2D-GIXD) measurements, 100 nm thick SiO2 layers coated on Si wafers were used. A cleaning process similar to that of the glass substrates was performed. The fabrication steps of the organic thermoelectric devices, except for substrate cleaning, were performed under N2.
Thermoelectric measurements
All thermoelectric properties were analyzed under N2. The sheet resistance was measured by a 4-point probe method with a tip distance of 1 mm using a Keithley 2400 source meter. For the Seebeck coefficients, the polymer films were placed on two Peltier devices and operated with a Keithley 2400 source meter to create a temperature difference between the Peltier sides. The thermovoltages were obtained from a Keithley 2182A nanovoltmeter connected with two probe tips attached to both sides of the Au contact electrodes. The temperatures were measured using two thermocouples connected to a Keithley 2700 multimeter and attached to the polymer films near the 2 contact electrodes. To confirm the reliability, the S value was estimated from a linear plot of the thermovoltage versus temperature difference, S = ΔV/ΔT. The median temperature value between the 2 Peltier devices was maintained at 300 K. The film thickness was determined using a surface profiler (Alpha Step IQ, KLA Tencor).
Organic field-effect transistor (OFET) fabrication
Top-contact bottom-gate OFETs were fabricated from neat EHT6-20DPP and PDPP3T films. Heavily doped Si wafers covered with 100 nm thick SiO2 dielectric layers were prepared as the gate substrate. They were cleaned by piranha treatment, rinsed, and sonicated with DI water multiple times. The substrates were then dried and treated with UV–ozone for 20 min. To form a self-assembled monolayer, the prepared substrates were dipped into the octadecyltrichlorosilane (ODTS) solution (1 vol% in toluene) for 1 h. The polymer solutions were spin-coated onto the ODTS-treated substrates and thermally annealed for 10 min. For the fabrication of the source/drain electrodes, Au layers (100 nm) were deposited onto the polymer films by thermal evaporation through a shadow mask with channel widths (W) and lengths (L) of 300 and 100 μm, respectively. The electrical properties of the OFETs were measured using a Keithley 4200-SCS parameter analyzer. All OFET characterizations were performed under a N2 atmosphere.
Other characterizations
Ultraviolet-visible-near infrared (UV-vis-NIR) absorption spectra were obtained using a JASCO V670 spectrophotometer. A cyclic voltammogram (CV) graph of a three-electrode system was obtained using Autolab PGSTAT302N, consisting of Pt, a Pt sheet, and Ag/AgCl electrodes, which operated as the working, counter and reference electrodes, respectively. 0.1 M Bu4NPF6 solution in acetonitrile was utilized as the electrolyte. For the measurement, the polymer films were prepared by dipping the working electrode into the polymer solution. Subsequently, a small amount of ferrocene was added to the electrolyte to obtain standard redox potential peaks. The average molecular weight (Mn) and weight average molecular weight (Mw) were measured using a polystyrene standard and a chloroform solvent by Waters GPC 2414. 2D-GIXD data were obtained using a high-resolution synchrotron X-ray beam source with an incident X-ray angle of approximately 0.2°, which was performed on the 3 C beamline of the Pohang Accelerator Laboratory, Republic of Korea. Atomic force microscopy (AFM) measurements were conducted using the dynamic noncontact mode (XE-150, Park systems).
Results and discussion
The structure of EHT6-20DPP is shown in Fig. 1(a). The polymer was synthesized via Stille coupling from 2,5-bis(2-octyldodecyl)-3,6-bis(5-(trimethylstannyl)thiophen-2-yl)-2,5-dihydropyrrolo[3,4-c]pyrrole-1,4-dione and 5,5′′′′′-dibromo-3,3′′′′′-bis(2-ethylhexyl)-2,2′:5′,2′′:5′′,2′′′:5′′′,2′′′′:5′′′′,2′′′′′ and sexithiophene that was obtained from bromination, Yamamoto coupling, and Suzuki coupling reactions. The full synthetic scheme and the detailed experimental procedure are described in the ESI.† The structures of the intermediate compounds, monomer and polymer were confirmed by 1H-Nuclear Magnetic Resonance (NMR), 13C-NMR, and mass spectroscopy (Fig. S1–S13, ESI†). The polymer had good solubility in common organic solvents and exhibited a number average molecular weight
of 22 kDa with a polydispersity index of 1.75, obtained by gel permeation chromatography (Fig. S14, ESI†). Tables S1 and S2 (ESI†) summarize the physical properties and material characteristics of the synthesized EHT6-20DPP. The highest occupied molecular orbital level (EHOMO) of EHT6-20DPP was estimated to be −5.13 eV from the CV plot (Fig. 1(b)). PDPP3T was selected as the reference polymer semiconductor material with the structure and CV plot shown in Fig. S15 (ESI†). Considering the decreased potential of FeCl3,27 the higher EHOMO of the EHT6-20DPP is expected to be more favorable for FeCl3 doping compared to PDPP3T (EHOMO = −5.34 eV). In other words, a shallow HOMO level allows the EHT6-20DPP to donate electrons easily to the p-type dopant, indirectly indicating higher doping efficiency. The thermal stabilities of the EHT6-20DPP were further characterized by thermogravimetric analysis and differential scanning calorimetry (Fig. S16, ESI†). EHT6-20DPP had a 5% decomposition temperature (Td) of 388 °C, and melting (Tm) and crystallization temperature (Tc) of approximately 223 and 208 °C, respectively. These results suggest that EHT6-20DPP exhibited highly crystalline structures with good thermal stability. Fig. 1(c) shows the UV-vis-NIR absorption spectra obtained from the samples including (1) EHT6-20DPP solution and (2) spin-cast EHT6-20DPP thin film, respectively. The maximum absorption intensity of the EHT6-20DPP thin film was red shifted with respect to that of the solution spectrum, which may be attributed to the enlarged charge delocalization caused by strong intermolecular interactions and π-orbital overlapping between planarized polymer backbones.28
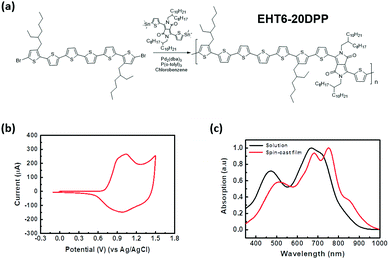 |
| Fig. 1 Characterization of synthesized EHT6-20DPP. (a) Synthetic route for EHT6-20DPP, (b) cyclic voltammogram, and (c) UV-vis spectra of EHT6-20DPP given at the solution and thin film state. | |
UV-vis-NIR spectroscopy was implemented to determine the optical properties of the EHT6-20DPP and PDPP3T polymer films. As shown in Fig. 2(a) and (b), the relative doping levels of both EHT6-20DPP and PDPP3T were controlled by varying the dopant concentration. When the polymer films were treated with FeCl3 dopants, the absorbances of EHT6-20DPP and PDPP3T polymers decreased at 762 and 770 nm, but increased at 1150, 1426, and 2000. These changes were attributed to the polaron formation resulting from the oxidation of the polymers by FeCl3 dopants.9,29 Because doping level is proportional to dopant concentration, the degree of peak changes increases with increasing FeCl3 concentration. In other words, when the doping concentration was increased, neutral polymer peaks (λ762
nm and λ770
nm) decreased and the oxidized polymer peaks (λ1150
nm, λ1426
nm, and >λ2000
nm) increased. Finally, λ1150
nm, λ1426
nm, and >λ2000
nm and the doping of polymers became saturated when the dopant concentration reached 16 mM (EHT6-20DPP) and 14 mM (PDPP3T) (Fig. 2(a) and (b)). The absorbance ratio between the oxidized and neutral forms was calculated to quantitatively analyze the polymer doping with FeCl3, as shown in Fig. 2(c) and (d) for EHT6-20DPP PDPP3T, respectively. The comparison of the absorbance intensity at λ1150
nm over λ762
nm (I1150
nm/I762
nm) for EHT6-20DPP and that at λ1426
nm over λ770
nm (I1426
nm/I770
nm) for PDPP3T showed how much the dopant affects the polymers.24 Although each polymer had a different increased ratio form, I1150
nm/I762
nm of EHT6-20DPP was higher than that of PDPP3T at a given dopant concentration range (4–18 mM). Because EHT6-20DPP has more thiophene groups than PDPP3T in each repeating unit, electron donation from EHT6-20DPP to FeCl3 was preferred, leading to more p-doping. Thus, EHT6-20DPP exhibited a higher doping efficiency than PDPP3T at equal dopant concentration. In fact, it could be estimated that the charge density values of EHT6-20DPP films were 1.94 × 1020 cm−3 at 10 mM FeCl3 doping and 2.63 × 1020 cm−3 at 16 mM. The charge density values of PDPP3T films showed 1.65 × 1020 cm−3 at 10 mM, and 2.20 × 1020 cm−3 at 16 mM, respectively (see details in Fig. S17 and S18, ESI†).
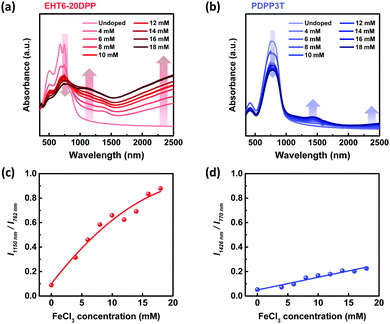 |
| Fig. 2 Absorption characteristics of polymers doped by FeCl3. UV-vis-NIR spectra show the (a) EHT6-20DPP and (b) PDPP3T films at various FeCl3 doping ratios. The ratio between the oxidized and neutral UV-vis intensity peaks of (c) EHT6-20DPP and (d) PDPP3T films by dopant concentration. | |
The carrier density of EHT6-20DPP was larger than that of PDPP3T, because of its high doping efficiency due to increased thiophene groups in a repeat unit. When doped at 10 mM, we estimated from the charge density, film density, and molar mass of repeating units that EHT6-20DPP produced 0.45 number of free charge carrier generated per repeating unit, whereas 0.16 number of free charge carrier was generated per repeating unit of PDPP3T. The doping behavior of π-conjugated polymers affected the electrical and thermoelectric properties of the material, with doping changing the mobile charge carrier densities. Fig. 3(a) shows the electrical conductivity of p-doped EHT6-20DPP and PDPP3T thin films in terms of the FeCl3 dopant concentration (4–18 mM). The electrical conductivity σ was determined using the sheet resistance and thickness of each sample. The thicknesses of EHT6-20DPP films were 93.9 ± 22.8 nm and 70.5 ± 20.4 nm before and after an optimized FeCl3 doping (10 mM), respectively, whereas the PDPP3T films showed 81.5 ± 10.2 nm and 88.3 ± 6.05 nm, respectively. The thickness of each sample depending on the doping concentration of FeCl3 is summarized in Table S3 (ESI†). The electrical conductivity of EHT6-20DPP increased from 1.55 S cm−1 at a FeCl3 concentration of 4 mM to 93.3 S cm−1 at 10 mM whereas that of PDPP3T also increased from 0.00174 S cm−1 at 6 mM to 0.433 S cm−1 at 16 mM, after which the conductivities were saturated. For PDPP3T doped with FeCl3 at a concentration of 4 mM, the film sheet resistance was too high to measure the conductivity. These results correspond to the doping efficiency previously identified in Fig. 2(c) and (d).30,31 Because doping efficiency was higher at a given dopant concentration, EHT6-20DPP exhibited a higher electrical conductivity than PDPP3T. Notably, OFETs prepared using both EHT6-20DPP and PDPP3T polymer semiconductors (without FeCl3) exhibited a hole μFET of 0.18 cm2 V−1 s−1 (inset of Fig. 3(a)) with a minimal difference in the saturation currents at a VG of −30 V (Fig. S19, ESI†). In other words, the hole-transport properties of the EHT6-20DPP thin film were similar to those of PDPP3T without any doping. However, when doped with FeCl3, the electrical conducting properties of both polymers with different numbers of thiophene groups in a repeating unit differed. The higher electrical conductivity of EHT6-20DPP than PDPP3T at a given doping concentration can be attributed to its higher charge carrier density. Electrical conductivity can be expressed by σ = enμ, where σ, e, n, and μ are the electrical conductivity, unit charge, charge carrier density, and charge carrier mobility, respectively. Considering this equation and the UV-vis-NIR spectra shown in Fig. 2, more polaron formation resulting from the oxidation of EHT6-20DPP by FeCl3 increased the charge carrier densities and electrical conductivity.
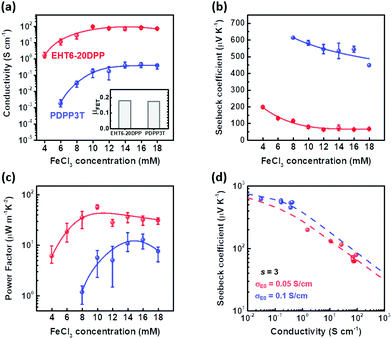 |
| Fig. 3 Thermoelectric properties of the polymers doped with FeCl3: (a) electrical conductivity, (b) Seebeck coefficient, (c) power factor, and (d) empirical plots of S–σ using the Kang–Snyder charge transport model (dashed lines). Inset figure shows the similar field effect mobility (μFET) of both undoped polymers. | |
Fig. 3(b) and (c) show the Seebeck coefficient and thermoelectric power factor of the EHT6-20DPP and PDPP3T films, respectively, which were calculated from σS2, where S is the Seebeck coefficient. The Seebeck coefficient plots showed a trend opposite to that of electrical conductivity. As FeCl3 doping concentration increased, the Seebeck coefficient of EHT6-20DPP decreased from 190 μV K−1 at an FeCl3 concentration of 4 mM to 60 μV K−1 at 16 mM, whereas that of the PDPP3T sample decreased from 613 μV K−1 at 8 mM to 448 μV K−1 at 18 mM; both became nearly saturated after 12 mM doping. In PDPP3T films doped with 4 and 6 mM FeCl3, the sheet resistances were too high to measure the Seebeck coefficient. In hopping transport-dominant conjugated polymers, the Seebeck coefficient can be expressed by:32,33
where
Etr is the transport level and
EF is the Fermi level. When the doping level is increased,
EF approached
Etr, which is located near the HOMO level. The Seebeck coefficient is then decreased by reducing the energy level difference between
Etr and
EF.
34,35 In this regard, finding a moderate doping level is important to obtain the optimal power factors as conductivity and the Seebeck coefficient having a trade-off relationship with doping. At a given FeCl
3 concentration of 10 mM, EHT6-20DPP has a power factor of 56.7 μW m
−1 K
−2, which is higher than that of PDPP3T (5.61 μW m
−1 K
−2) (
Table 1). The maximum power factor (12.8 μW m
−1 K
−2) of PDPP3T at an FeCl
3 concentration of 16 mM was still less than that of the EHT6-20DPP sample. As previously mentioned, these tendencies indicate higher doping efficiency of EHT6-20DPP due to more thiophene groups in each repeating unit. The relationship between the Seebeck coefficient and electrical conductivity (
S–σ) in conducting polymers can be analyzed using the charge transport model.
38 which proposed a transport function
σE(
E,
T), given as follows:
| 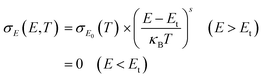 | (2) |
where
σE0(
T) is the transport coefficient,
Et is the transport edge, and
s is the transport parameter. The transport edge refers to the transport energy of charge carriers. The transport parameter is generally obtained from the charge transport type; in this equation,
s of the conducting polymer is fixed at 3. The migration of the charge carriers can be induced by the temperature gradient described by the Seebeck coefficient. In addition, the Seebeck coefficient can be expressed by the transport function of the energy of each carrier. Therefore, the electrical conductivity and Seebeck coefficient are related as transport functions.
22,36Fig. 3(d) shows the experimental values of the Seebeck coefficient
versus the electrical conductivity of FeCl
3-doped EHT6-20DPP (red) and PDPP3T (blue). Although we tried to increase the FeCl
3 concentration for obtaining high electrical conductivity in PDPP3T films, all the experimental values were measured below 1 S cm
−1 due to the electrical conductivity and doping saturation. The optimal transport coefficients (
σE0) of each polymer were determined by fitting the values according to the charge transport model (dashed line). The fitted curve in PDPP3T was located above EHT6-20DPP, indicating higher Seebeck coefficients at given electrical conductivities. This behavior can be seen in detail through the transport coefficient; PDPP3T (
σE0 = 0.1 S cm
−1) had a higher value than EHT6-20DPP (
σE0 = 0.05 S cm
−1). In other words, PDPP3T had high theoretical thermoelectric parameters. However, the actual experiment revealed that EHT6-20DPP had a higher power factor than PDPP3T at a given dopant concentration. PDPP3T is limited by its thermoelectric performance because of its low doping efficiency. Further study on the effects of doping on the crystal domains of both semiconductors should be implemented.
Table 1 Electrical and thermoelectric properties of EHT6-20DPP and PDPP3T with 10 mM FeCl3 doping
Polymer |
Undoped OFETs mobility (cm2 V−1 s−1) |
Conductivity (S cm−1) |
Seebeck coefficient (μV K−1) |
Power factor (μW m−1 K−2) |
EHT6-20DPP |
0.180 |
93.3 ± 7.53 |
78.0 ± 8.34 |
56.7 ± 4.58 |
PDPP3T |
0.175 |
0.166 ± 0.0989 |
582 ± 14.7 |
5.61 ± 2.66 |
To investigate the relationship between the thermoelectric properties and crystal structures of EHT6-20DPP with doping, 2D-GIXD measurements were implemented. Fig. 4(a) and (b) show the 2D-GIXD patterns of EHT6-20DPP thin films in their undoped and doped states, respectively. The patterns show that both EHT6-20DPP with and without doping exhibit (l00) diffraction peaks in the out-of-plane (qz) directions, indicating the well-ordered crystal stacking with lamellar chain formation.37–39 Moreover, (010) diffraction peaks were also observed in the in-plane (qxy) direction of both samples, representing π–π stacking in both states. Generally, EHT6-20DPP thin films are expected to prefer an edge-on molecular orientation with well-ordered lamellar domains regardless of the application of the doping process. The anisotropic charge transport in the edge-on oriented polymer semiconductor was considered to be favorable for planar charge transport between polymer chains, exhibiting high thermoelectric performances.38 Normalized cross-sectional intensity profiles would be helpful in determining the interchain distance (d-spacing) of EHT6-20DPP in the qxy and qz directions. From the 2D-GIXD patterns, the extracted intensity profiles in the qxy and qz directions are shown in Fig. 4(c) and (d), respectively. The d-spacing can be calculated by 2π/q, where q is the diffraction peak position. Because d-spacing and q are inversely proportional, the high peak position in the profile corresponds to a low d-spacing value. The d-spacing of the (100) planes (d100) in the EHT6-20DPP thin film was calculated to be 17.9 Å in the undoped state and 18.7 Å in the doped state. The increase in d-spacing after FeCl3 doping indicates that FeCl3 was incorporated between the lamellar domains of EHT6-20DPP. However, FeCl3 doping of the EHT6-20DPP thin film reduced the d-spacing of the (010) planes (d010) from 3.81 Å (undoped state) to 3.67 Å (doped state). This decrease corresponded to the close π–π stacking distance between each EHT6-20DPP, implying (1) planarization of the polymer backbone after doping or (2) compact packing of the polymer resulting from coulombic interactions between the oxidized polymers and active dopants.22,40 The effects of doping concentration on the crystal structures (2D-GIXD patterns, cross-sectional intensity profiles, and d-spacings) of the EHT6-20DPP thin films are shown in Fig. S20 (ESI†). As expected, d100 of EHT6-20DPP tended to increase (from 18.0 Å (4 mM) to 18.9 Å (18 mM)), but d010 decreased (from 3.77 Å (4 mM) to 3.65 Å (18 mM)) with increasing dopant concentration. These results suggest the number of dopants that change the crystal structure by directly interacting with EHT6-20DPP domains increased as the FeCl3 concentration increased. Comparing the structural analyses of EHT6-20DPP thin films with those of PDPP3T, the 2D-GIXD pattern of PDPP3T exhibited a clear π–π stacking (010) peak in the qz direction and a (l00) peak in the qxy direction, indicating face-on molecular orientations with less-ordered lamellar domains (see Fig. S21, ESI†). Furthermore, the cross-sectional intensity profiles revealed the minimal change in the diffraction peak position values in both directions as the dopant concentration increased. These characteristic PDPP3T crystals may be more effective for thermoelectric performance as there was no change in d100 upon doping; however, this also resulted in less dopant incorporation between the polymers, which induced poor doping efficiency. We inferred that it would be difficult to incorporate the dopant into the PDPP3T crystalline form obtained from the acetonitrile solvent, and it would only be able to enter the amorphous regions.41 Thus, the changes in the crystal orientation in EHT6-20DPP thin films, where d-spacing increased in the qz direction but decreased in qxy, could lead to more efficient charge transport due to the close π–π stacking distance.
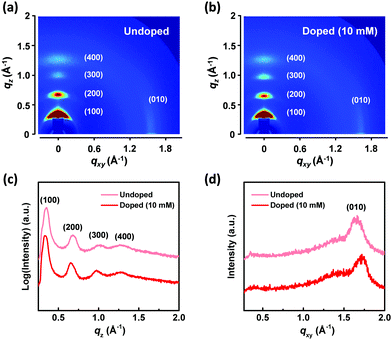 |
| Fig. 4 Crystallographic analysis of EHT6-20DPP films with FeCl3 doping. 2D-GIXD patterns of the (a) undoped and (b) doped EHT6-20DPP, and their cross-sectional intensity profiles in the (c) out-of-plane and (d) in-plane direction. | |
Both polymer semiconductors were further compared by investigating their surface morphologies using AFM as shown in Fig. 5. The EHT6-20DPP thin film maintained its surface morphology even after doping with FeCl3 at concentrations of 10, 16, and 18 mM. In the case of the PDPP3T films (Fig. 5(b)), similar surface morphology was observed at low dopant concentrations (10 mM); however, spherical aggregates were noted after doping with high FeCl3 concentration (≥16 mM). The creation of these aggregates was expected to result from the poor solubility of polar dopants in the PDPP3T matrix.42–44 Given that the crystal arrangement of PDPP3T did not significantly change after the doping process, excessive FeCl3 molecules could not contribute to doping but only formed inactive dopant aggregates on the PDPP3T surface. In contrast, excessive dopants with high FeCl3 concentration could also permeate the EHT6-20DPP crystals, thereby actively participating in doping. AFM and 2D-GIXD analyses indicated the superior doping efficiency of EHT6-20DPP compared to PDPP3T, where the FeCl3 dopant effectively incorporated EHT6-20DPP crystal domains. Collectively, these results suggest that a structural design for improving doping efficiency can be one of the key factors for increasing electrical conductivities and thermoelectric properties.
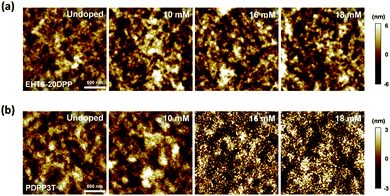 |
| Fig. 5 Surface morphologies of the polymer films with various dopant concentrations. AFM topographic images of (a) EHT6-20DPP and (b) PDPP3T films. | |
Conclusions
We designed a novel DPP-based conjugated polymer comprising eight thiophene groups in a repeat unit, EHT6-20DPP, and achieved good charge transport properties using a sequential doping method. In comparison with PDPP3T, including with three thiophene groups, EHT6-20DPP had a relatively shallow HOMO level with extended conjugation from eight thiophene groups in a repeat unit. UV-vis-NIR spectroscopy revealed that the EHT6-20DPP exhibited higher doping efficiency than PDPP3T owing to the greater number of thiophene groups as electron donating units and effective p-doping via the FeCl3 dopant. 2D-GIXD and AFM analyses were performed to investigate the effects of the FeCl3 dopant on the crystalline morphologies of the EHT6-20DPP films. The results showed that the π–π stacking distance of the EHT6-20DPP decreased, and the surface morphologies in the doped state were maintained. Additionally, the FeCl3 dopant was efficiently incorporated with EHT6-20DPP, which improved the charge transportation. In contrast, the doped PDPP3T barely changed the interchain distance but included inactive dopant aggregates in the film. Consequently, the EHT6-20DPP obtained an optimized power factor of 56.7 μW m−1 K−2 at 10 mM FeCl3 concentration. The thermoelectric properties of both polymers corresponded to the Kang–Snyder charge transport model. Based on our findings, we believe that this study could provide insight into a novel polymer backbone design for outstanding thermoelectric properties with good doping efficiency.
Conflicts of interest
There are no conflicts to declare.
Acknowledgements
This work was supported by a National Research Foundation (NRF) grant (2018R1A2A1A05078734) and the Korea Institute of Energy Technology Evaluation and Planning (KETEP 20173010013000). This work was also supported by the National R&D Program through the National Research Foundation of Korea (NRF) funded by the Ministry of Science and ICT (2020M3F3A2A01085792) and by the Korea Institute of Energy Technology Evaluation and Planning (KETEP) and the Ministry of Trade, Industry & Energy (MOTIE) of the Republic of Korea (No. 20192010106790).
Notes and references
-
L. Mateu and F. Moll, presented in part at the Microtechnologies for the New Millenium, Sevilla, Spain, 9–11 May 2005.
- H. Wang, A. Jasim and X. Chen, Appl. Energy, 2018, 212, 1083–1094 CrossRef.
- V. Leonov, IEEE Sens. J., 2013, 13, 2284–2291 Search PubMed.
- R. Kroon, D. A. Mengistie, D. Kiefer, J. Hynynen, J. D. Ryan, L. Yu and C. Müller, Chem. Soc. Rev., 2016, 45, 6147–6164 RSC.
- Y. J. Jeong, D.-J. Yun, S. H. Noh, C. E. Park and J. Jang, ACS Nano, 2018, 12, 7701–7709 CrossRef CAS.
- H. W. Ban, J. G. Oh, S. Jo, H. Jeong, D. H. Gu, S. Baek, S. Y. Lee, Y. I. Park, J. Jang and J. S. Son, Chem. Mater., 2019, 31, 5909–5918 CrossRef CAS.
- Y. J. Jeong, D.-J. Yun, S. Nam, E. H. Suh, C. E. Park, T. K. An and J. Jang, Org. Electron., 2016, 33, 40–47 CrossRef CAS.
- B. Russ, A. Glaudell, J. J. Urban, M. L. Chabinyc and R. A. Segalman, Nat. Rev. Mater., 2016, 1, 1–14 Search PubMed.
- E. H. Suh, Y. J. Jeong, J. G. Oh, K. Lee, J. Jung, Y. S. Kang and J. Jang, Nano Energy, 2019, 58, 585–595 CrossRef CAS.
- Y. Jo, J. G. Oh, C. Kim, T. K. An, J. Jang and J. Lee, J. Ind. Eng. Chem., 2020, 85, 111–117 CrossRef.
- Y. H. Ha, J. G. Oh, S. Park, S.-K. Kwon, T. K. An, J. Jang and Y.-H. Kim, Org. Electron., 2018, 63, 250–256 CrossRef CAS.
- M.-K. Jeong, K. Lee, J. Kang, J. Jang and I. H. Jung, New J. Chem., 2020, 86, 150–157 Search PubMed.
- A. Weathers, Z. U. Khan, R. Brooke, D. Evans, M. T. Pettes, J. W. Andreasen, X. Crispin and L. Shi, Adv. Mater., 2015, 27, 2101–2106 CrossRef CAS.
- Y. J. Jeong, J. Jung, E. H. Suh, D. J. Yun, J. G. Oh and J. Jang, Adv. Funct. Mater., 2019, 30, 1905809 CrossRef.
- G. Chen, W. Xu and D. Zhu, J. Mater. Chem. C, 2017, 5, 4350–4360 RSC.
- J. Jung, E. H. Suh, Y. J. Jeong, H. S. Yang, T. Lee and J. Jang, ACS Appl. Mater. Interfaces, 2019, 11, 47330–47339 CrossRef CAS.
- O. Bubnova and X. Crispin, Energy Environ. Sci., 2012, 5, 9345–9362 RSC.
- Y. Xue, C. Gao, L. Liang, X. Wang and G. Chen, J. Mater. Chem. A, 2018, 6, 22381–22390 RSC.
- Q. Zhang, Y. Sun, W. Xu and D. Zhu, Adv. Mater., 2014, 26, 6829–6851 CrossRef CAS.
- L. M. Cowen, J. Atoyo, M. J. Carnie, D. Baran and B. C. Schroeder, ECS J. Solid State Sci. Technol., 2017, 6, N3080 CrossRef CAS.
- L. Wang, C. Pan, A. Liang, X. Zhou, W. Zhou, T. Wan and L. Wang, Polym. Chem., 2017, 8, 4644–4650 RSC.
- J. Lee, J. Kim, T. L. Nguyen, M. Kim, J. Park, Y. Lee, S. Hwang, Y.-W. Kwon, J. Kwak and H. Y. Woo, Macromolecules, 2018, 51, 3360–3368 CrossRef CAS.
- X. Yan, M. Xiong, J. T. Li, S. Zhang, Z. Ahmad, Y. Lu, Z. Y. Wang, Z. F. Yao, J. Y. Wang, X. Gu and T. Lei, J. Am. Chem. Soc., 2019, 141, 20215–20221 CrossRef CAS.
- I. H. Jung, C. T. Hong, U. H. Lee, Y. H. Kang, K. S. Jang and S. Y. Cho, Sci. Rep., 2017, 7, 44704 CrossRef CAS.
- Y. Karpov, T. Erdmann, I. Raguzin, M. Al-Hussein, M. Binner, U. Lappan, M. Stamm, K. L. Gerasimov, T. Beryozkina, V. Bakulev, D. V. Anokhin, D. A. Ivanov, F. Gunther, S. Gemming, G. Seifert, B. Voit, R. Di Pietro and A. Kiriy, Adv. Mater., 2016, 28, 6003–6010 CrossRef CAS.
- S. F. Yang, Z. T. Liu, Z. X. Cai, M. J. Dyson, N. Stingelin, W. Chen, H. J. Ju, G. X. Zhang and D. Q. Zhang, Adv. Sci., 2017, 4, 1700048 CrossRef.
- Z. Liang, Y. Zhang, M. Souri, X. Luo, A. M. Boehm, R. Li, Y. Zhang, T. Wang, D.-Y. Kim, J. Mei, S. R. Marder and K. R. Graham, J. Mater. Chem. A, 2018, 6, 16495–16505 RSC.
- H. J. Cheon, X. Li, Y. J. Jeong, M. J. Sung, Z. Li, I. Jeon, X. Tang, H. G. Girma, H. Kong, S.-K. Kwon, T. K. An, S. H. Kim and Y.-H. Kim, J. Mater. Chem. C, 2020, 8, 8410–8419 RSC.
- M. Skompska, A. Szkurlat, A. Kowal and M. Szklarczyk, Langmuir, 2003, 19, 2318–2324 CrossRef CAS.
- C. T. Hong, W. Lee, Y. H. Kang, Y. Yoo, J. Ryu, S. Y. Cho and K.-S. Jang, J. Mater. Chem. A, 2015, 3, 12314–12319 RSC.
- T.-C. Chung, J. Kaufman, A. Heeger and F. Wudl, Phys. Rev. B: Condens. Matter Mater. Phys., 1984, 30, 702 CrossRef CAS.
- J. Liu, G. Ye, B. V. D. Zee, J. Dong, X. Qiu, Y. Liu, G. Portale, R. C. Chiechi and L. J. A. Koster, Adv. Mater., 2018, 30, 1804290 CrossRef.
- A. Kahn, Mater. Horiz., 2016, 3, 7–10 RSC.
- R. Schmechel, J. Appl. Phys., 2003, 93, 4653–4660 CrossRef CAS.
- T. Menke, D. Ray, J. Meiss, K. Leo and M. Riede, Appl. Phys. Lett., 2012, 100, 093304 CrossRef.
- S. Dongmin Kang and G. Jeffrey Snyder, Nat. Mater., 2017, 16, 252–257 CrossRef CAS.
- D. T. Duong, C. Wang, E. Antono, M. F. Toney and A. Salleo, Org. Electron., 2013, 14, 1330–1336 CrossRef CAS.
- G. P. Kini, Q. V. Hoang, C. E. Song, S. K. Lee, W. S. Shin, W.-W. So, M. A. Uddin, H. Y. Woo and J.-C. Lee, Polym. Chem., 2017, 8, 3622–3631 RSC.
- J. Rivnay, S. C. Mannsfeld, C. E. Miller, A. Salleo and M. F. Toney, Chem. Rev., 2012, 112, 5488–5519 CrossRef CAS.
- J. Hynynen, D. Kiefer, L. Yu, R. Kroon, R. Munir, A. Amassian, M. Kemerink and C. Muller, Macromolecules, 2017, 50, 8140–8148 CrossRef CAS.
- I. E. Jacobs, E. W. Aasen, J. L. Oliveira, T. N. Fonseca, J. D. Roehling, J. Li, G. Zhang, M. P. Augustine, M. Mascal and A. J. Moule, J. Mater. Chem. C, 2016, 4, 3454–3466 RSC.
- J. Liu, L. Qiu, R. Alessandri, X. Qiu, G. Portale, J. Dong, W. Talsma, G. Ye, A. A. Sengrian, P. C. T. Souza, M. A. Loi, R. C. Chiechi, S. J. Marrink, J. C. Hummelen and L. J. A. Koster, Adv. Mater., 2018, 30, 1804290 CrossRef.
- B. D. Naab, S. Guo, S. Olthof, E. G. Evans, P. Wei, G. L. Millhauser, A. Kahn, S. Barlow, S. R. Marder and Z. Bao, J. Am. Chem. Soc., 2013, 135, 15018–15025 CrossRef CAS.
- R. A. Schlitz, F. G. Brunetti, A. M. Glaudell, P. L. Miller, M. A. Brady, C. J. Takacs, C. J. Hawker and M. L. Chabinyc, Adv. Mater., 2014, 26, 2825–2830 CrossRef CAS.
Footnotes |
† Electronic supplementary information (ESI) available. See DOI: 10.1039/d0tc04094k |
‡ N. Y. Kim and T. S. Lee contributed equally to this work. |
|
This journal is © The Royal Society of Chemistry 2021 |