Template-free, controllable and scalable synthesis of poly(5-aminoindole) nanoparticles for printable electrochemical immunosensor with ultra-high sensitivity†
Received
11th September 2021
, Accepted 16th November 2021
First published on 16th November 2021
Abstract
Conductive polymer polyindole derivatives have good conductivities and abundant functional groups, which would offer great potential for versatile applications including biosensors, bioelectronics and energy devices. However, the polyindole derivatives are mainly synthesized by the electropolymerization method on conductive electrode surfaces, which limits large-scale synthesis and practical applications. Herein, we explore a strategy of template-free, controllable and scalable synthesis of poly-5-aminoindole (PIn-5-NH2) nanoparticles (NPs) and demonstrate the application of PIn-5-NH2 NPs in printable multiplexed electrochemical biosensors with ultra-high sensitivity. The synthesis of PIn-5-NH2 NPs is based on a self-templated method since the In-5-NH2 monomer with amphiphilic structures can form micelles by self-assembly in an aqueous solution. The diameter of PIn-5-NH2 NPs could be controlled by adjusting the synthesis conditions, such as monomer concentration, oxidant/monomer ratio and reaction time. The PIn-5-NH2 NPs possess distinct features, including good conductivity, large surface area, and abundant –NH2 functional groups for covalent binding of the antibody, and therefore offer substantial possibilities for developing an all-printable process to fabricate multiplexed electrochemical immunosensors. The printed multiplexed electrochemical immunosensors on the basis of the aqueous suspension of PIn-5-NH2 NPs linked with antibodies can simultaneously detect multiple cancer markers, and exhibit high sensitivity and good selectivity. Our facile and scalable synthesis strategy would offer great opportunities for versatile applications of PIn-5-NH2 NPs.
1. Introduction
The conductive polymer nanoparticles with controlled morphology and engineered properties have attracted much attention for various applications in biosensors,1–3 supercapacitors,4–6 catalysis,7,8 photothermal therapy,9 drug delivery10 and molecular imaging.11,12 The synthesis of conductive polymer nanoparticles is mainly based on the template methods, including the hard-template method and soft-template method. For example, Luo et al. synthesized poly(3,4-ethylenedioxythiophene) (PEDOT) hollow nanoparticles using polystyrene as a hard-template,13 and Hong et al. synthesized polypyrrole (PPy) nanoparticles using myristyl trimethyl ammonium bromide as a soft-template.14 The template method typically requires post-treatment to remove the template, which not only needs a complicated and time-consuming process but also may destroy nanostructures and properties.15–17 Up to now, large-scale controllable synthesis of conductive polymer nanoparticles via a facile, template-free, and green process has remained a huge challenge.
Poly-5-aminoindole (PIn-5-NH2), one of the polyindole derivatives, has great potential in electrochemical biosensor applications due to its distinctive features such as good conductivity and abundant –NH2 groups for the immobilization of biomolecules, which are not possessed by other conductive polymers such as PEDOT, PPy and polyaniline (PAni). Yue et al. synthesized a graphene–poly(5-aminoindole) composite film as a Pt catalyst support for methanol electrooxidation in an alkaline medium.18 Moreover, Yue et al. synthesized poly(5-aminoindole) and explored the application as a catalyst support for formic acid electrooxidation.19 M. Düdükcü et al. synthesized poly(5-aminoindole) on stainless steel surfaces for corrosion inhibition.21 It should be noted that, in all these works, PIn-5-NH2 was mainly synthesized by the electropolymerization method on conductive electrode surfaces, which limits large-scale synthesis and broad application of PIn-5-NH2.18–22 Recently, Richa Mishra et al. proposed an interface chemical synthesis method for the synthesis of PIn-5-NH2.23 However, this interface chemical synthesis is not scalable, and the synthesis process is not environment-friendly since a lot of chloroform solutions need to be used in this method. Therefore, the exploration of a facile and scalable synthesis of PIn-5-NH2 nanomaterials is highly demanded for practical applications.
Herein, we explore a template-free, controllable and scalable synthesis of PIn-5-NH2 nanoparticles (NPs) and demonstrate the application of PIn-5-NH2 NPs in printable multiplexed electrochemical immunosensors. In our novel synthesis strategy, deionized water is used as a solvent, H2O2 is used as an oxidant, no template or any other organic reagents are used, and the reaction is carried out at room temperature. Moreover, the synthesis of PIn-5-NH2 nanoparticles with controllable size can be achieved by adjusting the oxidant/monomer ratio, monomer concentration and reaction time. The formation of PIn-5-NH2 NPs should be attributed to the amphiphilic structures of 5-aminoindole (In-5-NH2), which could be easy to form micelles by self-assembly. As a demonstration of the potential application of PIn-5-NH2 NPs with the capability of large-scale production, all-printable multiplexed electrochemical immunosensors are fabricated on the basis of the aqueous suspension of the PIn-5-NH2 NPs linked with antibodies. With alpha fetoprotein (AFP) and carcinoembryonic antigen (CEA) as model biomarkers, the printed multiplexed label-free electrochemical immunosensors exhibit high sensitivity and good selectivity. This contribution would offer great opportunities for the scalable production of all-printable electrochemical immunosensors for clinical applications.
2. Experimental
2.1 Chemicals and materials
The 5-aminoindole (In-5-NH2) monomer was purchased from Shanghai Vita Chemical Regent Co., Ltd (Shanghai, China). Analytical-grade hydrogen peroxide (30 wt%) used as an oxidant was purchased from Sinopharm Chemical Reagent Co., Ltd. Graphene oxide (GO) was purchased from Nanjing Xian Feng Nanomaterials Technology Co., Ltd (Nanjing, China). All of the cancer biomarkers were purchased from Bosai Biotechnology Co., Ltd (Zhengzhou, China). Human serum samples were purchased from Guangzhou hongquan bio-tel. (Guangzhou, China). All of the interfering species and activation reagents of EDC, NHS and MES were purchased from J&K Scientific Ltd (Beijing China).
2.2 Apparatus and measurements
Scanning electron microscope (SEM) images were captured using a JSM-7500F scanning electron microscope. Fourier transform infrared (FT-IR) spectra were collected using a Nicolet Magna-IR 750. Transmission electron microscopy (TEM) characterization was performed using a HITACHI Transmission Electron Microscope, HT7700, operating at 100 kV. The electrochemical property measurements were performed on a CHI660E electrochemical workstation (Shanghai Chenhua Apparatus Corporation, China) with a standard three-electrode system. A platinum sheet electrode (5 mm × 5 mm) was used as the counter electrode, a saturated calomel reference electrode (SCE) was used as the reference electrode, and a bare glassy carbon electrode (GCE; Φ = 3 mm) was used as the working electrode.
2.3 Synthesis of PIn-5-NH2 nanoparticles
In a typical synthesis process, the 5-aminoindole monomer was dispersed in deionized water (6.7 mL) at different concentrations from 0.1 to 1 mg mL−1 (based on the total reaction solution volume of 20 mL) and sonicated for 5 min. Then, the solution was stirred magnetically for 30 min to form a uniform solution. 13.3 ml H2O2 with different concentrations (8 wt%; 12 wt%; 16 wt%; 20 wt%) were poured into a 5-aminoindole monomer solution. The mixture was allowed to react at different times (8 h, 16 h, 24 h) at room temperature. After completion of the reaction, the products were washed several times with water and ethanol. PIn-5-NH2 NPs were dispersed in deionized water at a concentration of about 0.5 mg mL−1.
2.4 Fabrication of electrochemical immunosensors based on PIn-5-NH2 nanoparticles
The PIn-5-NH2 NP solution (0.5 mg mL−1, 5 mL) was mixed with GO (0.5 mg mL−1, 5 mL), hydrazine solution (5.0 μL, 35 wt%) and ammonia solution (35.0 μL, 28 wt%) in a 20 mL round-bottom flask. After sonicating for 30 min, the mixed solution was placed in an oil bath at 95 °C for 40 min. Finally, the excess hydrazine and ammonia in the resulting dispersions were removed by dialysis for about 24 h. GO was added to the PIn-5-NH2 NPs solution could improve the dispersion of PIn-5-NH2 NPs and avoid agglomeration. In addition, after GO was reduced to rGO, the conductivity of the composite material can be increased, thereby increasing the electrochemical signal. PIn-5-NH2 NPs/rGO was diluted with deionized water (25 mL), and the concentration was estimated to be 0.2 mg mL−1. PIn-5-NH2 NPs/rGO suspension (8 μL; 0.2 mg mL−1) was dropped on the bare GCE and dried in an infrared drying oven. Then, the modified electrodes were immersed in a mixing solution containing EDC (100 mM), NHS (40 mM) and MES (100 mM) for 12 h to activate the –NH2 group on the PIn-5-NH2 NPs, and then washed with deionized water twice. After that, 8 μL of anti-AFP antibody was dropped onto the modified electrode and incubated in a 37 °C water bath for 1 h. The electrode was washed with PBS, and then 8 μL of BSA (1 mg mL−1) was dropped onto the modified electrode for 30 min to block the residual active sites. Then, the immunosensor electrode was obtained after washing with deionized water. The prepared PIn-5-NH2 NPs/anti-AFP/BSA/rGO/GCE immunosensor electrode was stored in a 4 °C refrigerator when not used.
2.5 Fabrication of printable multiplexed electrochemical immunosensors
In order to bind the antibody to the PIn-5-NH2 NPs/rGO nanocomposite through the –NH2 group, EDC (100 mM), NHS (40 mM) and MES (100 mM) were added to the PIn-5-NH2 NPs/rGO dispersion to activate the amino group for 12 h, and then the antibody (anti-AFP and anti-CEA) was added to the PIn-5-NH2 NPs/rGO dispersion and incubated in a 37 °C water bath for 2 h. The samples were centrifuged and washed to remove the excess unfixed antibody. Then, BSA (1 mg mL−1) was added to the PIn-5-NH2NPs/antibody/rGO dispersion to block the excess fixed site. After centrifugation and washing, the concentration of PIn-5-NH2 NPs/antibody/BSA/rGO was adjusted to 0.2 mg mL−1. Then, PIn-5-NH2 NPs/antibody/BSA/rGO was used as a sensing material for printable electrochemical immunosensors. The all-printing process for biosensors was performed on the basis of electrohydrodynamic jet printing technology. Firstly, the conductive circuits and electrodes were printed on PET sheets with Au NPs ink, and the printed Au electrodes were annealed at 150 °C for 1 hour. Next, a SU8 dielectric polymer was printed onto the conductive circuits as an insulating layer. Then, an Ag/AgCl reference electrode was prepared by printing Ag NPs ink onto the Au electrode and subsequent chloridization with a hydrochloric acid solution. Finally, PIn-5-NH2 NPs/anti-AFP/BSA/rGO and PIn-5-NH2 NPs/anti-CEA/BSA/rGO were printed onto the working electrodes to prepare multiplexed electrochemical immunosensors.
3. Results and discussion
3.1 Synthesis and characterization of PIn-5-NH2 nanoparticles
The morphology of the as-synthesized PIn-5-NH2 NPs was characterized using SEM (Fig. 1A–C), and the particle size distribution of PIn-5-NH2 NPs was statistically analyzed. The concentration of H2O2 has a great influence on the particle size of PIn-5-NH2 NPs. Fig. 1D shows the influence of the concentration of H2O2 on the particle size of PIn-5-NH2 NPs. The diameter of PIn-5-NH2 NPs was gradually reduced from 846 ± 69 nm to 487 ± 53 nm with the concentration of H2O2 increasing from 8 wt% to 20 wt%. In addition to H2O2, three other oxidants include (NH4)2S2O8, Fe2(SO4)3 and CuCl2 can also be used to prepare PIn-5-NH2 NPs. Fig. S1 (ESI†) displays SEM images of PIn-5-NH2 NPs synthesized using (NH4)2S2O8, Fe2(SO4)3 and CuCl2. The diameter of PIn-5-NH2 NPs prepared by (NH4)2S2O8, Fe2(SO4)3 and CuCl2 is much smaller than that prepared using H2O2, but the homogeneity of the particle size is poor. Fig. 1E shows a photograph of the aqueous suspension of PIn-5-NH2 NPs prepared by the chemical polymerization method using H2O2 as the oxidant, indicating a potential large-scale production.
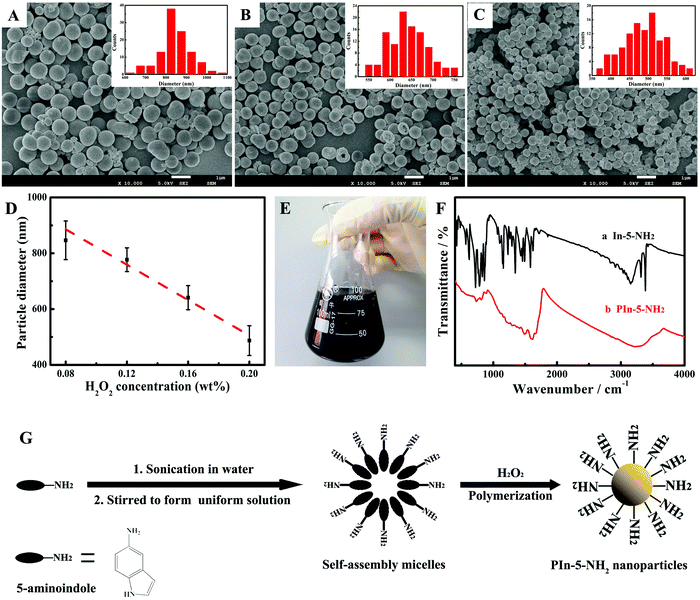 |
| Fig. 1 Template-free and scalable synthesis of PIn-5-NH2 NPs with the green process. (A–C) The SEM images of PIn-5-NH2 NPs synthesized with different concentrations of H2O2: (A) 8 wt%; (B) 16 wt%; (C) 20 wt%. The inset shows the statistical distribution of the diameters of PIn-5-NH2 NPs. (D) Statistics on the PIn-5-NH2 NP size as a function of H2O2 concentration. (E) A photograph showing the aqueous suspension of PIn-5-NH2 NPs. (F) FT-IR spectra of In-5-NH2 monomer and PIn-5-NH2 NP suspension. The concentration of the In-5-NH2 monomer was 0.1 mg mL−1. (G) The formation of In-5-NH2 micelles by self-assembly for the fabrication of PIn-5-NH2 NPs. | |
FT-IR spectra of the In-5-NH2 monomer and PIn-5-NH2 polymer were recorded to study the polymerization mechanism. As shown in Fig. 1F, the absorption peaks of PIn-5-NH2 are obviously broadened when compared with those of In-5-NH2 monomer, suggesting successful chemical polymerization of the In-5-NH2 monomer.24,25 The absorption peaks between 720 cm−1 and 861 cm−1 in the spectrum of PIn-5-NH2 can be assigned to the three adjacent C–H deformation vibration of ring hydrogens on the benzenic ring, which indicates that benzene rings are 1,2,3-trisubstitution.26 The absorption peak at 1628 cm−1 can be assigned to the –NH2 scissoring vibration (N–H bending), which appears in both In-5-NH2 and PIn-5-NH2, indicating that the –NH2 group is not involved in polymerization.23 In the pyrrole ring, the
CH–N stretching band appeared at 890 cm−1 for the In-5-NH2 monomer and PIn-5-NH2, indicating that the polymerization should not happen at the 2 position. While the N–H stretching vibration of the pyrrole ring was located at approximately 3385 cm−1 for the In-5-NH2 monomer and disappeared for PIn-5-NH2.23 According to the above results, the polymerization should happen at the 1 and 3 positions. Regarding the mechanism of nanoparticle formation, the self-assembled soft-template of the In-5-NH2 monomer resulting from the amphiphilic structure of In-5-NH2 is a rational explanation. In In-5-NH2, the amino-functional group is hydrophilic and the macrocyclic structure at the other end is hydrophobic. Fig. 1G shows the self-templating process with self-assembled In-5-NH2 micelles in an aqueous solution facilitating the formation of PIn-5-NH2 NPs. Similar amphiphilic characteristics are also observed in the study of aniline, which has a hydrophobic benzene ring and a hydrophilic –NH2 group.
In order to achieve a controllable synthesis of PIn-5-NH2 NPs, the effects of other factors including monomer concentration, ethanol volume and reaction time on the particle size of PIn-5-NH2 NPs were systematically studied. Fig. 2A shows the influence of the concentration of the In-5-NH2 monomer on the particle size of PIn-5-NH2 NPs. The results of SEM images and statistics of particle size distribution indicate that the size of PIn-5-NH2 NPs gradually increases from 327 nm to 744 nm with the concentration of the In-5-NH2 monomer increasing from 0.1 mg mL−1 to 1 mg mL−1. Since In-5-NH2 monomers can form micelles in the aqueous solution, the In-5-NH2 monomers with higher concentrations would form larger micelles, resulting in PIn-5-NH2 NPs with larger size. Ethanol can increase the solubility of the In-5-NH2 monomer in the reaction system. As shown in Fig. 2B, with the volume fraction of ethanol increasing from 2.5% to 25%, the size of PIn-5-NH2 NPs increased from 599 nm to 918 nm. Moreover, ethanol has a negative effect on the preparation of PIn-5-NH2 nanoparticles with good size uniformity. Regarding the reaction time, we note that when the reaction time was extended from 8 h to 24 h, there was a decrease in the diameter of PIn-5-NH2 NPs from 581 ± 52 nm to 442 ± 41 nm, and the diameter of PIn-5-NH2 NPs achieved to a stable size at about 16 h, which is the optimal reaction time in our synthesis.
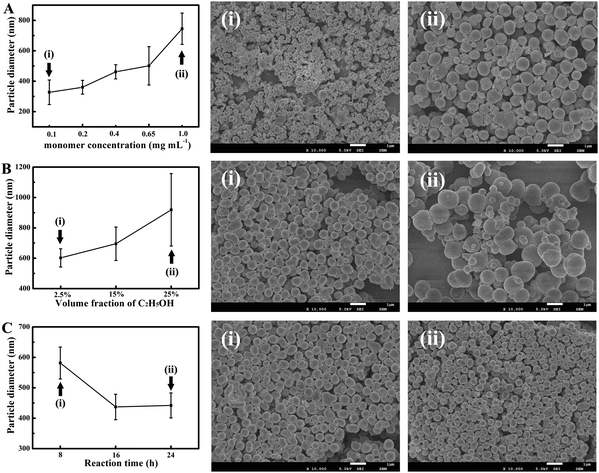 |
| Fig. 2 Effect of monomer concentration, the volume of ethanol and reaction time on the size of PIn-5-NH2 NPs. (A) Concentration of the In-5-NH2 monomer: 0.1 mg mL−1; 0.2 mg mL−1; 0.4 mg mL−1; 0.65 mg mL−1; 1 mg mL−1; H2O2 wt% = 20%; (B) volume fraction of ethanol: 2.5%; 15%; 25%; In-5-NH2 monomer: 0.1 mg mL−1; H2O2 wt% = 20%; total reaction volume = 20 mL; (C) reaction time: 8 h; 16 h; 24 h; In-5-NH2 monomer: 0.1 mg mL−1; H2O2 wt% = 20%. Reaction temperature = 25 °C. | |
3.2 Construction and characterization of the PIn-5-NH2 based electrochemical immunosensor
Fig. 3A illustrates the construction process of the electrochemical immunosensor based on PIn-5-NH2 NPs. The antibody was immobilized to PIn-5-NH2 NPs with the EDC/NHS reaction, and rGO was added to improve the stability of PIn-5-NH2 NPs suspension and subsequent conductivity of the immunosensor. Fig. 3B and C show cyclic voltammetry (CV) and electrochemical impedance spectra (EIS) of each step during the construction of the electrochemical immunosensor, respectively. The PIn-5-NH2 NPs/rGO/GCE electrode displays a good current (142.1 μA, Fig. 4A, curve a) and low resistance (30 Ω, Fig. 4B, curve a). When anti-AFP was immobilized onto PIn-5-NH2 NPs/rGO/GCE, the peak current severely decreased from 142.1 μA to 108.7 μA, and Ret significantly increased from 30 Ω to 259 Ω. These results indicate that the anti-AFP was successfully bonded onto PIn-5-NH2 NPs (Fig. 4A and B, curve b). BSA was subsequently used to block non-specific sites, and then the peak current decreases and Ret increases again (Fig. 4A and B, curve c). For AFP detection, the AFP antigen was incubated to the immunosensor to form an insulating antigen–antibody complex (Fig. 4A and B, curve d), which results in a dramatic decrease of the peak current in CV and increase of Ret. All the CV and EIS results confirm that the PIn-5-NH2 NPs/anti-AFP/BSA/rGO/GCE immunosensor was successfully fabricated.
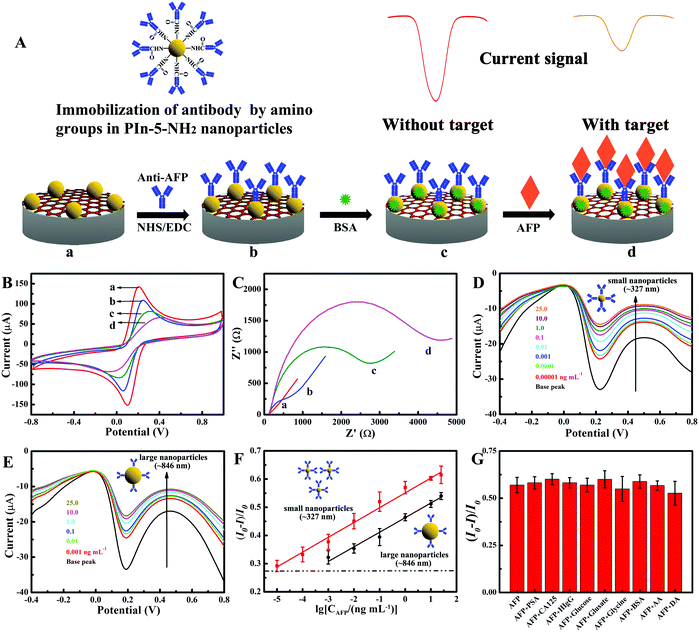 |
| Fig. 3 The electrochemical characterization of PIn-5-NH2 based immunosensors. (A) Schematic illustration of the fabrication procedure of the immunosensor based on PIn-5-NH2 NPs. (B) CV and (C) EIS of PIn-5-NH2/rGO/GCE (a), PIn-5-NH2 NPs/anti-AFP/rGO/GCE (b), PIn-5-NH2/anti-AFP/BSA/rGO/GCE (c) and modified GCE after incubation with 1 ng mL−1 AFP (d). CVs and EIS: 5.0 mM K3Fe(CN)6/K4Fe(CN)6 (1 : 1) containing 0.1 M KCl; scan rate: 100 mV s−1; frequency range: 0.1–10 000 Hz; signal amplitude: 0.5 mV. DPV responses of the PIn-5-NH2 NPs/anti-AFP/BSA/rGO/GCE immunosensors with (D) 327 nm PIn-5-NH2 NPs and (E) 846 nm PIn-5-NH2 NPs. (F) Calibration curves of PIn-5-NH2 NPs/anti-AFP/BSA/rGO/GCE immunosensors with 327 nm and 846 nm PIn-5-NH2 NPs. (G) Interference test of PIn-5-NH2 NPs/anti-AFP/BSA/rGO/GCE immunosensors upon the addition of different analytes, showing of the response of immunosensor to 1 ng mL−1 AFP, 1 ng mL−1 AFP + 100 ng mL−1 PSA, 1 ng mL−1 AFP + 100 U mL−1 CA125, 1 ng mL−1 AFP + 100 ng mL−1 HIgG, 1 ng mL−1 AFP + 1 mg mL−1 glucose, 1 ng mL−1 AFP + 1 mg mL−1 glusate, 1 ng mL−1 AFP + 1 mg mL−1 glycine, 1 ng mL−1 AFP + 50 ng mL−1 BSA, 1 ng mL−1 AFP + 1 mg mL−1 ascorbic acid, 1 ng mL−1 AFP + 200 ng mL−1 dopamine. | |
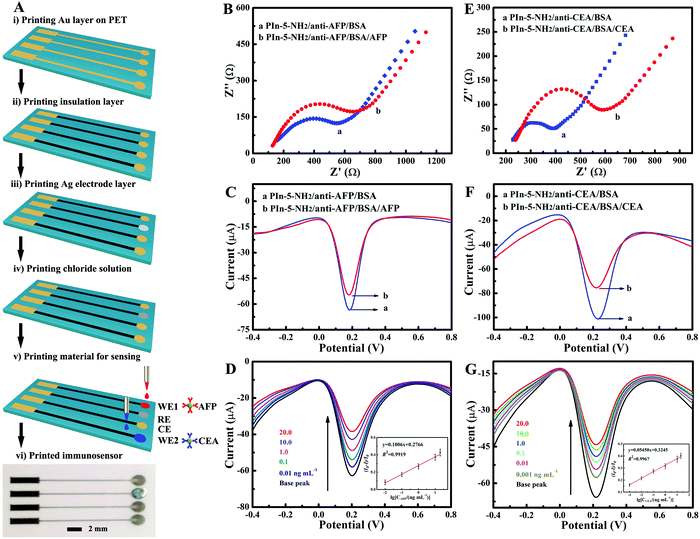 |
| Fig. 4 The printed multiplex electrochemical immunosensors based on PIn-5-NH2 NPs. (A) Schematic illustration of the fabrication process of printable immunosensors. (i) Printing conductive circuits and electrodes with Au NP ink on the PET substrate. (ii) Printing SU8 insulation layer on the Au circuits. (iii) Printing the Ag electrode layer for the reference electrode. (iv) Printing chloride solution to prepare the Ag/AgCl reference electrode. (v) Printing PIn-5-NH2/anti-AFP/BSA/rGO and PIn-5-NH2/anti-CEA/BSA/rGO active nanomaterials for working electrodes. (vi) A photograph of the printed multiplex electrochemical immunosensors. The diameter of the working electrodes (WE1, WE2), reference electrode (RE) and counter electrode (CE) are all 2 mm. (B) EIS, (C) DPV and (D) sensing performance for the AFP detection using the printed PIn-5-NH2/anti-AFP/BSA/rGO electrochemical immunosensors. (E) EIS, (F) DPV and (G) sensing performance of CEA detection using the printed PIn-5-NH2/anti-CEA/BSA/rGO electrochemical immunosensors. | |
The fabrication process of PIn-5-NH2 NPs/anti-AFP/BSA/rGO/GCE immunosensor, including incubation time of antibody and antigen, and the mass ratio of rGO to PIn-5-NH2 NPs, was carefully optimized to improve the immunoassay performance. Fig. S2(A) (ESI†) shows the effect of the incubation time of the antibody on the analytical performance of immunosensors. The inhibition ratio increases with incubation time increased from 15 min to 60 min and decreases with the incubation time increasing from 60 min to 120 min. Therefore, 60 min was chosen as the optimal incubation time for antibody anti-AFP. Fig. S2(B) (ESI†) displays the inhibition ratio as a function of AFP antigen incubation time, which indicates that 120 min is the optimal incubation time for AFP antigen. Fig. S2(C) (ESI†) shows the inhibition ratio as a function of graphene concentration, which indicates that 0.1 mg mL−1 is the optimal concentration. Meanwhile, the mass ratio of graphene and PIn-5-NH2 NPs were optimized. When the mass ratio of rGO to PIn-5-NH2 NPs was 1
:
1, the inhibition ratio was the largest (Fig. S2(D), ESI†).
Next, the influence of the size of PIn-5-NH2 NPs on immunoassay performance was investigated. Fig. 4D and E show the differential pulse voltammetry (DPV) responses of the PIn-5-NH2 NPs/anti-AFP/BSA/rGO/GCE immunosensors with 327 nm and 846 nm PIn-5-NH2 NPs, respectively. The calibration curves of PIn-5-NH2 NPs/anti-AFP/BSA/rGO/GCE immunosensors with 327 nm and 846 nm PIn-5-NH2 NPs are shown in Fig. 4F. The linear regression equation could be expressed as (I0 − I)/I0 = 0.05261
lg
CAFP + 0.5512, (R2 = 0.9943; n = 5) for the immunosensor with 327 nm PIn-5-NH2 NPs, and (I0 − I)/I0 = 0.05189
lg
CAFP + 0.4633, (R2 = 0.9910; n = 5) for the immunosensor with 846 nm PIn-5-NH2 NPs. The linear range and detection limit of the immunosensors with 327 nm PIn-5-NH2 NPs are 0.01 pg mL−1 to 25 ng mL−1, and 3.3 fg mL−1 (S/N = 3), respectively. The linear range and detection limit of the immunosensors with 846 nm PIn-5-NH2 NPs are 1 pg mL−1 to 25 ng mL−1, and 330 fg mL−1 (S/N = 3), respectively. Therefore, the detection limit of PIn-5-NH2/anti-AFP/BSA/rGO/GCE immunosensor with 327 nm PIn-5-NH2 NPs is two orders of magnitude lower than that of PIn-5-NH2/anti-AFP/BSA/rGO/GCE immunosensor with 846 nm PIn-5-NH2 NPs, which may result from the larger specific surface area of 327 nm PIn-5-NH2 NPs and therefore more amino groups for immobilization of more antibodies. In comparison with other AFP immunosensors reported in the literature, our PIn-5-NH2 NPs/anti-AFP/BSA/rGO/GCE immunosensors exhibit much better immunoassay performance in the linear range and detection limit (Table 1).
Table 1 Comparison of analytical performances of different AFP immunosensors
Materials |
Linear range (ng mL−1) |
Detection limit (pg mL−1) |
Ref. |
PEDOT functionalized with hyperbranched polyglycerol.
Poly(2-hydroxyethyl methacrylate)/graphene oxide nanocomposite.
Polyamidoamine dendrimer.
Gold nanoparticles–dextran–reduced graphene oxide.
|
PIn-5-NH2 NPs/rGO |
0.00001–25 |
0.0033 |
This work |
FeS2–AuNPs |
0.0001–100 |
0.028 |
27
|
PEDOT–HPGa |
0.0001–1.0 |
0.035 |
28
|
PHEMA/GOb |
0.0025–25 |
0.403 |
29
|
Cu3Pt nanoframes |
0.0001–10 |
0.033 |
30
|
AuNPs/HRP |
0.0001–10 |
0.03 |
31
|
Pd@Ag@CeO2 |
0.0004–100 |
0.13 |
32
|
Polydopamine nanoparticles |
0.001–50 |
0.3 |
33
|
Au@PAMAMc–C60 |
0.0001–10 |
0.03 |
34
|
Fe3O4@PS/PDA–Au nanotubes |
0.01–100 |
8.8 |
35
|
AuNPs–Dex–rGOd |
0.01–20 |
0.05 |
36
|
Co3O4@MnO2–thionine |
0.001–100 |
0.33 |
37
|
In order to investigate the specificity of the PIn-5-NH2 NPs/anti-AFP/BSA/rGO/GCE immunosensor, several interference substrates were chosen for control experiments (Fig. 4G). There is an obvious current response to the addition of 1.0 ng mL−1 AFP, while there is less inhibition ratio to the addition of 100 ng mL−1 PSA, 100 U mL−1 CA125, 100 ng mL−1 HIgG, 1 mg mL−1 glucose, 1 mg mL−1 glusate, 1 mg mL−1 glycine, 50 ng mL−1 BSA, 1 mg mL−1 ascorbic acid, 200 ng mL−1 dopamine in the same sample. These results indicate that the selectivity of the as-prepared immunosensor is excellent. The stability of the immunosensor was also investigated (Fig. S2(E), ESI†). Five immunosensors were stored in PBS (pH = 7.0) at 4 °C, and their performances were measured every week. The current response retained 94.89% and 85.40% of its initial current after one week and three weeks, respectively, which indicates that the immunosensors have acceptable long-term stability. To investigate the repeatability of the immunosensor (Fig. S2(F), ESI†), five fresh PIn-5-NH2 NPs/anti-AFP/BSA/rGO/GCE immunosensors were prepared using the same procedure for the detection of AFP (8 μL, 1 ng mL−1). The relative standard deviations (RSD) of the five measurements were 3.4363%, indicating good reproducibility. To evaluate the practicability of the immunosensors in real sample detection, recovery experiments are performed by standard addition methods in human serum samples. The results displayed in Table S1 (ESI†) show that the recovery is in the range of 97.3% to 110.0%, indicating that immunosensors have great potential for detection of AFP in real clinical samples.
3.3 The printable electrochemical immunosensor based on PIn-5-NH2 NPs
Multiplexed detection of several antigens simultaneously within a single immunosensor chip is highly demanded in clinical diagnosis with small quantities of blood samples. However, the traditional layer-by-layer dip-coating method described in the last section has great limitations in the low-cost and massive fabrication of electrochemical immunosensors equipped with multiple types of antibodies with distinct electrodes. Recently, several efforts have been reported to develop all-printing techniques with high-throughput deposition of active compounds in precise spatial control for multiplexed electrochemical biosensors. Nevertheless, for construction of printable electrochemical immunosensors, the printable electroactive nanomaterials immobilized with antibodies remain one of the major challenges. With the advantages of scalable synthesis, good conductivity and abundant –NH2 groups, PIn-5-NH2 NPs linked with antibodies show substantial possibilities for the printing process to fabricate multiplexed electrochemical immunosensors. As a proof-of-concept, the PIn-5-NH2 NPs based electrochemical immunosensor, which has two channels for simultaneous detection of AFP and CEA was explored (Fig. 4A). The multiplexed electrochemical immunosensors are printed on polyethylene terephthalate (PET) substrates with an electrohydrodynamic jet printing technique. The printed electrochemical immunosensor consists of two working electrodes (WE1, WE2), a shared Ag/AgCl reference electrode (RE), and a shared Au counter electrode (CE). The conductive circuits and electrodes are printed with Au NPs ink, and a SU8 dielectric polymer is printed onto the conductive circuits as the insulating layer. For the fabrication of the Ag/AgCl electrode, Ag NPs ink was printed onto the Au electrode and subsequently, the hydrochloric acid solution was applied to prepare Ag/AgCl RE. Without any chemical modification of the working electrodes, the prepared suspension of PIn-5-NH2 NPs/anti-AFP/BSA/rGO and PIn-5-NH2 NPs/anti-CEA/BSA/rGO was printed to prepare the sensing electrodes of immunosensors.
The detection process of the printed electrochemical immunosensors was monitored using EIS and DPV measurements. Fig. 4B and C show the EIS and DPV of PIn-5-NH2 NPs/anti-AFP/BSA/rGO immunosensors for AFP detection, and Fig. 4E and F show that the EIS and DPV of PIn-5-NH2 NPs/anti-CEA/BSA/rGO immunosensor for CEA detection. The Ret of PIn-5-NH2 NPs/anti-AFP/BSA/rGO immunosensor was 194 Ω, and it increased to 276 Ω after the incubation of AFP antigen. The DPV of PIn-5-NH2 NPs/anti-AFP/BSA/rGO immunosensor displays a remarkable peak current (54.39 μA), and the peak current decreases to 46.04 μA when the insulative antigen–antibody complex was formed for AFP detection. Similarly, after CEA incubation, Ret of PIn-5-NH2 NPs/anti-CEA/BSA/rGO immunosensor increases from 81 Ω to 174 Ω, and DPV peak current of PIn-5-NH2 NPs/anti-CEA/BSA/rGO immunosensor decreases from 79.59 μA to 52.34 μA. The immunoassay performance of the PIn-5-NH2 NPs/anti-CEA/BSA/rGO immunosensor for AFP detection is shown in Fig. 4D. The linear regression equation of inhibition ratio of the current response with AFP concentration could be expressed as (I0 − I)/I0 = 0.1006
lg
CAFP + 0.2766, (R2 = 0.9919; n = 5). The linear range is from 0.01 ng mL−1 to 20 ng mL−1 with a detection limit of 3.3 pg mL−1 (S/N = 3). In addition, the linear regression equation for CEA detection could be expressed as (I0 − I)/I0 = 0.05450
lg
CCEA + 0.3245, (R2 = 0.9967; n = 5). The linear range of PIn-5-NH2 NPs/anti-CEA/BSA/rGO immunosensor was from 0.001 ng mL−1 to 20 ng mL−1 with an excellent detection limit of 0.33 pg mL−1 (S/N = 3). All these results indicate that the printed electrochemical immunosensors based on PIn-5-NH2 NPs have the capability for multiplexed detection of different antigens simultaneously within a single immunosensor chip, and possess good immunoassay performance similar to immunosensors fabricated with the time-consuming traditional method.
4. Conclusion
In summary, a template-free and green synthesis strategy was developed to produce PIn-5-NH2 NPs on a large scale, and the application of PIn-5-NH2 NPs in printable multiplexed electrochemical immunosensors was demonstrated. The synthesis of PIn-5-NH2 NPs was based on a self-templated method since the In-5-NH2 monomer with amphiphilic structures can form micelles by self-assembly in an aqueous solution. The diameter of PIn-5-NH2 NPs could be controlled by adjusting the synthesis conditions, such as the monomer concentration, oxidant/monomer ratio and reaction time. With the distinguished features of scalable synthesis, large surface area, good conductivity and abundant –NH2 groups for linking with antibodies, the PIn-5-NH2 NPs show substantial possibilities for developing an all-printing process to fabricate multiplexed electrochemical immunosensors. The printed electrochemical immunosensors based on PIn-5-NH2 NPs have the capability for multiplexed detection of different antigens simultaneously within a single immunosensor chip, and possess good immunoassay performance similar to the immunosensors fabricated with the time-consuming traditional method. The electrochemical immunosensors based on PIn-5-NH2 NPs have good sensitivity, specificity, stability and reproducibility, and show great promise for clinical applications. Based on the facile and scalable synthesis, PIn-5-NH2 NPs would promote the applications of polyindole derivatives in versatile research fields.
Conflicts of interest
The authors declare that they have no known competing financial interests or personal relationships that could have appeared to influence the work reported in this paper.
Acknowledgements
Zhiqiang Luo acknowledges the support of the National Natural Science Foundation of China (grant no. 81771974). Bin Wang acknowledges the support of the Sanming Project of Medicine in Shenzhen (grant no. SZSM201812055).
References
- O. Kwon, J. Hong, S. Park, Y. Jang and J. Jang, J. Phys. Chem. C, 2010, 114, 18874–18879 CrossRef CAS.
- F. Jiang, R. Yue, Y. Du, J. Xu and P. Yang, Biosens. Bioelectron., 2013, 44, 127–131 CrossRef CAS.
- H. Chang, H. Zhang, J. Lv, B. Zhang, W. L. Wei and J. G. Guo, Biosens. Bioelectron., 2016, 86, 156–163 CrossRef CAS.
- Z. Wang, C. Zhang, C. Xu, Z. Zhu and C. Chen, Ionics, 2017, 23, 147–156 CrossRef CAS.
- J. Zhang, Y. Yu, L. Liu and Y. Wu, Nanoscale, 2013, 5, 3052–3057 RSC.
- W. Fan, C. Zhang, W. Tjiu, K. Pramoda, C. He and T. Liu, ACS Appl. Mater. Interfaces, 2013, 5, 3382–3391 CrossRef CAS PubMed.
- B. Ma, S. Ghasimi, K. Landfester, F. Vilela and K. Zhang, J. Mater. Chem. A, 2015, 3, 16064–16071 RSC.
- J. Yan, L. Yang, M. Lin, J. Ma, X. Lu and P. Lee, Small, 2013, 9, 596–603 CrossRef CAS PubMed.
- J. L. Geng, C. Y. Sun, J. Liu, L. D. Liao, Y. Y. Yuan, N. Thakor, J. Wang and B. Liu, Small, 2015, 11, 1603–1610 CrossRef CAS.
- R. Liu, Y. Guo, G. Odusote, F. Qu and R. Priestley, ACS Appl. Mater. Interfaces, 2013, 5, 9167–9171 CrossRef CAS PubMed.
- X. Zhen, C. Zhang, X. Chen, Q. Miao, K. Lim and K. Pu, ACS Nano, 2016, 10, 6400–6409 CrossRef CAS PubMed.
- C. Xie, X. Zhen, Q. Miao, Y. Lyu and K. Pu, Adv. Mater., 2018, 30, 1801331 CrossRef PubMed.
- S. Luo, H. Yu, A. Wan, Y. Han and J. Ying, Small, 2008, 4, 2051–2058 CrossRef CAS.
- S. Jeon, C. Kim, J. Ko and S. Im, J. Mater. Chem., 2011, 21, 8146–8151 RSC.
- M. Wan, Adv. Mater., 2008, 20, 2926–2932 CrossRef CAS.
- Z. Wei, L. Zhang, M. Yu, Y. Yang and M. Wan, Adv. Mater., 2003, 15, 1382–1385 CrossRef CAS.
- L. Zhang and M. Wan, Adv. Funct. Mater., 2003, 13, 815–820 CrossRef CAS.
- R. Yue, Q. Zhang, C. Wang, Y. Du, P. Yang and J. Xu, Electrochim. Acta, 2013, 107, 292–300 CrossRef CAS.
- R. Yue, F. Jiang, Y. Du, J. Xu and P. Yang, Electrochim. Acta, 2012, 77, 29–38 CrossRef CAS.
- R. Yue, Z. Yao, J. Geng, Y. K. Du, J. Xu and P. Yang, J. Solid State Electrochem., 2013, 17, 751–760 CrossRef CAS.
- M. Düdükcü and G. Avcı, Prog. Org. Coat., 2016, 97, 110–114 CrossRef.
- B. Broda and G. Inzelt, Acta Chim. Slov., 2014, 61, 357–365 CAS.
- R. Mishra, S. Gupta, A. Kumar and R. Prakash, Mater. Chem. Phys., 2016, 183, 606–614 CrossRef CAS.
- Y. Hu, D. Hu, S. Ming, X. Duan, F. Zhao, J. Hou, J. Xu and F. Jiang, Electrochim. Acta, 2016, 189, 64–73 CrossRef CAS.
- H. Liu, S. Zhen, S. Ming, K. Lin, H. Gu, Y. Zhao, Y. Li, B. Lu and J. Xu, J. Solid State Electrochem., 2016, 20, 2337–2349 CrossRef CAS.
- X. Ma, W. Zhou, D. Mo, J. Hou and J. Xu, Electrochim. Acta, 2015, 176, 1302–1312 CrossRef CAS.
- L. Zhang, X. Xie, Y. Yuan, Y. Chai and R. Yuan, Electroanalysis, 2019, 31, 1–8 CrossRef.
- L. Ma, S. Jayachandran, Z. Li, Z. Song, W. Wang and X. Luo, J. Electroanal. Chem., 2019, 840, 272–278 CrossRef CAS.
- Y. Liang, X. Zhao, N. Wang, J. Wang, H. Chen, L. Bai and W. Wang, RSC Adv., 2019, 9, 17187 RSC.
- A. Wang, X. Zhu, Y. Chen, P. Yuan, X. Luo and J. Feng, Sens. Actuators, B, 2019, 288, 721–727 CrossRef CAS.
- D. Lu, Q. Xu, G. Pang and F. Lu, Biomed. Microdevices, 2018, 20, 46 CrossRef.
- X. Zhang, Y. Li, H. Lv, Z. Gao, C. Zhang, S. Zhang, Y. Wang, Z. Xu and Z. Zhao, J. Electrochem. Soc., 2018, 165, B931 CrossRef CAS.
- H. Xiang, Y. Wang, M. Wang, Y. Shao, Y. Jiao and Y. Zhu, Nanoscale, 2018, 10, 13572–13580 RSC.
- P. Chen, T. Wang, X. Zheng, D. Tian, F. Xia and C. Zhou, New J. Chem., 2018, 42, 4653–4660 RSC.
- F. Peng, M. Chu, J. Sun, Y. Liu, Q. Zhang, Y. Chen, F. Wang and W. Zhao, J. Electroanal. Chem., 2018, 814, 52–58 CrossRef CAS.
- J. Zhou, C. Zhang, Y. Chen, Z. Wang, L. Lan, Y. Wang, B. Han, M. Pan, J. Jiao and Q. Chen, J. Electroanal. Chem., 2019, 833, 126–132 CrossRef CAS.
- Y. Wang, G. Zhao, H. Wang, W. Cao, B. Du and Q. Wei, Biosens. Bioelectron., 2018, 106, 179–185 CrossRef CAS.
Footnotes |
† Electronic supplementary information (ESI) available: [DETAILS]. See DOI: 10.1039/d1tb01998h |
‡ These authors contributed equally. |
|
This journal is © The Royal Society of Chemistry 2022 |