Blue-emitting butterfly-shaped donor–acceptor-type 1,3,5,9-tetraarylpyrenes: easily available, low-cost conventional fluorophores for high-performance near ultraviolet electroluminescence with CIEy < 0.05†
Received
30th July 2020
, Accepted 14th November 2020
First published on 16th November 2020
Abstract
Exploring the near ultraviolet (NUV) emitters with excellent electroluminescence performance is a crucial issue for high-density information storage, high-quality flat-panel displays and high-quality solid-state lighting. In this paper, three blue-emitting butterfly-shaped donor–acceptor (DA)-type pyrene-based emitters (3a–c) with a 1,3-A/5,9-D molecular structure have been successfully designed and synthesized by an easily available, low-cost method. All three compounds have conventional fluorescence characteristics with strong NUV emission both in solution and in thin film, and have good thermal stability, which meets the preparation basis of NUV organic light-emitting diodes (OLEDs). With the DA pyrenes (3a–c) as dopants, CBP-based OLED devices exhibit maximum EQEs of ∼3.0% with CIE coordinates with y < 0.05, which are remarkable compared to the reported NUV OLEDs with a similar color gamut. This is the first demonstration of using DA pyrenes as high efficiency NUV emitters in fluorescent OLEDs. Furthermore, employing 3c as a representative host, the DSA-Ph-doped sky-blue device displays a low turn-on voltage of 2.6 V and a maximum EQE, current efficiency (CE) and luminance of 6.84%, 12.95 cd A−1 and 13
954 cd m−2, respectively, which are comparable to the most advanced blue hosts such as MADN. This study not only demonstrates the potential of the present DA pyrenes in NUV OLEDs, but also provides tactics for the rational design of blue emitters by using the DA pyrene-based architecture.
Introduction
Near ultraviolet (NUV) emitters with excellent electroluminescence (EL) performance are of great importance for their unique applications in high-density information storage devices1 and lighting sources2–4 and as potential host materials for visible light emission.5–7 Nevertheless, due to their intrinsic wide band-gap, which leads to unbalanced charge-carrier injection and transportation in devices, high-performance organic light-emitting diodes (OLEDs) based on NUV emitters are not easy to achieve.4,8–11
It is well known that the construction of donor–acceptor (DA)-type fluorophores has been one of the effective strategies for the development of high-performance OLED materials.12–17 DA-type fluorophores not only have high photoluminescence quantum yields (PLQYs) resulting from the effective radiative decay of their excited intramolecular charge-transfer (ICT) states but also show impressive bipolar charge-transporting abilities as they possess electron- and hole-transporting moieties.7,18–21 Despite the above-mentioned challenge in NUV OLEDs, thanks to the efforts of scientists and progress in modern synthetic chemistry, various DA-type NUV emitters22–39 with fascinating EL performance (CIEy < 0.05) have been reported in recent years. These DA-type NUV emitters developed to date can be roughly classified into five types of material systems according to the different exciton radiative mechanisms (Table 2): (i) conventional fluorescence-type organic molecules,22–25 (ii) triplet–triplet annihilation (TTA)-type organic molecules,26–28 (iii) thermally activated delayed fluorescence (TADF)-type organic molecules,29–32 (iv) hybrid local and charge-transfer (HLCT)-type organic molecules,33–37 and (v) triplet–polaron interaction (TPI)-type organic molecules.38,39 To date, there are only a few efficient and stable DA-type NUV luminescent materials.26,35,38
Owing to its strong blue-violet fluorescence emission and charge-transporting properties, pyrene has been widely used as a building block for organic optoelectronic materials,40–42 especially in blue OLEDs.43–56 Among them, some of the non-DA-type pyrenes49–51 have been utilized as NUV OLED emitters (Fig. 1a). Examples of these are 2,7-bis(3,6-di-tert-butylcarbazol-9-yl)pyrene49 (4b), 2,7-bis(3-(3,6-di-tert-butyl-9H-carbazol-9-yl)phenyl)pyrene50 (5) and 1,6-bis(2,2′-(3,5-di-phenyl-phenyl)phenyl)pyrene51 (DPPP), which were reported by Kaafarani, Müllen and Xu, respectively. In NUV OLED applications (Table 2), for 4b, an EL emission peak at 435 nm was observed, resulting in CIEy = 0.044; however, a low device efficiency of 0.04 cd A−1 was achieved due to the unbalanced charge-carrier transportation in the active materials.49 Regarding 5, it can offer an exceptional deep EL colour purity (CIEy = 0.024) and a good EQE of 3.09%, but the device efficiencies are not available.50 The DPPP-based device shows a violet emission peak at 396 nm with CIEy = 0.041, and the maximum CE is only 0.74 cd A−1, corresponding to a maximum EQE of 2.2%.51 However, to date, there has been no report on NUV OLEDs based on DA-type pyrenes,52–56 which can provide desirable NUV emission with a CIEy value < 0.05.
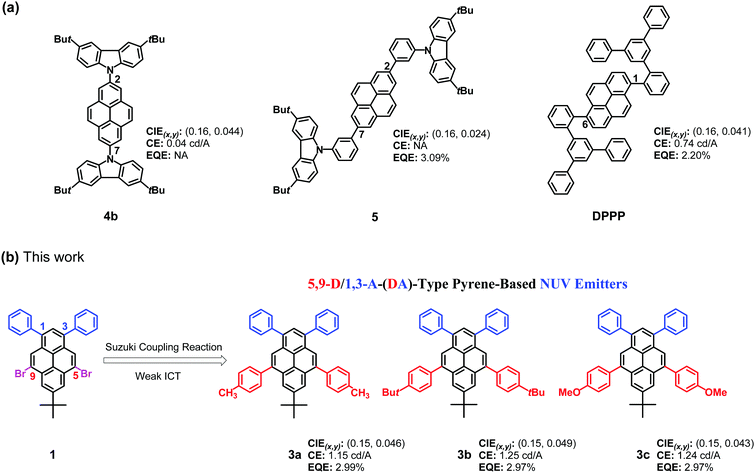 |
| Fig. 1 The chemical structures of (a) non-DA-type pyrene-based NUV emitters and (b) DA-type pyrene-based NUV emitters (3) in this work. | |
On the other hand, we recently developed a fascinating building block of 5,9-dibromo-7-tert-butyl-1,3-diphenylpyrene57 (1, Fig. 1b). Such a 5,9-dibrominated pyrene is an attractive archetypical structure for the development of functional π-conjugated materials for the following reasons: (i) the two phenyl groups at the active sites of the 1,3-positions could further enhance π-extension of the molecular structure, which is beneficial for high PLQYs,58 owing to the effective π-delocalization over the whole π-framework; (ii) modification at the K-region of the 5,9-positions enables incorporation into the backbone structures of π-conjugated small-molecules59 and polymers leading to various pyrenes, in which changes of π-conjugation patterns and rather rigid bulkiness are possible, providing variable photophysical properties with relatively weak ICT trends for generating short-wavelength emission, and effective steric hindrance to interchromophore packing. Accordingly, we have previously designed and synthesized two types of DA pyrenes (i.e., 1,3-A/5,9-D-type and 1,3-D/5,9-A-type) by covalently integrating two p-OMe/CHO-substituted phenyl units into 1 at the K-region of the 5,9-positions.57 Among them, we found that the 1,3-A/5,9-D-type pyrenes exhibit some intriguing properties, which are promising for NUV light-emitting devices. In this work, we synthesized three blue-emitting butterfly-shaped 1,3-A/5,9-D-type pyrenes (3a–c, Scheme 1) by Suzuki cross-coupling reaction. All three DA pyrenes form stable and homogeneous amorphous films by vacuum deposition, exhibiting high thin-film (5 wt% doped in CBP) PLQYs of 58–68% with NUV emission. These DA pyrenes are electrochemically stable as evaluated by both reversible oxidation and reduction. Furthermore, they exhibit unusual nondispersive bipolar carrier transporting properties with good transmitting capacity of holes and electrons. The 3a–c-doped CBP-based OLEDs exhibited NUV emission (CIEy < 0.05), and their maximum EQEs were infinitely close to 3.0%. Moreover, with 3c as a representative host, the DSA-Ph-doped sky-blue device achieves a turn-on voltage lower than 2.60 V, and the maximum EQE reaches 6.84% with a maximum luminance of 13
954 cd m−2. It is worth noting that the performance of the present DA pyrene-based devices is among the best for NUV OLEDs with CIEy < 0.0522–39,49–51 although they are conventional fluorophores. In particular, the highly efficient 3c-based DSA-Ph-doped device reveals the great prospect of DA pyrene-based hosts with respect to the most advanced blue hosts such as MADN.60
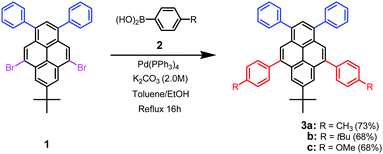 |
| Scheme 1 Synthetic route of the DA-type 1,3,5,9-tetraarylpyrenes (3a–c). | |
Results and discussion
Synthesis and characterization
Scheme 1 displays that the three DA pyrenes (3a–c) could be facilely synthesized in only one-step. The starting material of 5,9-dibromo-7-tert-butyl-1,3-diphenylpyrene (1), which was prepared from 7-tert-butyl-1,3-diphenylpyrene,58 was coupled with various p-donating-substituted phenylboronic acids (2) by Suzuki cross-coupling reaction. The crude compounds were purified by silica column chromatography, recrystallization and final sublimation. Their chemical structures were fully characterized by 1H/13C NMR spectroscopy, FT-IR spectroscopy, mass spectrometry and elemental analysis (see the ESI†). To evaluate the morphological stability of the DA pyrenes in the vacuum deposition process, differential scanning calorimetry (DSC) and thermal gravimetric analysis (TGA) were carried out. The results are displayed in Fig. S1 and S2 (ESI†). The blue DA pyrenes showed excellent thermal stability: no obvious glass transition temperature (Tg) was observed during DSC measurements. Additionally, TGA showed that the decomposition temperatures (Td, 5% weight loss) were all ≧345 °C. As a consequence, these DA pyrenes have a high Td and no Tg, which imply that they can maintain their amorphous morphology in a wide temperature range, which is ideal and outstanding for the vacuum deposition process and device fabrication.
Theoretical calculation
To validate the feasibility of this design for NUV emitters, density functional theory (DFT) calculations of the DA pyrenes 3 were performed by the Gaussian 16 program, using the B3LYP functional and the polarized 6-31G* basis set.61 In the optimized structures, all the DA pyrenes possessed large dihedral angles (∼54°–60°) between the four aryl groups and the center pyrene ring (Fig. 2). The large torsion angles could effectively inhibit the π–π accumulation of pyrene cores in the solid state. In the distributions of frontier molecular orbitals (FMOs), the HOMOs of the DA pyrenes are mainly located over the entire pyrene π-framework, while the 5,9-substituted aryl rings have limited contributions to this system, implying that the two aryl rings have an electron-donating nature.57 However, the LUMOs of the DA pyrenes are predominantly located both on the center pyrene core and the 1,3-substituted phenyl rings, indicating that the two phenyl rings have an electron-accepting nature.57 The HOMO/LUMO distributions of the DA pyrenes imply that small different ground-state and/or excited-state electronic structures exist in each molecule, originating from the electronic effect of the different p-donating-substituted phenyl rings at the 5,9-positions, thereby analogous photophysical properties are expected. The dipole moments of 3a–c were calculated as 0.74 for 3a, 0.75 for 3b, and 2.30 for 3c, respectively, deriving from their slightly different weak polar symmetrical structures. The UV-vis absorption spectra of S0 → S1 transitions of 3 were also computed to give similar theoretical evidence for their slightly different electronic structures (Fig. S3, ESI†). All the calculated values of the HOMOs/LUMOs are displayed in Fig. 2 and Table S1 in the ESI.†
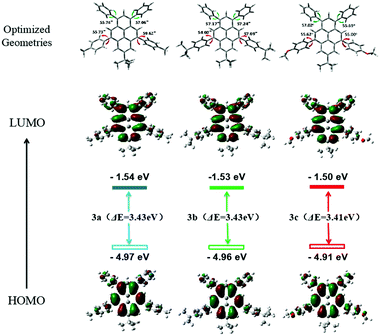 |
| Fig. 2 Molecular geometries, the HOMO and LUMO distributions and energy levels of 3a–c calculated using DFT calculations. | |
Single-crystal X-ray analysis
We further confirmed the chemical structure of 3b by single-crystal X-ray analysis.62 The crystals were grown by slow evaporation of a CH2Cl2/CHCl3 solution. The crystallographic data of 3b are displayed in Table S2 (ESI†). As shown in Fig. 3a, the dihedral angles of 3b between the four aryl substituents and the pyrene ring are ca. 55°–69°. The two dihedral angles of 3b between the two 5,9-substituted aryl rings and the center pyrene core show more distortion (67.6° and 68.1°) with respect to the single-crystal of 3c57 (61.8° and 67.1°), possibly deriving from the more bulky tert-butyl moiety compared to the OMe group. The results are highly consistent with the DFT computed data. Additionally, abundant intermolecular interactions, such as C–H⋯interactions, were observed in the single-crystal of 3b (Fig. 3c), which may possibly rigidify its molecular conformation and restrain the molecular motion against the effect of aggregation-caused quenching (ACQ),63 leading to bright emission in the solid state. Moreover, the packing structures of 3b can be depicted as a regular packing form of J-aggregations64 (Fig. S5, ESI†). As shown in Fig. 3d, large packing distances between neighboring molecules in the single-crystals of 3b were observed (ca. 9.256 and 14.166 Å), demonstrating that π–π stacking and excimer formation were completely suppressed in the solid state, which is favorable for obtaining high PLQYs.59
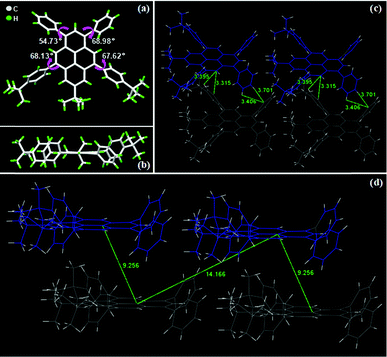 |
| Fig. 3 Single-crystal structures of 3b: (a) top view (up), (b) side view (bottom), (c) intermolecular interactions of C–H⋯π (green dashed lines) and (d) intermolecular stacking distances. | |
Photophysical properties
Fig. 4 shows the optical properties of the DA pyrenes 3a–c recorded in CH2Cl2 (∼10−5 M) and as neat thin-films, and the corresponding data are summarized in Table 1. As shown in Fig. 4a, all the DA pyrenes reveal broad S0 → 1CT absorption bands at 350–400 nm, which could be attributed to the ICT processes.65 Among them, for 3a and 3b, almost the same broad and featured fine structure characteristics of their spectra were observed, originating from their weak polar structure, which resulted in weak ICT processes; however, for 3c, a broader and less well-resolved spectrum was obtained, which can be attributed to its stronger ICT processes, which originated from its relatively stronger polar structure. This tiny difference of S0 → 1CT absorption bands between 3a/3b and 3c mainly originated from their slightly different electronic structures. The results are also well consistent with the DFT calculations on both their FMOs and UV-vis absorption of S0 → S1 transitions (Fig. S3, ESI†). The emission maxima of 3 were recorded at 415 nm for 3a, 415 nm for 3b, and 419 nm for 3c in the NUV region, respectively. Accordingly, the 1CT energies of 3 calculated from the onset of their prompt emission spectra are 3.07 eV for 3a, 3.07 eV for 3b, and 3.05 eV for 3c, respectively. As shown in Fig. 4b, for the DA pyrenes, their thin film emission spectra exhibited a prominent maximum emission in the blue-violet region at 428 nm for 3a, 434 nm for 3b, and 432 nm for 3c, respectively. Interestingly, the emission of 3b is slightly red-shifted by ∼2 nm relative to the spectrum of 3c despite the weaker electron-donating nature of the tBu group than the OMe group, which can probably be attributed to its packing structures with J-aggregations64 and the different dielectric constants.66 The result was also consistent with the single-crystal analysis.
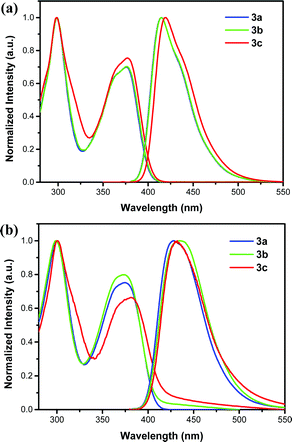 |
| Fig. 4 UV-vis absorption spectra and emission spectra of the DA pyrenes 3: (a) CH2Cl2 solutions (∼10−5 M) and (b) thin films. | |
Table 1 Photophysical properties of compounds 3a–c
Compd |
λ
abs,max
(nm) |
λ
em,max (nm) |
Φ
f
|
E
g
(eV) |
E
onsetox d (V) |
HOMO/LUMOe (eV) |
S1/T1f (eV) |
S1/T1g (eV) |
τ (ns) |
K
r/Knr (×108) |
T
m/Td (°C) |
CH2Cl2 (ε × 105) |
Film |
CH2Cl2 |
Film |
CH2Cl2/film/doped filmb |
Molar absorption coefficient (ε): M−1 cm−1.
The compounds 3a, 3b and 3c, 5 wt%-doped in CBP thin films.
Optical band-gap estimated from the onset point (onset) of the absorption band: Eopt = 1240/λonset.
E
onsetox is the onset oxidation potential, potential vs. calomel electrode, working electrode: glassy carbon, and 0.1 M Bu4NPF6–CH2Cl2.
The HOMO and LUMO energy levels were calculated according to the equations: HOMO = −(4.8 eV + E1/2ox) and LUMO = HOMO + Eg.
S1/T1 values were obtained by DT-DFT calculations.
S1/T1 values were obtained from the fluorescence (at room temperature) and phosphorescence (at 77 K) spectra in toluene.
|
3a
|
376 |
375 |
415 |
428 |
0.67/0.18/0.68 |
3.07 |
1.04 |
−5.84/−2.77 |
3.33/1.95 |
3.16/2.34 |
3.72 |
1.8/0.89 |
316/345 |
3b
|
376 |
374 |
415 |
434 |
0.68/0.18/0.58 |
3.07 |
1.00 |
−5.80/−2.73 |
3.33/1.95 |
3.16/2.34 |
3.09 |
2.2/1.0 |
363/385 |
3c
|
377 |
375 |
419 |
432 |
0.74/0.36/0.60 |
3.05 |
0.92 |
−5.72/−2.67 |
3.19/1.94 |
3.13/2.33 |
2.73 |
2.7/0.95 |
312/365 |
As shown in Fig. S7–S9 (ESI†), the spectral properties of solvatochromism for 3 were measured in different organic solvents. All three compounds showed slightly solvato-chromic absorption (∼3 nm) and emission (∼5 nm) due to their weak ICT processes (Table S3, ESI†). The relationship between the Stokes-shifts in various solvents and the Lippert equation67,68 shows a linear correlation between these two factors (Fig. S11, ESI†). The PLQYs of 3 recorded in dilute CH2Cl2 and as thin films are 67% and 18% for 3a, 68% and 18% for 3b, and 74% and 36% for 3c, respectively. In contrast, the 5 wt%-doped CBP films of 3 exhibited higher quantum yields of 68%, 58% and 60%, respectively. The results suggest that these DA pyrenes may be potential candidates for the fabrication of NUV host–guest-based OLEDs.69
Electrochemical properties
To investigate the electrochemical properties of the DA pyrenes 3a–c, the oxidation and reduction potentials were measured in CH2Cl2 solutions and N,N-dimethylformamide (DMF) solutions, respectively. As shown in Fig. 5, the oxidation potentials are around +1.04 ± 0.05 V for 3a, +1.00 ± 0.05 V for 3b, and +0.92 ± 0.05 V for 3c, respectively, and the reduction potentials are around −0.86 ± 0.05 V for 3a, −0.86 ± 0.05 V for 3b, and −1.08 ± 0.05 V for 3c, respectively. The oxidation/reduction potential of 3a is slightly higher than those of 3b and 3c, which is possibly due to the weakest electron donating nature of the Me group with respect to the tBu group and the OMe group.70 The optical bandgap (Eg) was calculated to be 3.07 eV for 3a, 3.07 eV for 3b, and 3.05 eV for 3c from their UV-vis absorption onsets. Accordingly, the HOMOs/LUMOs of 3 were estimated from the CVs and the Egs, and all data are summarized in Table 1. The HOMOs of 3a (−5.84 eV), 3b (−5.80 eV) and 3c (−5.72 eV) are comparable to that of 1,1-bis(di-4-tolyl-aminophenyl)cyclohexane (TAPC)46 (−5.60 eV) and the LUMOs of 3a (−2.77 eV), 3b (−2.73 eV) and 3c (−2.67 eV) are close to that of 3,5,3′′,5′′-tetra-3-pyridyl-1,1′;3,1′′-terphenyl (B3PyPB)71 (−2.7 eV), which are beneficial for good hole/electron-injection in the fabrication of OLEDs.72
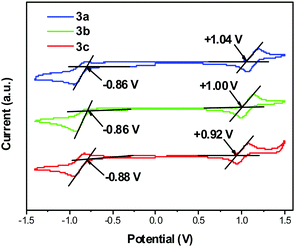 |
| Fig. 5 Cyclic voltammograms of compounds 3a–c in 0.1 M Bu4NPF6–CH2Cl2 (oxidation)/N,N-dimethylformamide (DMF) (reduction). | |
Electroluminescence
The DA pyrenes were employed for OLED applications and devices A, B, and C were fabricated by using 3a, 3b, and 3c as dopants, respectively. The device structure for A–C was indium tin oxide (ITO)/dipyrazino[2,3-f:20,30-h]quinoxaline-2,3,6,7,10,11-hexacarbonitrile (HAT-CN) (5 nm)/TAPC (40 nm)/4,4′-bis(N-carbazolyl)-1,1′-biphenyl (CBP): dopant (3a or 3b or 3c) (5 wt%) (20 nm)/B3PyPB (40 nm)/LiF (1 nm)/Al (100 nm). Among the above-mentioned materials, HAT-CN served as the hole injection layer (HIL) and TAPC acted as the hole transporting layer (HTL). CBP was the host material used to disperse the DA pyrene emitting dopants. B3PyPB was selected as an electron transporting layer (ETL) and hole blocker (HBL). Lithium fluoride (LiF) was used as an electron injection layer (EIL) and aluminum (Al) was used as a metal cathode. All molecular structures are displayed in the ESI† (Fig. S10). The EL properties including EL spectra, the current density and luminance versus voltage, and the EQE versus current density, and the energy level diagrams of the materials, are shown in Fig. 6, and all data are displayed in Table 2. The maximum EL emission spectra of devices A–C are between 422 and 423 nm, and they show no emission from the CBP host or obvious trailing at long wavelengths, indicating complete energy transfer and suppression of excimer or exciplex formation in the EL process (Fig. 6a). For all three devices, the CIE coordinate of y is less than 0.05, meeting the standard of NUV emission (Fig. 6a), the turn-on voltage is between 3.2 and 3.6 V, illustrating a good charge-carrier injection and transport (Fig. 6b), and the maximum EQE is infinitely close to 3.0% (Fig. 6c) (Table 2). When the current density is increased from 0.1 to 10 mA cm−2, the EL spectrum remains stable, which proves that the device has good color stability (Fig. S12, ESI†). It is worth noting that the full width at half-maximum (FWHM) of the EL spectra of all the devices is less than 60 nm. In the 3b-based device C, CE, PE, and EQE values of 1.25 cd A−1, 1.31 lm W−1 and 2.97% were achieved, respectively, which are remarkable among the reported NUV OLEDs22–39,49–51 with a similar color gamut. The high-performance of the 3-doped CBP-based NUV devices could be attributed to the following possible factors: (i) the choice of an appropriate host and optimization of the dopant concentration are important procedures for achieving high device performance. As shown in Fig. S12 (ESI†), the overlap between the PL spectrum of CBP and the absorption spectrum of 3 was remarkable, enabling efficient host-to-guest Förster energy transfer, and hence, highly improved efficiency. (ii) The use of the DA type light-emitting dopant is a momentous factor for obtaining high device performance. As shown in Fig. 6d, the HOMO of the DA pyrene-based dopant is shallower than that of the CBP host, while the LUMO is deeper, which may contribute to the direct trapping of holes and electrons on the dopant, leading to a good charge-carrier balance and high efficiency. (iii) The high PLQY of the doped film is an important factor in increasing the EQE value of the device to close to 3.0%. In this study, the PLQY of 5 wt% 3 in the CBP films was measured to be 68% for 3a, 58% for 3b, and 60% for 3c, respectively. Accordingly, the theoretical upper limit for EQE would be in the ranges of 3.4–5.1% for 3a, 2.9–4.4% for 3b and 3.0–4.5% for 3c, respectively, depending on the outcoupling efficiency. Consequently, the measured EQE value was below the theoretically obtained values, which could originate from the conventional fluorescence characteristics of 3.
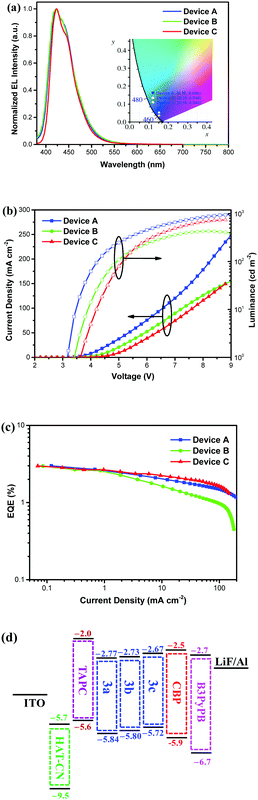 |
| Fig. 6 (a) EL spectra. Inset shows the 1931 CIE chromaticity of devices A, B, and C. (b) Current density and luminance vs. voltage characteristics of devices A, B, and C. (c) EQEs vs. current density of devices A, B, and C. (d) Energy level diagrams for electrochemical properties of devices A, B, and C. | |
Table 2 Summary of key characteristics of 3a–c-based OLED devices and other high performance NUV light-emitting devices with CIEy < 0.05
Emitters |
FWHMa (nm) |
V
on
(V) |
CEc (cd A−1) |
PEd (lm W−1) |
EQEe (%) |
λ
EL
(nm) |
CIEg (x, y) |
Ref. |
Full width at half-maximum (FWHM).
Turn-on voltage at 1 cd m−2.
Maximum current efficiency.
Maximum power efficiency.
Maximum external quantum efficiency.
The maximum electroluminescence wavelength.
Commission Internationale de l'Eclairage (CIE) coordinates at 5 V.
Doped devices.
Non-doped devices. (A: device A; B: device B; C: device C.)
|
3a (A) |
54 |
3.2 |
1.15 |
1.13 |
2.99 |
422 |
(0.15, 0.046) |
This workh |
3b (B) |
58 |
3.4 |
1.25 |
1.31 |
2.97 |
422 |
(0.15, 0.049) |
This workh |
3c (C) |
59 |
3.6 |
1.24 |
1.09 |
2.97 |
423 |
(0.15, 0.043) |
This workh |
PPI-2BI
|
— |
3.6 |
1.78 |
1.45 |
4.12 |
432 |
(0.15, 0.047) |
22
|
TPI-Bz
|
— |
3.2 |
0.50 |
0.45 |
1.45 |
420 |
(0.16, 0.043) |
23
|
PIMNA
|
— |
3.8 |
0.51 |
— |
2.43 |
412 |
(0.16, 0.035) |
24
|
SiBPI
|
— |
3.8 |
1.04 |
— |
2.62 |
424 |
(0.17, 0.046) |
25
|
C3FLA-2
|
— |
— |
2.20 |
1.20 |
8.00 |
416 |
(0.16, 0.048) |
26
|
TPAXAM
|
49 |
3.4 |
— |
— |
4.62 |
428 |
(0.15, 0.049) |
27
|
TDAF1
|
49 |
4.0 |
1.53 |
— |
5.30 |
— |
(0.16, 0.041) |
28
|
TDAF2
|
44 |
4.0 |
1.10 |
— |
4.10 |
— |
(0.16, 0.044) |
28
|
SDPS-4PhCz
|
— |
3.0 |
1.15 |
1.12 |
4.92 |
417 |
(0.14, 0.040) |
29
|
pBTCz-26DPPM
|
— |
4.5 |
2.35 |
1.68 |
5.13 |
— |
(0.16, 0.048) |
30
|
ICzCz
|
49 |
— |
0.70 |
0.50 |
2.30 |
416 |
(0.17, 0.040) |
31
|
CZs2
|
— |
2.8 |
0.82 |
0.84 |
2.70 |
417 |
(0.16, 0.044) |
32
|
DFPBI
|
61 |
3.0 |
1.48 |
— |
4.18 |
435 |
(0.15, 0.042) |
33
|
DFPBI
|
56 |
3.0 |
1.62 |
— |
4.96 |
425 |
(0.16, 0.039) |
33
|
Tmtp
|
— |
3.3 |
1.24 |
1.14 |
3.00 |
436 |
(0.15, 0.040) |
34
|
TPIBCz
|
— |
3.2 |
2.10 |
2.20 |
5.47 |
432 |
(0.16, 0.047) |
35
|
TPIBNCz
|
— |
3.2 |
2.34 |
2.14 |
5.99 |
428 |
(0.16, 0.048) |
35
|
mTPA-PPI
|
47 |
3.2 |
0.84 |
0.48 |
3.33 |
404 |
(0.16, 0.049) |
36
|
TPA-PIM
|
35 |
— |
1.14 |
0.79 |
3.28 |
420 |
(0.16, 0.046) |
37
|
TPATZ
|
50 |
3.1 |
2.41 |
2.20 |
5.92 |
430 |
(0.16, 0.047) |
38
|
TPA-TAZ
|
— |
— |
— |
— |
6.80 |
— |
(0.16, 0.043) |
39
|
4b
|
— |
4.2 |
0.04 |
— |
— |
435 |
(0.16, 0.044) |
49
|
5
|
— |
— |
— |
— |
3.09 |
412 |
(0.16, 0.024) |
50
|
DPPP
|
50 |
4.0 |
0.74 |
— |
2.20 |
396 |
(0.16, 0.041) |
51
|
Single-carrier devices
To gain a better understanding of the different EL performance and the charge-carrier injection and transport ability, the hole-only and the electron-only devices of the DA pyrenes 3a–c were fabricated. The configuration structure of the hole-only device is ITO/TAPC (10 nm)/3a or 3b or 3c (70 nm)/TAPC (10 nm)/Al (100 nm), and that of the electron-only device is ITO/B3PyPB (10 nm)/3a or 3b or 3c (70 nm)/B3PyPB (10 nm)/LiF (1 nm)/Al (100 nm). For the hole-only devices, TAPC (2.0 eV) was used to block electrons near the Al cathode (4.3 eV, corresponding to a large energy barrier of 2.3 eV) to ensure the pure hole characteristics of the device. Similarly, in the electron-only devices near the ITO anode (4.8 eV), B3PyPB (6.7 eV, corresponding to a large energy barrier of 1.9 eV) was used to prevent hole injection. As shown in Fig. 7, for all three single-carrier devices, significant currents were observed, while being less balanced, demonstrating the bipolar charge-transporting abilities of the DA pyrenes 3. Additionally, compared to those of the 3b and 3c devices, the hole and electron currents of the 3a devices are both much higher. These results could explain why the 3a-based device achieved a relatively lower turn-on voltage and higher current density/luminescence, while the 3b/3c-based device exhibited higher EL efficiencies. Thus, the single-carrier characteristic is an important factor for improved device performance.73
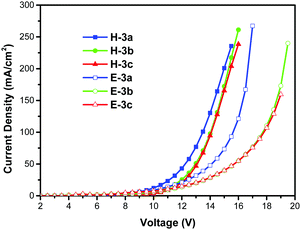 |
| Fig. 7 Current density–voltage curves of 3a-/3b-/3c-based hole-only and electron-only devices. | |
To further explore the ability of the DA pyrenes to act as hosts in sky-blue OLEDs, a doped device D was fabricated by using 3c as a representative host and DSA-Ph as a dopant with the following configuration: ITO/HAT-CN (5 nm)/(TAPC) (40 nm)/3c: DSA-Ph (5 wt%) (20 nm)/B3PyPB (40 nm)/LiF (1 nm)/Al (100 nm). All molecular structures and energy level diagrams of device D are shown in Fig. S12 and S20, ESI,† respectively. The EQE–luminance characteristics, current density–voltage–luminance and CE–luminance–PE for the sky-blue device are shown in Fig. 8, and key device performance data are summarized in Table 3. From the normalized EL spectra of device D in Fig. 8a (inset), a main luminescence peak located at 468 nm and two shoulder peaks at 492 nm and 536 nm can be seen, which are typical characteristic luminescence peaks of the guest material DSA-Ph.60 The result indicates that there is an efficient Förster energy transfer in this doping system. The device shows a low turn-on voltage value (Von, voltage at 1.0 cd m2) of 2.6 V, which reveals a low charge-carrier injection barrier from both TAPC and B3PyPB to 3c. In particular, device D exhibits a maximum CE of 12.95 cd A−1, a maximum PE of 13.56 lm W−1 and a maximum EQE of 6.84%. The high performance of the 3c-hosted sky-blue device with the DSA-Ph dopant may also be attributed to the previously described possible factors for devices A–C. Accordingly, the capability of 3 as a blue host material can be compared with that of both the pyrene-based blue hosts74 (i.e.TPP, TMTP and TOTP) and the anthracene-derived blue hosts60,74,75 (i.e.ADN, TBADN, MADN and MBA) (Table 3).
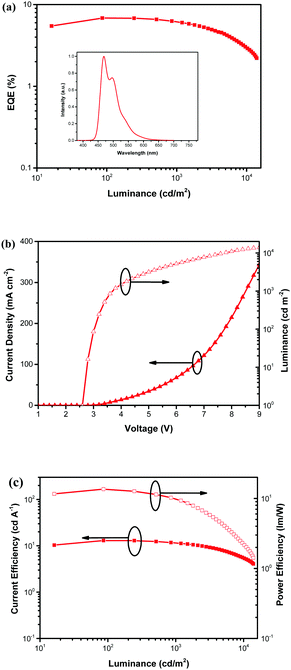 |
| Fig. 8 (a) EQE vs. luminance curve of device D, inset: normalized EL spectra. (b) Current density–voltage–luminance characteristics of device D. (c) CE vs. luminance and PE vs. luminance curves of device D. | |
Table 3 Key performance parameters of the EL performance of device D, and the representative DSA-Ph-doped TOTP, ADN, TBAADN, MADN and MBA-based sky-blue OLEDs
Dopant |
V
on
(V) |
CEb (cd A−1) |
PEc (lm W−1) |
EQEd (%) |
L
(cd m−2) |
λ
EL
(nm) |
CIEg (x, y) |
Ref. |
Turn-on voltage at 1 cd m−2.
Maximum current efficiency.
Maximum power efficiency.
Maximum external quantum efficiency.
Maximum luminance.
EL emission peak of the EL spectrum at 8 V.
Commission Internationale de l'Eclairage (CIE) coordinates at 8 V.
All EL data were collected at 20 mA cm−2. (D: device D.)
|
3c (D) |
2.6 |
12.95 |
13.56 |
6.84 |
13 954 |
468 |
(0.15, 0.28) |
This work |
TOTP
|
7.1 |
8.64 |
3.82 |
4.32 |
— |
— |
(0.15, 0.28) |
74
|
ADN
|
6.6 |
8.08 |
3.82 |
3.94 |
— |
— |
(0.15, 0.35) |
74
|
TBADN
|
7.1 |
9.08 |
4.01 |
4.56 |
— |
— |
(0.15, 0.33) |
74
|
MADN
|
4.0 |
9.70 |
5.50 |
— |
— |
464 |
(0.16, 0.32) |
60
|
MBA
|
3.0 |
16.54 |
16.57 |
9.47 |
13 800 |
468 |
(0.15, 0.26) |
75
|
Conclusions
In summary, three pyrene-based highly efficient NUV fluorophores with the 1,3-A/5,9-D molecular feature, 3a, 3b and 3c, have been developed via a DA structure design strategy, which incorporates benzene as a weak electron-acceptor and a p-donating-substituted phenyl unit as a mild electron-donor. The DA pyrene-doped devices with the CBP host exhibit NUV light emission with CIE coordinates of (0.15, 0.046) for 3a, (0.15, 0.049) for 3b and (0.15, 0.043) for 3c at 5 V, respectively. Among them, the 3b-based device shows higher performance with EQE, CE, and PE values of 2.97%, 1.25 cd A−1, and 1.31 lm W−1, respectively, which are among the best for NUV OLEDs to date and is the first demonstration of using DA pyrenes as high efficiency NUV emitters in conventional fluorescent OLEDs. Moreover, using 3c as a representative host, the DSA-Ph-doped sky-blue device shows a low turn-on voltage of 2.6 V and a maximum EQE, CE and luminance of 6.84%, 12.95 cd A−1 and 13
954 cd m−2, respectively, which are comparable to those of the most advanced blue hosts. Our results not only demonstrate the potential of the present DA pyrenes in NUV OLEDs with high efficiency and favorable colour-purity, but also provide tactics for the rational design of blue emitters by using the DA type molecular feature.
Conflicts of interest
No competing financial interests have been declared.
Acknowledgements
This work was financially supported by the National Key Research & Development Program (2016YFB0401005), the National Natural Science Foundation of China (61874170), and the Program for Science & Technology Innovation Team of Shaanxi Province (2018TD-030).
References
- H. V. Santen and J. H. M. Neijzen, Jpn. J. Appl. Phys., 2003, 42, 1110–1112 CrossRef.
- D. F. O'Brien, M. A. Baldo, M. E. Thompson and S. R. Forrest, Appl. Phys. Lett., 1999, 74, 442–444 CrossRef.
- S.-J. Yeh, M.-F. Wu, C.-T. Chen, Y.-H. Song, Y. Chi, M.-H. Ho, S.-F. Hsu and C.-H. Chen, Adv. Mater., 2005, 17, 285–289 CrossRef CAS.
- T.-C. Chao, Y.-T. Lin, C.-Y. Yang, T. S. Hung, H.-C. Chou, C.-C. Wu and K.-T. Wong, Adv. Mater., 2005, 17, 992–996 CrossRef CAS.
- C. W. Tang and S. A. VanSlyke, Appl. Phys. Lett., 1987, 51, 913–915 CrossRef CAS.
- M. A. Baldo, D. F. O'Brien, Y. You, A. Shoustikov, S. Sibley, M. E. Thompson and S. R. Forrest, Nature, 1998, 395, 151–154 CrossRef CAS.
- S. J. Lee, J. S. Park, K. J. Yoon, Y. I. Kim, S. H. Jin, S. K. Kang, Y. S. Gal, S. Kang, J. Y. Lee, J. W. Kang, S. H. Lee, H. D. Park and J. J. Kim, Adv. Funct. Mater., 2008, 18, 3922–3930 CrossRef CAS.
- Y. Y. Lyu, J. H. Kwak, O. Kwon, S. H. Lee, D. Kim, C. H. Lee and K. Char, Adv. Mater., 2008, 20, 2720–2729 CrossRef CAS.
- A. L. Fisher, K. E. Linton, K. T. Kamtekar, C. Pearson, M. R. Bryce and M. C. Petty, Chem. Mater., 2011, 23, 1640–1642 CrossRef CAS.
- S. Tang, M. Liu, P. Lu, H. Xia, M. Li, Z. Q. Xie, T. Z. Shen, C. Gu, H. P. Wang, B. Yang and Y. G. Ma, Adv. Funct. Mater., 2007, 17, 2869–2877 CrossRef CAS.
- X. Tang, L. Yao, H. Liu, F. Shen, S. Zhang, Y. Zhang, H. Zhang, P. Lu and Y. Ma, J. Mater. Chem. C, 2014, 2, 5019–5027 RSC.
- T.-H. Huang, J. T. Lin, L.-Y. Chen, Y.-T. Lin and C.-C. Wu, Adv. Mater., 2006, 18, 602–606 CrossRef CAS.
- M.-Y. Lai, C.-H. Chen, W.-S. Huang, J. T. Lin, T.-H. Ke, L.-Y. Chen, M.-H. Tsai and C.-C. Wu, Angew. Chem., Int. Ed., 2008, 47, 581–585 CrossRef CAS.
- J. Ye, Z. Chen, M.-K. Fung, C. Zheng, X. Ou, X. Zhang, Y. Yuan and C.-S. Lee, Chem. Mater., 2013, 25, 2630–2637 CrossRef CAS.
- W. Li, Y. Pan, R. Xiao, Q. Peng, S. Zhang, D. Ma, F. Li, F. Shen, Y. Wang, B. Yang and Y. Ma, Adv. Funct. Mater., 2014, 24, 1609–1614 CrossRef CAS.
- X. Qiu, S. Xue, Y. Wu, M. Chen, Q. Sun and W. Yang, J. Mater. Chem. C, 2016, 4, 5988–5995 RSC.
- I. S. Park, S. Y. Lee, C. Adachi and T. Yasuda, Adv. Funct. Mater., 2016, 26, 1813–1821 CrossRef CAS.
- S.-L. Lin, L.-H. Chan, R.-H. Lee, M.-Y. Yen, W.-J. Kuo, C.-T. Chen and R.-J. Jeng, Adv. Mater., 2008, 20, 3947–3952 CrossRef CAS.
- C.-H. Chen, W.-S. Huang, M.-Y. Lai, W.-C. Tsao, J. T. Lin, Y.-H. Wu, T.-H. Ke, L.-Y. Chen and C.-C. Wu, Adv. Funct. Mater., 2009, 19, 2661–2670 CrossRef CAS.
- Q. S. Zhang, J. Li, K. Shizu, S. P. Huang, S. Hirata, H. Miyazaki and C. Adachi, J. Am. Chem. Soc., 2012, 134, 14706–14709 CrossRef CAS.
- J.-Y. Hu, Y.-J. Pu, F. Satoh, S. Kawata, H. Katagiri, H. Sasabe and J. Kido, Adv. Funct. Mater., 2014, 24, 2064–2071 CrossRef CAS.
- B. Liu, Z.-L. Zhu, J.-W. Zhao, D. He, Z.-Y. Wang, C.-Y. luo, Q.-X. Tong, C.-S. Lee and S.-L. Tao, Chem. – Eur. J., 2018, 24, 15566–15571 CrossRef CAS.
- W.-C. Chen, Y. Yuan, Y. Xiong, A. L. Rogach, Q.-X. Tong and C.-S. Lee, ACS Appl. Mater. Interfaces, 2017, 9, 26268–26278 CrossRef CAS.
- T. Shan, Y. Liu, X. Tang, Q. Bai, Y. Gao, Z. Gao, J. Li, J. Deng, B. Yang, P. Lu and Y. Ma, ACS Appl. Mater. Interfaces, 2016, 8, 28771–28779 CrossRef CAS.
- Z. Gao, F. Liu, J. Li, G. Cheng and P. Lu, RSC Adv., 2016, 6, 81744–81749 RSC.
- J.-H. Jou, S. Kumar, P.-H. Fang, A. Venkateswararao, K. R. J. Thomas, J.-J. Shyue, Y.-C. Wang, T.-H. Li and H.-H. Yu, J. Mater. Chem. C, 2015, 3, 2182–2194 RSC.
- R. Kim, S. Lee, K.-H. Kim, Y.-J. Lee, S.-K. Kwon, J.-J. Kim and Y.-H. Kim, Chem. Commun., 2013, 49, 4664–4666 RSC.
- C.-C. Wu, Y.-T. Lin, K.-T. Wong, R.-T. Chen and Y.-Y. Chien, Adv. Mater., 2004, 16, 61–65 CrossRef CAS.
- R. Wang, Y. Liu, T. Hu, X. Wei, J. Liu, Z. Li, X. Hu, Y. Yi, P. Wang and Y. Wang, Org. Electron., 2019, 71, 24–30 CrossRef CAS.
- Q. Zhang, S. Xiang, Z. Huang, S. Sun, S. Ye, X. Lv, W. Liu, R. Guo and L. Wang, Dyes Pigm., 2018, 155, 51–58 CrossRef CAS.
- J.-A. Seo, Y. Im, S. H. Han, C. W. Lee and J. Y. Lee, ACS Appl. Mater. Interfaces, 2017, 9, 37864–37872 CrossRef CAS.
- J. Ye, Z. Chen, M.-K. Fung, C. Zheng, X. Ou, X. Zhang, Y. Yuan and C.-S. Lee, Chem. Mater., 2013, 25, 2630–2637 CrossRef CAS.
- X. Qiu, S. Ying, C. Wang, M. Hanif, Y. Xu, Y. Li, R. Zhao, D. Hu, D. Ma and Y. Ma, J. Mater. Chem. C, 2019, 7, 592–600 RSC.
- G. Li, J. Zhao, D. Zhang, J. Zhu, Z. Shi, S. Tao, F. Lu and Q. Tong, New J. Chem., 2017, 41, 5191–5197 RSC.
- W.-C. Chen, Y. Yuan, S.-F. Ni, Q.-X. Tong, F.-L. Wong and C.-S. Lee, Chem. Sci., 2017, 8, 3599–3608 RSC.
- H. Liu, Q. Bai, L. Yao, H. Zhang, H. Xu, S. Zhang, W. Li, Y. Gao, J. Li, P. Lu, H. Wang, B. Yang and Y. Ma, Chem. Sci., 2015, 6, 3797–3804 RSC.
- W. Li, L. Yao, H. Liu, Z. Wang, S. Zhang, R. Xiao, H. Zhang, P. Lu, B. Yang and Y. Ma, J. Mater. Chem. C, 2014, 2, 4733–4736 RSC.
- J. Shi, Q. Ding, L. Xu, X. Lv, Z. Liu, Q. Sun, Y. Pan, S. Xue and W. Yang, J. Mater. Chem. C, 2018, 6, 11063–11070 RSC.
- A. Obolda, Q. Peng, C. He, T. Zhang, J. Ren, H. Ma, Z. Shuai and F. Li, Adv. Mater., 2016, 28, 4740–4746 CrossRef CAS.
- Y. Qiao, J. Zhang, W. Xu and D. Zhu, Tetrahedron, 2011, 67, 3395–3405 CrossRef CAS.
- F. Tang, K. Wu, Z. Zhou, G. Wang, B. Zhao and S. Tan, ACS Appl. Energy Mater., 2019, 2, 3918–3926 CrossRef CAS.
- T. M. Figueira-Duarte and K. Müllen, Chem. Rev., 2011, 111, 7260–7314 CrossRef CAS.
- J. N. Moorthy, P. Natarajan, P. Venkatakrishnan, D.-F. Huang and T. J. Chow, Org. Lett., 2007, 9, 5215–5218 CrossRef CAS.
- M. Y. Lo, C. Zhen, M. Lauters, G. E. Jabbour and A. Sellinger, J. Am. Chem. Soc., 2007, 129, 5808–5809 CrossRef CAS.
- K.-C. Wu, P.-J. Ku, C.-S. Lin, H.-T. Shih, F.-I. Wu, M.-J. Huang, J.-J. Lin, I.-C. Chen and C.-H. Cheng, Adv. Funct. Mater., 2008, 18, 67–75 CrossRef CAS.
- J.-Y. Hu, Y.-J. Pu, G. Nakata, S. Kawata, H. Sasabe and J. Kido, Chem. Commun., 2012, 48, 8434–8436 RSC.
- T. Suzuki, Y. Nonaka, T. Watabe, H. Nakashima, S. Seo, S. Shitagaki and S. Yamazaki, Jpn. J. Appl. Phys., 2014, 53, 052102 CrossRef.
- H. Jung, S. Kang, H. Lee, Y.-J. Yu, J. H. Jeong, J. Song, Y. Jeon and J. Park, ACS Appl. Mater. Interfaces, 2018, 10, 30022–30028 CrossRef CAS.
- B. R. Kaafarani, A. O. El-Ballouli, R. Trattnig, A. Fonari, S. Sax, B. Wex, C. Risko, R. S. Khnayzer, S. Barlow, D. Patra, T. V. Timofeeva, E. J. W. List, J.-L. Brédas and S. R. Marder, J. Mater. Chem. C, 2013, 1, 1638–1650 RSC.
- D. Chercka, S.-J. Yoo, M. Baumgarten, J.-J. Kim and K. Müllen, J. Mater. Chem. C, 2014, 2, 9083–9086 RSC.
- Z.-Q. Wang, C.-L. Liu, C.-J. Zheng, W.-Z. Wang, C. Xu, M. Zhu, B.-M. Ji, F. Li and X.-H. Zhang, Org. Electron., 2015, 23, 179–185 CrossRef CAS.
- G. Mallesham, C. Swetha, S. Niveditha, M. E. Mohanty, N. J. Babu, A. Kumar, K. Bhanuprakash and V. J. Rao, J. Mater. Chem. C, 2015, 3, 1208–1224 RSC.
- Y.-F. Li, X. Xie, X.-j. Gong, M.-L. Liu, R.-F. Chen, D.-Q. Gao and W. Huang, Dyes Pigm., 2017, 145, 43–53 CrossRef CAS.
- Z. Wang, C. Zheng, W. Fu, C. Xu, J. Wu and B. Ji, New J. Chem., 2017, 41, 14152–14160 RSC.
- C. Fu, S. Luo, Z. Li, X. Ai, Z. Pang, C. Li, K. Chen, L. Zhou, F. Li, Y. Huang and Z. Lu, Chem. Commun., 2019, 55, 6317–6320 RSC.
- W. Jiang, Y. Shen, Y. Ge, C. Zhou, Y. Wen, H. Liu, H. Liu, S. Zhang, P. Lu and B. Yang, J. Mater. Chem. C, 2020, 8, 3367–3373 RSC.
- R. Liu, H. Ran, Z. Zhao, X. Yang, J. Zhang, L. Chen, H. Sun and J.-Y. Hu, ACS Omega, 2018, 3, 5866–5875 CrossRef CAS.
- X. Feng, J.-Y. Hu, L. Yi, N. Seto, Z. Tao, C. Redshaw, M. R. J. Elsegood and T. Yamato, Chem. – Asian J., 2012, 7, 2854–2863 CrossRef CAS.
- X. Yang, Z. Zhao, H. Ran, J. Zhang, L. Chen, R. Han, X. Duan, H. Sun and J.-Y. Hu, Dyes Pigm., 2020, 173, 107881 CrossRef CAS.
- M.-T. Lee, H.-H. Chen, C.-H. Liao and C.-H. Tsai, Appl. Phys. Lett., 2004, 85, 3301–3303 CrossRef CAS.
-
M. J. Frisch, G. W. Trucks, H. B. Schlegel, G. E. Scuseria, M. A. Robb, J. R. Cheeseman, G. Scalmani, V. Barone, G. A. Petersson, H. Nakatsuji, X. Li, M. Caricato, A. V. Marenich, J. Bloino, B. G. Janesko, R. Gomperts, B. Mennucci, H. P. Hratchian, J. V. Ortiz, A. F. Izmaylov, J. L. Sonnenberg, D. Williams-Young, F. Ding, F. Lipparini, F. Egidi, J. Goings, B. Peng, A. Petrone, T. Henderson, D. Ranasinghe, V. G. Zakrzewski, J. Gao, N. Rega, G. Zheng, W. Liang, M. Hada, M. Ehara, K. Toyota, R. Fukuda, J. Hasegawa, M. Ishida, T. Nakajima, Y. Honda, O. Kitao, H. Nakai, T. Vreven, K. Throssell, J. A. Montgomery, Jr., J. E. Peralta, F. Ogliaro, M. J. Bearpark, J. J. Heyd, E. N. Brothers, K. N. Kudin, V. N. Staroverov, T. A. Keith, R. Kobayashi, J. Normand, K. Raghavachari, A. P. Rendell, J. C. Burant, S. S. Iyengar, J. Tomasi, M. Cossi, J. M. Millam, M. Klene, C. Adamo, R. Cammi, J. W. Ochterski, R. L. Martin, K. Morokuma, O. Farkas, J. B. Foresman and D. J. Fox, Gaussian 16, Revision A.03, Gaussian, Inc., Wallingford CT, 2016 Search PubMed.
- CCDC 1992133 for 3b contain the supplementary crystallographic data for this paper†.
- C.-T. Chen, Chem. Mater., 2004, 16, 4389–4400 CrossRef CAS.
- B. Heyne, Photochem. Photobiol. Sci., 2016, 15, 1103–1114 RSC.
- A. G. Crawford, A. D. Dwyer, Z. Liu, A. Steffen, A. Beeby, L. O. Palsson, D. J. Tozer and T. B. Marder, J. Am. Chem. Soc., 2011, 133, 13349–13362 CrossRef CAS.
- P. Tyagi, A. Venkateswararao and K. R. J. Thomas, J. Org. Chem., 2011, 76, 4571–4581 CrossRef CAS.
- E. Lippert, Phys. Sci., 1955, 10, 541–545 Search PubMed.
- N. Mataga, Y. Kaifu and M. Koizumi, Bull. Chem. Soc. Jpn., 1956, 29, 465–470 CrossRef CAS.
- C. W. Tang, S. A. Vanslyke and C. H. Chen, J. Appl. Phys., 1989, 65, 3610–3616 CrossRef CAS.
- J.-Y. Hu, M. Era, M. R. J. Elsegood and T. Yamato, Eur. J. Org. Chem., 2010, 72–79 CrossRef.
- H. Sasabe, E. Gonmori, T. Chiba, Y.-J. Li, D. Tanaka, S.-J. Su, T. Takeda, Y.-J. Pu, K. Nakayama and J. Kido, Chem. Mater., 2008, 20, 5951–5953 CrossRef CAS.
- N. Chopra, J. Lee, Y. Zheng, S.-H. Eom, J. Xue and F. So, ACS Appl. Mater. Interfaces, 2009, 1, 1169–1172 CrossRef CAS.
- Y.-J. Pu, G. Nakata, F. Satoh, H. Sasabe, D. Yokoyama and J. Kido, Adv. Mater., 2012, 24, 1765–1770 CrossRef CAS.
-
C.-C. Yeh, M.-T. Lee, H.-H. Chen and C. H. Chen, SID 04 DIGEST, 2004, pp. 789–791 Search PubMed.
- Z. Li, G. Gan, Z. Ling, K. Guo, C. Si, X. Lv, H. Wang, B. Wei and Y. Hao, Org. Electron., 2019, 66, 24–31 CrossRef CAS.
Footnotes |
† Electronic supplementary information (ESI) available: Details of DFT calculations, single-crystal X-ray structures, FT-IR spectra, PL spectra, 1H/13C NMR spectra and MS spectra. CCDC 1992133. For ESI and crystallographic data in CIF or other electronic format see DOI: 10.1039/d0tc03612a |
‡ H. Ran and Z. Zhao contributed equally to this work. |
|
This journal is © The Royal Society of Chemistry 2021 |
Click here to see how this site uses Cookies. View our privacy policy here.