Integration of IR-808 and thiol-capped Au–Bi bimetallic nanoparticles for NIR light mediated photothermal/photodynamic therapy and imaging†
Received
6th October 2020
, Accepted 8th November 2020
First published on 10th November 2020
Abstract
Near infrared (NIR) light detonated phototherapy for cancer treatment based on photothermal therapy (PTT) and photodynamic therapy (PDT) has attracted increasing attention owing to its deep tissue penetration. However, the low absorption ability and therapeutic efficiency of the photosensitive drug have restricted the development of phototherapy to a great degree. Herein, a kind of IR808 dye sensitized glutathione (GSH) cladded Au–Bi bimetallic nanoparticles (Au–Bi-GSH@IR808) was prepared to enhance the inhibition effect of tumors. In this nanoplatform, the construction of GSH cladded Au–Bi bimetallic nanoparticles can effectively generate 1O2 while exhibiting outstanding photothermal conversion efficiency (η = 34.2%) upon 808 nm laser irradiation. Furthermore, IR808 as a small molecule dye endows the Au–Bi-GSH@IR808 with a higher 808 nm light absorption ability and stronger photothermal and photodynamic effects. The IR808 sensitized Au–Bi bimetallic nanoparticles with a small size (5 nm), hydrophilia and dispersible nature, exhibit a noticeably enhanced therapeutic peculiarity. Additionally, the prominent CT imaging property of Au–Bi-GSH@IR808 means it is expected to be used as a CT imaging contrast agent in clinical applications. The results of the in vitro and in vivo experiments indicate that the synthesized nanoparticles have an excellent ablation effect on cancer cells, and they are expected to be widely used in the accurate diagnosis and treatment of cancer.
Introduction
Cancer is one of the main diseases which grievously harm human health and is one of the leading causes of death all over the world.1,2 At the moment, the therapeutic effects of the commonly used clinical cancer therapy methods such as surgery, radiotherapy,3–6 ultrasonic therapies,7–9 microwave therapies10–12 and chemotherapy13–17 remain unsatisfactory. Thus, it is very important to develop new therapeutic technologies with higher cancer cell inhibition efficiency and fewer side effects.18–21 Photodynamic therapy (PDT) is a burgeoning method for cancer therapy with few side effects, minimal invasiveness, and high space-time selectivity.22–26 In order to obtain a perfect PDT effect, the three main factors of optical excitation, photosensitizers, and oxygen must be considered.27–29 The photosensitizer is the core factor, which determines the absorption efficiency of the light, the utilization of the oxygen and the productivity of the therapeutic reactive oxygen species (ROS).30–32 Unfortunately, traditional photosensitizers (PSs) such as porphyrin and phthalocyanine have low solubility in water and high toxicity to normal tissues. Besides, the excitation lights of these PSs are always located in the visible region, and they have shallow penetration depths and obvious photo-toxicity and thermal effects in bio-tissue.33,34 Compared with light with shorter wavelengths, biological tissues are more transparent to NIR photons, which are considered as a better excitation light in PDT.35 Thus, it is still urgent to develop a new photosensitive agent with properties of NIR light response, hydrophilia, low toxicity and high ROS productivity.
Among all the kinds of photosensitizer developed in the last ten years, gold nanoparticles capped with sulfhydryl organic molecules such as glutathione and captopril have been considered to be a special and promising one because of their small particle dimension, fluorescence properties, and hydrophilicity.36,37 Most importantly, for the aim of PDT applications, the prominent advantage of this material is the wide light absorption range from UV to NIR.38 The NIR light induced ROS generation ability of the nanoparticles can solve the problem of low penetration in human tissue for the high efficiency deep tissue PDT.39–41 However, comparatively speaking, NIR light absorption is relatively weaker than that in the UV and visible region, which always implies the low utilization of the NIR laser and ROS productivity.42,43 Meanwhile, some other side effects such as overheating of the surrounding bio-tissue may occur, deriving from the photons that remains unabsorbed. Thus, more research efforts are still needed to further improve the NIR light absorption of sulfhydryl capped gold nanoparticles. On the other hand, to obtain better inhibition effects to cancer cells, synergistic treatment which combines multi-mode therapeutic methods to ablate the tumor more effectively has already been conducted in clinical applications. Generally speaking, different therapeutic methods always need various trigger conditions, which may make synergistic treatment more complex. Thus, to simplify the process of the synergistic treatment and decrease the sides effects, the “all in one” method that achieve various therapeutic effects through one agent has gained more recognition.44 For the photo-induced therapy system, endowing one agent with PDT and PTT effects is also a promising method for achieving enhanced therapeutic efficiency.45,46 However, to date, research into the PDT/PTT dual-mode therapy effects of sulfhydryl capped gold nanoparticles is still limited.47
In this study, we developed GSH molecule capped gold nanoparticles with PDT/PTT dual-mode therapeutic effects upon single 808 nm light irradiation.48 The nanoparticles were further designed from the bi-basic components of the gold nucleus and GSH surface.49,50 Firstly, we introduced the bismuth element into the gold nucleus to construct Au–Bi bimetallic nanoparticles, bringing an outstanding enhancement of the photothermal conversion effect, which can combine with the inherent PDT effect of the nanoparticles for dual-mode therapy.51,52 Secondly, we further modified the GSH with IR808 molecules for a higher NIR photon capture ability.53–56 Serious experimental results indicated that the modification can obviously enhance the PDT and PTT effect simultaneously.57 The combination of PDT and PTT can achieve synergistic therapy, and the inhibitory effect of tumor cell growth is further improved compared with that of the Au-GSH sample. The higher atomic number of Bi provides great application potential for Au–Bi-GSH@IR808 as an excellent contrast agent for CT imaging because of its high X-ray attenuation effect.58,59 Especially, according to the results of the in vivo and in vitro experiments, Au–Bi-GSH@IR808 is effective in the treatment of tumors, which is attributed to the synergistic therapeutic effect of PDT and PTT in the NIR window.
Results and discussion
Fabrication and characterization
As illustrated in Scheme 1, the Au–Bi-GSH NPs were prepared by a chemical reduction process, which was triggered by rapid addition of sodium borohydride. The NIR fluorescent dye IR808 used in this work was fabricated by using the commercial dye IR-783 as the raw reagent through a nucleophilic substitution reaction. Au–Bi-GSH@IR808 NPs were constructed from Au–Bi-GSH NPs and IR808-NHS, which were mixed with 10 mL of DMF at room temperature, stirred for 24 h under N2 protection and then freeze-dried to yield Au–Bi-GSH@IR808 NPs. The micro-morphology of the as-prepared sample as well as its constitution were firstly investigated. The TEM image in Fig. 1a reveals the uniformity and dispersity of the Au–Bi-GSH@IR808 NPs, and the average size can be measured as 5 nm (Fig. S1, ESI†). Meanwhile, the HRTEM image in Fig. 1b displays the co-existing polycrystallinity in the Au–Bi-GSH@IR808 NPs. From the SAED patterns of the two selected regions, we can observe the adjacent interplanar lattices of (200) from Au and (110) from Bi with the lattice spacing of 0.203 nm and 0.227 nm, respectively. In addition, the SAED patterns of the Au–Bi-GSH@IR808 sample in Fig. 1b display the typical crystal lattice of the cubic phase of Au and the hexagonal phase of Bi, which further affirmed the co-existence of the corresponding interplanar lattices and the Au/Bi bimetallic crystals.
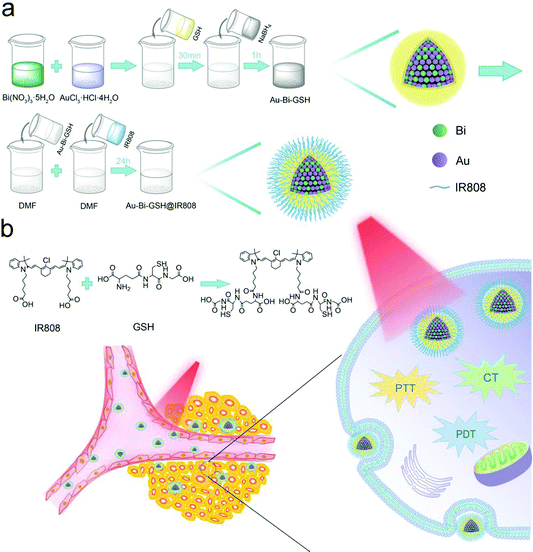 |
| Scheme 1 (a) Synthetic procedure for Au–Bi-GSH@IR808 NPs. (b) Au–Bi-GSH@IR808 NPs recognized and were assimilated by the tumor cell membrane protein under laser irradiation generated PDT/PTT dual-mode therapeutic effects to kill cancer cells. | |
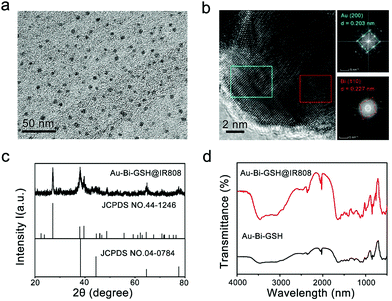 |
| Fig. 1 (a) TEM image of the Au–Bi-GSH@IR808 NPs. (b) HRTEM and SAED images of the Au–Bi-GSH@IR808 NPs. (c) Powder XRD patterns of the Au–Bi-GSH@IR808 NPs. (d) Fourier transform infrared spectroscopy (FT-IR) spectra of the Au–Bi-GSH NPs and Au–Bi-GSH@IR808. | |
Furthermore, the XRD patterns in Fig. 1c can confirm the successful synthesis of Au–Bi-GSH@IR808. In Fig. 1c, the XRD patterns of the as-fabricated Au–Bi-GSH@IR808 are presented. The standard patterns of the Bi crystal phase (JCPDS 44-1246) and Au crystal phase (JCPDS 04-0784) are also shown for comparison. It is obvious that Au–Bi-GSH@IR808 was successfully fabricated as the XRD pattern exhibits the co-existence of the representative diffraction peaks of the Bi crystal phase and the Au crystal phase. As shown, the XRD pattern of the Au–Bi-GSH@IR808 sample reveals the deviation characteristic peaks of different individual monometallic nanoparticles. This confirms the formation of bimetallic nanoparticles, designating that the Au–Bi nanocrystal is formed. Meanwhile, the surface properties of Au–Bi-GSH@IR808 were also investigated by Fourier transform infrared (FT-IR) spectroscopy, as shown in Fig. 1d. The Au–Bi-GSH NPs exhibit a stretching vibration of –SH at 2565 cm−1 (2590–2550 cm−1), and the bands for –C–S vibration at 849 cm−1 and 646 cm−1 verify the bimetallic Au–Bi-GSH NPs.51,52 Furthermore, due to the thiol-capped (GSH ligands), Au–Bi-GSH NPs can be well-dispersed in water solutions. When Au–Bi-GSH are further addressed with IR808 dye, new peaks of the sample emerge in the region of 1000–1500 cm−1. When further treated with the IR808 dye, new peaks of the sample appear in the region of 1000–1500 cm−1, designating that the IR808 dyes are successfully anchored on the Au–Bi-GSH surface through the sulfate and carboxylic groups.
The elements in the surface of Au–Bi-GSH@IR808 can be revealed through X-ray photoelectron spectroscopy (XPS), including the Au, Bi, S, C and O elements (Fig. 1a). The high resolution XPS spectra of Au 4f, Bi 4f (Fig. 2b and c) and S 2p (Fig. S2, ESI†) further elucidate the composition of the Au–Bi-GSH@IR808 NPs. The Au 4f XPS spectrum of Au–Bi-GSH@IR808 is shown in Fig. 2b, fitted to two energy sides centered at around at 88.0 and 84.2 eV. In addition, the Bi 4f spectrum (Fig. 2c) of Au–Bi-GSH@IR808 displays simple spin–orbit doublets which are mainly composed of dominant Bi3+ peaks (Bi3+ 4f5/2 at 162.4 eV and Bi3+ 4f7/2 at 158.0 eV), and there are also some asymmetries on the lower energy surface, which can be fitted to the Bi0 chemical state (Bi0 4f5/2 at 160.7 eV and Bi0 4f7/2 at 155.5 eV). These facts further indicate the formation of Au–Bi bimetallic nanoparticles. Furthermore, the good dispersity and stability of the sample is a primary prerequisite in applications of tumor therapy. The Au–Bi-GSH@IR808 is very stable in aqueous solution, which was confirmed by the zeta potentials and the digital images of the suspension of Au–Bi-GSH@IR808 (Fig. 2d). Meanwhile, the zeta potentials of IR808, Au–Bi-GSH@IR808 and GSH are −6.28 mV, −7.79 mV, and −11.4 mV, respectively. Especially, the zeta potential of Au–Bi-GSH@IR808 is closer to that of IR808, indicating that the Au–Bi-GSH has been successfully modified with the IR808 molecule. As the crucial property in the application of phototherapy, the light-absorption function of the samples was studied by the UV-vis spectrophotometer. As shown in Fig. 2e, the as-prepared Au–Bi-GSH NPs have a broad light absorption ability in the range of 350–850 nm. However, the relative absorption rate of the Au–Bi-GSH NPs in the NIR region is obviously weaker than that in the visible light region. As the IR808 absorbs photons primarily located between 650 and 850 nm with the maximum absorption peak at 808 nm (Fig. 2e), it can be used to improve the absorption ability of the Au–Bi-GSH NPs at 808 nm. Compared with the UV-vis absorption spectra of the Au–Bi-GSH NPs, the emergence of the obvious characteristic peak at 808 nm in the UV-vis absorption spectra of Au–Bi-GSH@IR808 corresponds well to that of IR808, further revealing the successful fabrication of Au–Bi-GSH@IR808. As shown in Fig. S3a (ESI†), the 808 nm NIR laser power before (P0) and after (P) passing through Au–Bi-GSH@IR808 was decreased more than that of the Au–Bi-GSH sample with the equal Bi concentration of 100 mg mL−1, which can be obviously observed from the laser beams in these solutions (Fig. S3b, ESI†). The contrast images of the same laser power (P0 = 1 W) through the Au–Bi-GSH and Au–Bi-GSH@IR808 illustrate the higher absorption of the Au–Bi-GSH@IR808 for the 808 nm laser. Digital images of the 808 nm laser (with different P0) spots after passing through the Au–Bi-GSH@IR808 (Fig. S3c, ESI†) and Au–Bi-GSH sample (Fig. S3d, ESI†) also proved that the absorption capacity of Au–Bi-GSH@IR808 is better than that of Au–Bi-GSH. Notably, it suggests that Au–Bi-GSH@IR808 could be better for use in near infrared light induced phototherapy. To further investigate the solution independent light absorption properties, the diffuse reflection spectra of the as-prepared Au–Bi-GSH@IR808 sample were obtained. As shown in Fig. 2f, a broad light absorption phenomenon in the region of UV to NIR was observed, further proving its NIR light absorption ability. The stability of the IR808 molecule modified nanoparticles was investigated, as shown in Fig. S4 (ESI†). No obvious change can be found in the UV-Vis spectra of the solution for different storage times, indicating the stable properties of the sample.
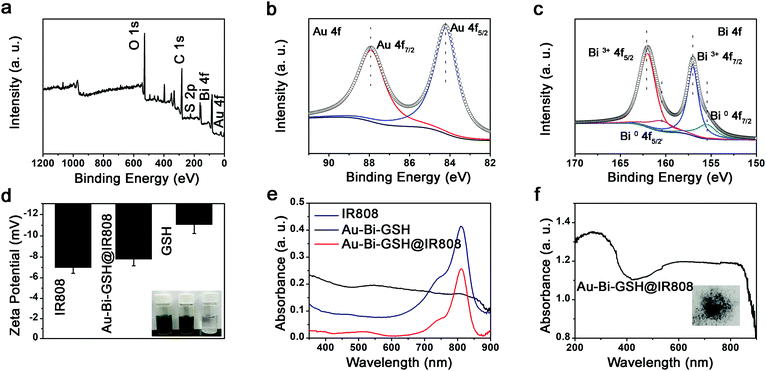 |
| Fig. 2 (a) Survey XPS spectrum of Au–Bi-GSH@IR808 NPs. (b) High resolution Au 4f spectra of Au–Bi-GSH@IR808 NPs. (c) High resolution Bi 4f spectra of Au–Bi-GSH@IR808 NPs. (d) Zeta potentials of IR808, Au–Bi-GSH@IR808, and GSH NPs. (e) UV-vis absorption spectra of IR808, Au–Bi-GSH and Au–Bi-GSH@IR808 NPs. (f) Diffuse reflection spectra of Au–Bi-GSH@IR808 NPs. | |
Next, we evaluated the photodynamic properties of the Au–Bi-GSH@IR808 sample. The ROS manufacture of the Au-GSH NPs has been well confirmed by former work. Here, DCFH-DA was used as a molecule probe to confirm the photodynamic performances of the Au–Bi-GSH@IR808. DCFH-DA can be oxidized by ROS to produce DCF with fluorescent properties, and the ROS production can be quantitatively analyzed by the optical emission spectrum of DCF.35,57Fig. 3a exhibits the emission spectrum changes of DCFH-DA solution containing Au–Bi-GSH@IR808 under 808 nm laser irradiation for different times. In Fig. 3a, it is obvious that all of the emission intensities of the DCFH-DA solutions were advanced after irradiation with the 808 nm laser, demonstrating that the Au–Bi-GSH@IR808 can produce the ROS with the 808 nm laser irradiation. With the prolongation of irradiation time of the 808 nm laser, the emission peak at 520–560 nm increases gradually, which proves that more ROS were produced. Electron paramagnetic resonance spectroscopy (EPR) equipment was used to analyze and confirm the type of ROS. In Fig. 3b, compared with the control group, the characteristic peaks of 1O2 can be observed clearly in the solutions containing the Au–Bi-GSH and Au–Bi-GSH@IR808 samples, further illustrating that the modification of the IR808 molecule will not change the type of the as-generated ROS. Besides, it is clear that the peak intensity of the Au–Bi-GSH@IR808 sample is stronger comparatively, further indicating that the modification of the IR808 molecule can enhance the 1O2 generation rate, which may bring better therapeutic effects in cancer cell inhibition applications. Before investigation into the intracellular ROS generation, the cellular uptake process of the Au–Bi-GSH@IR808 was firstly conducted through confocal laser scanning microscopy (CLSM). HeLa cells cultivated with DAPI and Au–Bi-GSH@IR808 for 0.5, 1 and 3 hours at 37 °C are shown in Fig. 3c. The Au–Bi-GSH@IR808 was tracked by its fluorescence, which is demonstrated in the overlay images. As shown, there is only a faint fluorescence in the first 0.5 h, which illustrates that few Au–Bi-GSH@IR808 nanoparticles were swallowed by the HeLa cells. With the extension of incubation time, a robust fluorescence signal appeared in the cytoplasm and nucleus, indicating that more Au–Bi-GSH@IR808 nanoparticles had entered into the cells. This result indicates that the Au–Bi-GSH@IR808 can pass through the cytomembrane and be swallowed by cancer cells in a relatively short period of time. The intracellular ROS production of the Au–Bi-GSH@IR808 sample was monitored by using non-fluorescent DCFH-DA as a ROS-index molecule probe, which passively spread through the cytomembrane and generated the non-fluorescent polar derivative DCFH by hydrolysis. DCFH can be oxidized further by the ROS to show the green fluorescence properties of (λem = 525 nm, irradiation with 488 nm light) emission dichlorofluorescein (DCF). As shown in Fig. 3d, the green fluorescence in HeLa cells incubated with Au–Bi-GSH@IR808 and irradiated with the 808 nm laser, becomes brighter with the prolongation of irradiation time, revealing the ROS generation and potential PDT effect. In a word, the as-prepared Au–Bi-GSH@IR808 with higher NIR light absorption properties can be used as a potential photosensitizer to trigger photodynamic anti-tumor treatment.
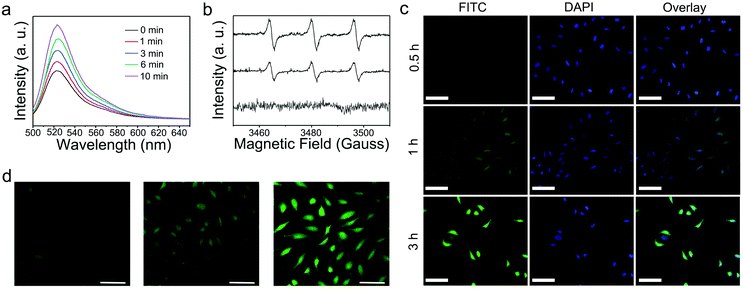 |
| Fig. 3 (a) The fluorescence spectra of DCFH-DA solution treated with Au–Bi-GSH@IR808 under 808 nm irradiation versus irradiation times. (b) The detection of the reactive oxygen species (ROS) of the Au–Bi-GSH@IR808 nanoparticles, Au–Bi-GSH and the blank control. (c) CLSM images of HeLa cells incubated with FITC labelled Au–Bi-GSH@IR808 for different times. (d) CLSM images of HeLa cells incubated with Au–Bi-GSH@IR808 for 24 h. The cells were stained with DCFH-DA and further irradiated with an 808 nm laser for different times (5, 10, and 15 min, from left to right). Scale bars for all images are 100 μm. | |
In vitro photothermal property
The thermal effect of the samples upon 808 nm laser irradiation was investigated. Firstly, the photothermal performance of the Au–Bi-GSH and Au–Bi-GSH@IR808 was given in Fig. 4a. As shown, when irradiated by 808 nm NIR laser (0.5 W cm−2), both samples presented outstanding photothermal effects upon the 808 nm irradiation. Meanwhile, the temperature of the Au–Bi-GSH@IR808 solution was enhanced by 43.3 °C, which is higher than the 36.1 °C of the Au–Bi-GSH solution, indicating a better sample. The photothermal properties of Au–Bi-GSH@IR808 with various concentrations (0, 10, 20, 50, 100, and 200 μg mL−1) under the 808 nm NIR laser (0.5 W cm−2) are shown in Fig. 4b. At the same time, infrared thermal images of different concentrations of Au–Bi-GSH@IR808 NPs and culture medium irradiated by the 808 nm near infrared laser at different time points were obtained (Fig. 4c). Meanwhile, Fig. 4d is the temperature change curve of Au–Bi-GSH@IR808 (0, 10, 20, 50, 100, 200 μg mL−1) under 808 nm laser irradiation for 600 s. The solutions increased by 1.8, 8.7, 14.6, 25.8, 38.4, and 43.3 °C, indicating that the temperature increased with the concentration of Au–Bi-GSH@IR808. The photothermal conversion efficiency of Au–Bi-GSH and Au–Bi-GSH@IR808 was measured under the same conditions by the literature method and our former work.45,46 The deionized water and the dispersion of Au–Bi-GSH@IR808 and Au–Bi-GSH were irradiated with continued 808 nm NIR laser with the same power (10 min, 0.5 W cm−2) in order to obtain the temperature equilibrium between the dispersion and the surrounding environment, and then the NIR laser was switched off. During the whole procedure (20 min), the temperature–time curves (Fig. 4e and Fig. S5a, ESI†) and the system time constant τs (Fig. 4f and Fig. S4b, ESI†), which can be obtained from the temperature curves after the NIR laser was turned off, were determined. It is obvious that the photothermal effect of Au–Bi-GSH@IR808 is better than that of Au–Bi-GSH. Thus, ηT of Au–Bi-GSH@IR808 was calculated using the following equation as:
where h represents the heat transfer coefficient; A represents the surface area of the container; ΔTmax,dis represents the maximum temperature of dispersion solution after irradiation; ΔTmax,H2O represents the maximum temperature of deionized water solution after irradiation; I represents the laser power; and A808 represents the absorbance of the sample at 808 nm. The linear time from the cooling period versus the negative natural logarithm of the driving force temperature is shown in Fig. 4f and Fig. S5b (ESI†). Based on the equation, the photothermal conversion efficiency of Au–Bi-GSH@IR808 and Au–Bi-GSH was calculated as 34.2% and 25.8%, respectively. Apparently, Au–Bi-GSH@IR808 has the higher photothermal conversion efficiency. Besides, the photothermal stability of the Au–Bi-GSH@IR808 sample was also investigated. As shown in Fig. S6 (ESI†), the cyclic photothermal performance of the Au–Bi-GSH@IR808 NPs shows that the temperature of the solution can be enhanced to as high as that of the first irradiation time after four cycles, confirming a good photothermal stability.
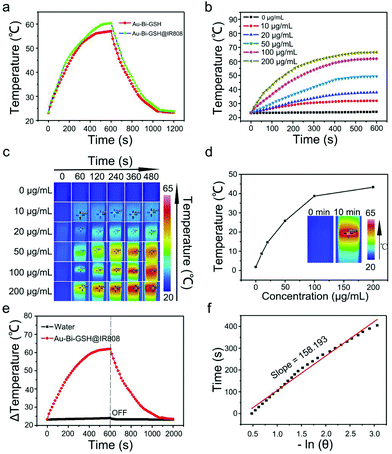 |
| Fig. 4 (a) Temperature elevation of Au–Bi-GSH and Au–Bi-GSH@IR808 upon 808 nm laser irradiation as a function of irradiation time. (b) Temperature elevation curves of culture medium and Au–Bi-GSH@IR808 dispersions suspensions at various concentrations as a function of irradiation time. (c) Infrared thermal images of different concentrations of Au–Bi-GSH@IR808 NPs and culture medium irradiated by 808 nm near infrared laser at different time points. (d) The temperature at 600 s versus concentration of the Au–Bi-GSH@IR808 NPs aqueous dispersions curve. (e) The temperature curve of the dispersion corresponds to the on/off of the laser. (f) The time data versus −ln θ curve of Au–Bi-GSH@IR808, from the cooling period of panel. | |
The biocompatibility and low toxicity of the materials are of great significance to biological applications. Before feasible application, the biocompatibility of Au–Bi-GSH@IR808 should be assessed first. The cytocompatibility and cytotoxicity of the Au–Bi-GSH@IR808 was evaluated by using L929 and HeLa cells for the correlative experimentation. Fig. 5a displays the cell viability of L929 cells cultured with the Au–Bi-GSH@IR808 sample at various concentrations for 24 h checked with 3-4,5-dimethylthiazol-2-yl-2,5-diphenyl tetrazolium bromide (MTT) assays. After 24 h incubation, the cells cultured with different concentrations all have a high activity of viability (88.2–95.1%), indicating that the sample has wonderful biocompatibility and low cytotoxicity to normal cells. Likewise, the blood compatibility has outstanding significance on the in vivo application of Au–Bi-GSH@IR808. Thus, the effect of Au–Bi-GSH@IR808 on blood was conducted simultaneously by hemolytic experiments. In Fig. 5b, Au–Bi-GSH@IR808 was dissolved in PBS at six different concentrations (25, 50, 100, 200, 400, and 800 μg mL−1), using PBS as the negative control and deionized water as the positive control. As shown in Fig. 5b, the solution of the positive control group with deionized water as the solvent is red. In contrast, the solutions of the other groups with Au–Bi-GSH@IR808 are pellucid and have a distinct red precipitation. The hemolysis rate of the supernatant of each group was further analyzed by using a UV-Vis spectrophotometer. It is found that the hemolysis efficiency of the Au–Bi-GSH@IR808 is lower than 1.03%, which indicates that the as-synthesized nanoparticles has excellent blood compatibility. To prove the anti-tumor effect of the synthesized samples, three groups of HeLa cells with diverse treatments and the corresponding cell viabilities of each groups were quantitatively checked by the MTT method, as shown in Fig. 5c. The HeLa cells viability illuminated by 808 nm NIR laser only confirms that the 808 nm NIR laser exhibits no distinct cell killing effect. Apparently, when the HeLa cells were incubated with the Au–Bi-GSH@IR808 sample and then irradiated with the 808 nm NIR light, the viability was much lower than those in the Au–Bi-GSH and 808 nm NIR laser groups. This suggests that Au–Bi-GSH@IR808 can be better used in near infrared light induced phototherapy. It also reveals that the toxicity effect of Au–Bi-GSH under the 808 nm NIR laser can be further improved by modification of the IR808 molecule. The toxicity effect of Au–Bi-GSH@IR808 under the 808 nm NIR laser is ascribed to its synergistic photothermal and photodynamic effects. A similar phenomenon can be found through the fluorescence labeling assays. The HeLa cells treated with the above three conditions were dyed with propidium iodide (PI, a red color represents the dead cells) and Calcein-AM (AM, a green color represents the viable cells). As shown in Fig. 5d, almost all of the HeLa cells illuminated by the 808 nm NIR laser are labeled by the AM, suggesting that the 808 nm NIR laser exhibits no distinct cell killing effect. In Fig. 5e, a part of the red cells labeled by PI can be seen, which validates that a large number of HeLa cells have died from the inhibition effect of Au–Bi-GSH upon NIR light. Comparatively, in Fig. 5f, more HeLa cells incubated with Au–Bi-GSH@IR808 and irradiated by the 808 nm NIR laser are labeled by PI, which is consistent with the former experimental results and further confirms that a better HeLa cell inhibition effect can be achieved.
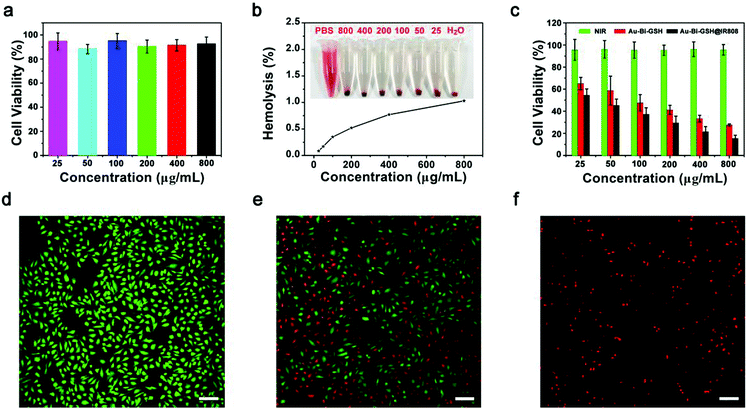 |
| Fig. 5 (a) Compatibility of L929 cells cultured with different samples. (b) A hemolytic assay of Au–Bi-GSH@IR808 by human red blood cells. (c) The intracellular toxicity of Au–Bi-GSH@IR808 (0.1 mL) at different concentrations determined by MTT assay (the cells incubated with Au–Bi-GSH@IR808 and Au–Bi-GSH were irradiated with 0.5 W cm−2 808 nm laser for 30 min). (d)–(f) CLSM images of HeLa cells after various treatments (d),–(f), the control group, Au–Bi-GSH group, and the Au–Bi-GSH@IR808 group, respectively dyed with AM and PI. All the scale bars are 100 μm. | |
In vitro and in vivo imaging performance
Due to its high resolution in the three-dimensional structure and deep penetration in bio-tissues, the CT imaging technique has been performed to analyze the sample distribution in vivo. Considering the high X-ray attenuation coefficient of the Bi element, it can be used as an effective developer to improve the signal contrast in CT imaging. Fig. 6a reveals the corresponding CT imaging performance of Au–Bi-GSH@IR808 aqueous solution with different concentrations. With the increasing sample concentration, the gray level of the images changed gradually from black shadow to white shadow, displaying a concentration dependent X-ray CT imaging effect. Au–Bi-GSH@IR808 is expected to reduce the latent side effects and complications caused by high-dose contrast agents in clinical applications. As presented in Fig. 6b, a fitting curve between the concentration of Au–Bi-GSH@IR808 and the measured CT value was obtained. Fig. 6b gives the fitting curves between the measured CT value and the concentration of Au–Bi-GSH@IR808 with the slope (22.769). The large slope implies the advantage of our as-synthesized material as contrast agents. Due to the high X-ray attenuation capability of the Au–Bi-GSH@IR808, we then researched their properties as an in vivo CT contrast agent. Fig. 6c displays the images of the mice before and after in situ injection of Au–Bi-GSH@IR808 into the axillary tumors. The mice were anesthetized by an intraperitoneal injection of 10% trichloroacetaldehyde, and then intratumorally injected with Au–Bi-GSH@IR808 into the tumors. It is obvious that the injection groups have distinct higher contrast effects. As shown in the circle, the CT imaging signal of the tumors in mice is obviously increased, and the corresponding tumors can be discovered in the three-dimensional reconstructed images. After injection with the Au–Bi-GSH@IR808 sample, the tumor site has a CT value of 128.9 Hounsfield units (HUs), which is distinctly higher than that without injection of the Au–Bi-GSH@IR808 sample (18.4 HU).
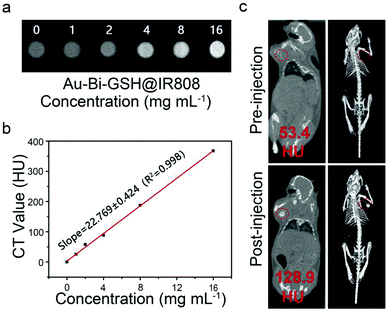 |
| Fig. 6 (a) The in vitro CT images of Au–Bi-GSH@IR808 NPs at different concentrations. (b) Concentration and HU value curves of Au–Bi-GSH@IR808 NPs solution. (c) In vivo CT images of a tumor-bearing mouse: pre- (top images) and post-injection (bottom images) at the tumor site. | |
In vivo synergetic therapy
The tumor inhibition effect of the as-synthesized samples was further studied through the in vivo experiments. First of all, ICP-MS was used to quantitatively investigate the biological distribution of Au–Bi-GSH@IR808 in mice. It is observed that Au (Fig. S7a, ESI†) and Bi (Fig. S7b, ESI†) accumulated less in the heart, kidney and tumor, but more in the spleen, lung and liver. Furthermore, along with metabolism for 24 h, Au–Bi-GSH@IR808 can be metabolized out of the body over time. Consequently, the results further validate the biological applicability of Au–Bi-GSH@IR808 as a tumor therapy agent. Then twenty-five mice were randomly separated into five groups and every group had five mice. Here, U14 tumor-implanted female mice were kept in five groups, respectively: the control group, the 808 nm laser irradiation group which was injected with saline, the mice which were intratumorally injected with Au–Bi-GSH NPs, the group which were injected with Au–Bi-GSH@IR808, and the last group which was injected with Au–Bi-GSH@IR808 and illuminated with the 808 nm NIR laser. 5 min after injection, the tumor-implanted mice of the second, fourth, fifth and sixth group were irradiated with 808 nm NIR laser for 10 min (0.5 W cm−2). The real-time temperature change during 808 nm NIR light irradiation was analyzed by an infrared thermal camera in Fig. 7a. After 10 min irradiation with an 808 nm NIR laser, the temperature of the tumor site in the mice injected with saline only increased by 3 °C, which was not high enough to harm the tumors. In contrast, the temperature of the tumor site in the mice injected with Au–Bi-GSH@IR808 was enhanced by 25 °C with the prolongation of irradiation time, which was high enough to conduct photothermal therapy (Fig. 7b). The above therapy processes were conducted repeatedly at the first, third, and 5th day and the mouse weight and tumor volume were recorded within 14 days. As shown in Fig. 7c, the Au–Bi-GSH NPs plus NIR laser group revealed medium inhibition of tumor growth in a few days. Comparatively, for the Au–Bi-GSH@IR808 plus NIR laser groups, the tumor only enhanced by a small volume, confirming the better tumor inhibition effect, which is consistent with that of the in vitro assays. The 60 days survival rate of the tumor-bearing mice in the treatment groups also indicates that the Au–Bi-GSH@IR808 + NIR group can lead to the highest tumor inhibition effect, as shown in Fig. S9 (ESI†). The digital photographs of the mice and tumors collected from the representative mice prove that the tumor size in the treatment group of Au–Bi-GSH@IR808 plus 808 nm NIR laser is the smallest, which uncovers the highest tumor inhibition efficiency (Fig. S8, ESI†). Meanwhile, the mouse weight in all of the groups maintains a steady growth tendency and no obvious weight decrease can be found in the therapeutic groups (Fig. 7d), indicating that the as-prepared nanoparticles and NIR light irradiation have minimum side effects on the mouse. To further certify the inhibition effect of Au–Bi-GSH@IR808, H&E stained sections of the tumor in Fig. 7e show the significantly increased apoptotic and necrotic tumor cells in the best inhibition group (Au–Bi-GSH@IR808 plus 808 nm NIR laser), which is due to the synergistic therapeutic effect. For the group treated by Au–Bi-GSH@IR808 plus NIR laser, the PTT effect caused by Au–Bi-GSH@IR808 in tumor sites can not only inhibit the tumor growth, but also enhance the PDT effect synergistically, for the appropriate hyperthermia may accelerate the blood flow velocity, increasing the intratumoral oxygen content and promoting tumor oxygenation. The pathomorphological analysis and the H&E stained images of the major organs: the kidney, liver, spleen, heart, and lung are shown in Fig. S10 (ESI†). It is notable that there is no obvious evident organ damage to all five of the groups, validating that the as-synthesized samples have excellent biocompatibility and low toxicity in vivo. In brief, the developed Au–Bi-GSH@IR808 can be used as a potential photosensitizer for PDT clinical applications.
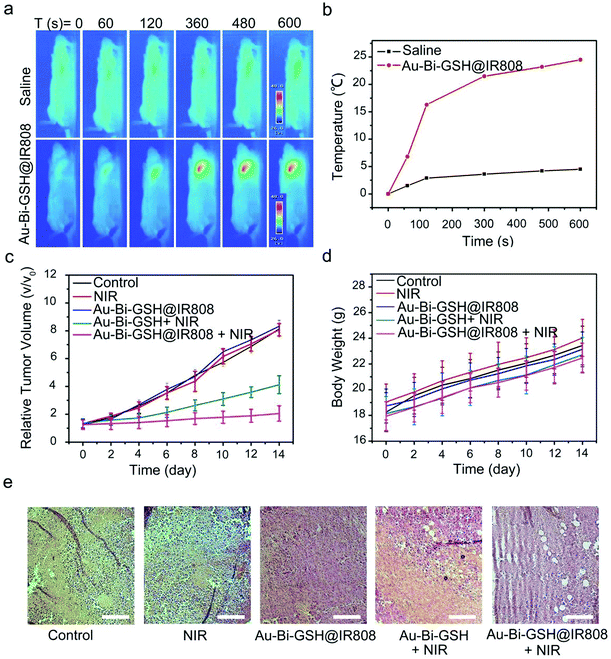 |
| Fig. 7 (a) Tumor section thermal images of tumor-bearing mice injected with saline and Au–Bi-GSH@IR808 NPs under the irradiation of an 808 nm NIR laser (0.5 W cm−2) for different times (0, 60, 120, 360, 480 and 600 s). (b) The tumor temperature change curves of mice injected with saline and Au–Bi-GSH@IR808 recorded by the infrared thermography camera. The relative (c) tumor volumes and (d) average body weight of mice after 14 days of treatment in different groups. (e) Histological H&E staining images of tumor sections after 14 days of treatment. The scale of all the images was 100 μm. | |
Experimental
Synthesis of Au–Bi-GSH
Au–Bi-GSH NPs were synthesized using the chemical reduction method published previously. Firstly, 0.10 mmol Bi(NO3)3·5H2O was dissolved in 5 mL EG, 0.10 mmol AuCl3·HCl·4H2O and 0.23 mmol TOAB were dissolved in 5.0 mL methanol, and mixed and stirred for 20 min. Thereafter, 1.0 mmol GSH was dissolved in 5 mL deionized water and with great speed added into the previous mixture under stirring for 30 min. Then, 2.0 mmol NaBH4 dissolved in 5 mL deionized water (0 °C) was with great speed added into the previous mixture under vigorous mixing and stirring for 1 h. After the reaction, the mixture is centrifuged at a low rotating speed to remove the unreacted sediments and the supernatant was washed with ethanol several times to collect the sediments and then dried under vacuum.
Synthesis of IR808
In short, 0.12 mmol of IR-783 and 80 mg of 4-mercaptobenzoic acid were dissolved in 5 ml DMF and stirred for 24 h under N2 protection at room temperature. Then, the mixture was vacuum dried at 40 °C in order to evaporate DMF. The diethyl ether was added to the product and washed several times. Following that, the mixture was filtered through a 0.45 μm polytetra-fluoroethylene (PTFE) syringe, the sediment was collected by centrifugation, and then vacuum dried to yield the IR808 dye.
After that, the IR808 was activated by EDCL and NHS to form IR808-NHS. In a nutshell, 30 mg IR808 and 19 mg NHS were dissolved in DMF (5 mL), 6 mg EDCL (dissolved in 1 mL DMF) was added to the mixture at room temperature and then stirred for 2 h under N2 protection. Finally, the mixture was vacuum distilled and evaporated to obtain the IR808-NHS.
Synthesis of Au–Bi-GSH@IR808
Au–Bi-GSH NPs and IR808-NHS were mixed with 10 mL of DMF at room temperature and then stirred for 24 h under N2 protection and then freeze-dried to yield Au–Bi-GSH@IR808 NPs.
Characterization
X-ray powder diffraction (XRD) patterns were obtained with a Rigaku D/max-TTR-III diffractometer using CuKα radiation (λ = 0.15405 nm) in the range of 20° to 80° with a scanning rate of 15° min−1. Transmission electron microscopy (TEM) and high-resolution TEM (HRTEM) micrographs were obtained from a FEI Tecnai G2 S-Twin transmission electron microscope equipped with a field emission gun operating at 200 kV. The UCL emission spectra were obtained from the Edinburgh FLS980 equipment. Fourier-transform infrared (FT-IR) spectra were obtained on an AVATAR 360 FT-IR spectrophotometer. UV-vis absorption spectra were detected by the UV-1601 UV-vis spectrophotometer. Zeta potentials were measured on zetasizer equipment. X-ray photoelectron spectroscopy measured the composition of the substance by using A VG ESCALAB MK II electron energy spectrometer (Mg KR, 1253.6 eV). All tests were implemented at room temperature.
In vitro viability of Au–Bi-GSH@IR808
To determine the viability of Au–Bi-GSH@IR808 with L929 cells, the cells were seeded in a 96-well plate (200 μL, per well) and incubated for 24 h (5% CO2, 37 °C). Au–Bi-GSH@IR808 was diluted into different concentrations (25, 50, 100, 200, 400 and 800 μg mL−1) and then put into 96-well plates and incubated for another 24 h (5% CO2, 37 °C). Thereafter, 20 μL of MTT solution (5 mg mL−1) was put into each well and continued to be incubated for another 4 h (5% CO2, 37 °C). Then, 150 μL of DMSO was added into each well. Finally, the absorbance at 490 nm was recorded by using a microplate reader for calculation.
In vitro cytotoxicity of Au–Bi-GSH@IR808
To analyze the cytotoxicity of Au–Bi-GSH@IR808 with HeLa cells, the cells were planted in a 96-well plate (200 μL, per well) and then cultured for 24 h in 5% CO2 at 37 °C. Thereafter, the Au–Bi-GSH and Au–Bi-GSH@IR808 were dissolved in culture and diluted to different concentrations (25, 50, 100, 200, 400 and 800 μg mL−1), and then the cells were treated with the following treatments: NIR irradiation only and Au–Bi-GSH and Au–Bi-GSH@IR808 NIR irradiation, respectively. After 4 h of incubation, the redundant nanoparticles were removed by washing with PBS three times. The cells were exposed to an 808 nm NIR laser (0.5 W cm−2) and cultured in the dark for 48 h. Subsequently, 20 μL of the MTT solution (5 mg mL−1) was put into each well and continued to be incubated for another 4 h (5% CO2, 37 °C). Next, 150 μL of DMSO was added to the wells, and the absorbance at 490 nm was detected using a microplate reader for calculation. Finally, the HeLa cells were dyed with propidium iodide (PI) and Calcein AM to distinguish dead and live cells.
In vivo toxicity
All the female Kunming mice were purchased from Second Affiliated Hospital, Harbin Medical University. All the animal experiments were conducted in compliance with the specifications of The National Regulation of China for Care and Use of Laboratory Animals and approved by Harbin Medical University (SYDW 2019-82). The U14 cells were injected subcutaneously under the left axilla of each mouse to induce tumor formation. When the average tumors grew to 6–8 mm, the mice were randomly divided into five groups (n = 5 per group). The first two groups of the mice were injected with saline and set as the control and NIR groups, respectively. The rest of the three groups of mice were intravenously injected with Au–Bi-GSH@IR808 (group three), Au–Bi-GSH (group four), and Au–Bi-GSH@IR808 (group five). After 24 h, the tumor of the mice was irradiated with an 808 nm NIR laser for 10 min (0.72 W cm−2, with a 5 min break after 5 min illumination, groups 2, 3, and 5). All the groups of mice underwent second samples injections (7 days after the first injection) and treatment. The body weights and the tumor sizes were measured and recorded every 2 days after treatment, up to 14 days, and estimated as volume V = (width2 × length)/2. The relative tumor volume was calculated as V/V0 (V0 is the corresponding tumor volume before treatment).
Conclusions
In summary, a kind of IR808 dye sensitized nanoparticles with GSH cladded bimetallic nanoparticles (Au–Bi-GSH@IR808) was successfully constructed for X-ray CT imaging guided PTT/PDT dual mode cancer therapy. The as-fabricated Au–Bi-GSH@IR808 has a strong NIR absorbance, excellent photothermal conversion efficiency (η = 34.2%), and high ROS productivity upon single 808 nm light excitation for the ablation of tumor cells in vitro and in vivo. Furthermore, IR808 enhances the absorption ability of Au–Bi-GSH@IR808 at 808 nm, which effectively solves the problem of the low absorption value of Au–Bi NPs at the NIR region, and makes a high utilization of NIR light to produce synergistic PDT and PTT antitumor effects. The multifunctional Au–Bi-GSH@IR808 nanocomposite which combined photothermal imaging and CT has great potential in cancer treatment.
Conflicts of interest
There are no conflicts to declare.
Acknowledgements
Financial support from the National Natural Science Foundation of China (NSFC 51972075, 51972076, 51902066 and 51602073), Outstanding Youth Fund of Heilongjiang Province (YQ2019E016), Natural Science Foundation of Shandong Province (ZR2019ZD29), Open Funds of the State Key Laboratory of Rare Earth Resource Utilization (RERU2020002) and the Fundamental Research Funds for the Central Universities is greatly acknowledged.
References
- J. Massague and A. C. Obenauf, Nature, 2016, 529, 298–306 CrossRef CAS.
- J. Wang, J. Sun, W. Hu, Y. Wang, T. Chou, B. Zhang, Q. Zhang, L. Ren and H. Wang, Adv. Mater., 2020, 32, 2001862 CrossRef CAS.
- N. Lu, W. P. Fan, X. Yi, S. Wang, Z. T. Wang, R. Tian, O. Jacobson, Y. J. Liu, B. C. Yung, G. F. Zhang, Z. G. Teng, K. Yang, M. M. Zhang, G. Niu, G. M. Lu and X. Y. Chen, ACS Nano, 2018, 12, 1580–1591 CrossRef CAS.
- X. J. Yu, A. Li, C. Z. Zhao, K. Yang, X. Y. Chen and W. W. Li, ACS Nano, 2017, 11, 3990–4001 CrossRef CAS.
- G. S. Song, Y. Y. Chen, C. Liang, X. Yi, J. J. Liu, X. Q. Sun, S. D. Shen, K. Yang and Z. Liu, Adv. Mater., 2016, 28, 7143–7148 CrossRef CAS.
- C. Zhang, K. L. Zhao, W. B. Bu, D. L. Ni, Y. Y. Liu, J. W. Feng and J. L. Shi, Angew. Chem., Int. Ed., 2015, 54, 1770–1774 CrossRef CAS.
- L. Cheng, J. J. Liu, X. Gu, H. Gong, X. Z. Shi, T. Liu, C. Wang, X. Y. Wang, G. Liu, H. Y. Xing, W. B. Bu, B. Q. Sun and Z. Liu, Adv. Mater., 2014, 26, 1886–1893 CrossRef CAS.
- Y. J. Liu, J. He, K. K. Yang, C. L. Yi, Y. Liu, L. M. Nie, N. M. Khashab, X. Y. Chen and Z. H. Nie, Angew. Chem., Int. Ed., 2015, 54, 15809–15812 CrossRef CAS.
- S. Gao, G. H. Wang, Z. N. Qin, X. Y. Wang, G. Q. Zhao, Q. J. Ma and L. Zhu, Biomaterials, 2017, 112, 324–335 CrossRef CAS.
- H. T. Shi, T. L. Liu, C. H. Fu, L. L. Li, L. F. Tan, J. Z. Wang, X. L. Ren, J. Ren, J. X. Wang and X. W. Meng, Biomaterials, 2015, 44, 91–102 CrossRef CAS.
- H. T. Shi, M. Niu, L. F. Tan, T. L. Liu, H. B. Shao, C. H. Fu, X. L. Ren, T. C. Ma, J. Ren, L. L. Li, H. Y. Liu, K. Xu, J. X. Wang, F. Q. Tang and X. W. Meng, Chem. Sci., 2015, 6, 5016–5026 RSC.
- J. Wu, G. R. Williams, S. Niu, Y. Yang, Y. Li, X. Zhang and L.-M. Zhu, Theranostics, 2020, 10, 841–855 CrossRef CAS.
- Y. Y. Zhang, C. Teh, M. H. Li, C. Y. Ang, S. Y. Tan, Q. Y. Qu, V. Korzh and Y. L. Zhao, Chem. Mater., 2016, 28, 7039–7050 CrossRef CAS.
- M. H. Chan, S. P. Chen, C. W. Chen, Y. C. Chan, R. J. Lin, D. P. Tsai, M. Hsiao, R. J. Chung, X. Y. Chen and R. S. Liu, J. Phys. Chem. C, 2018, 122, 2402–2412 CrossRef CAS.
- M. Li, Y. J. Guan, A. D. Zhao, J. S. Ren and X. G. Qu, Theranostics, 2017, 7, 2996–3006 CrossRef CAS.
- X. Ding, J. H. Liu, J. Q. Li, F. Wang, Y. H. Wang, S. Y. Song and H. J. Zhang, Chem. Sci., 2016, 7, 6695–6700 RSC.
- M. Lyu, D. Zhu, Y. Duo, Y. Li and H. Quan, Biomaterials, 2020, 233, 119656 CrossRef.
- S. H. Zhang, C. X. Sun, J. F. Zeng, Q. Sun, G. L. Wang, Y. Wang, Y. Wu, S. X. Dou, M. Y. Gao and Z. Li, Adv. Mater., 2016, 28, 8927–8936 CrossRef CAS.
- Y. Deng, X. Tian, S. Lu, M. Xie, H. Hu, R. Zhang, F. Lv, L. Cheng, H. Gu, Y. Zhao and Y. Pan, ACS Appl. Mater. Interfaces, 2018, 10, 31106–31113 CrossRef CAS.
- C. X. Yue, P. Liu, M. B. Zheng, P. F. Zhao, Y. Q. Wang, Y. F. Ma and L. T. Cai, Biomaterials, 2013, 34, 6853–6861 CrossRef CAS.
- H. Wang, Z. Liu, S. Wang, C. Dong, X. Gong, P. Zhao and J. Chang, ACS Appl. Mater. Interfaces, 2014, 6, 3219–3225 CrossRef CAS.
- B. Liu, C. X. Li, Z. Y. Cheng, Z. Y. Hou, S. S. Huang and J. Lin, Biomater. Sci., 2016, 4, 890–909 RSC.
- D. Lin, T. Qin, Y. Wang, X. Sun and L. Chen, ACS Appl. Mater. Interfaces, 2014, 6, 1320–1329 CrossRef CAS.
- Z. X. Zhao, S. G. Shi, Y. Z. Huang, S. H. Tang and X. L. Chen, ACS Appl. Mater. Interfaces, 2014, 6, 8878–8885 CrossRef CAS.
- S. Luo, Z. Yang, X. Tan, Y. Wang, Y. Zeng, Y. Wang, C. Li, R. Li and C. Shi, ACS Appl. Mater. Interfaces, 2016, 8, 17176–17186 CrossRef CAS.
- N. M. Idris, M. K. Gnanasammandhan, J. Zhang, P. C. Ho, R. Mahendran and Y. Zhang, Nat. Med., 2012, 18, 1580–1585 CrossRef CAS.
- Y. Y. Liu, Y. Liu, W. B. Bu, C. Cheng, C. J. Zuo, Q. F. Xiao, Y. Sun, D. L. Ni, C. Zhang, J. A. Liu and J. L. Shi, Angew. Chem., Int. Ed., 2015, 54, 8105–8109 CrossRef CAS.
- Z. Z. Yu, W. Pan, N. Li and B. Tang, Chem. Sci., 2016, 7, 4237–4244 RSC.
- W. P. Fan, P. Huang and X. Y. Chen, Chem. Soc. Rev., 2016, 45, 6488–6519 RSC.
- Y. Huang, Q. Liu, Y. Wang, N. He, R. Zhao, J. Choo and L. Chen, Nanoscale, 2019, 11, 12220–12229 RSC.
- X. W. Liu, R. R. Deng, Y. H. Zhang, Y. Wang, H. J. Chang, L. Huang and X. G. Liu, Chem. Soc. Rev., 2015, 44, 1479–1508 RSC.
- X. G. Liu, C. H. Yan and J. A. Capobianco, Chem. Soc. Rev., 2015, 44, 1299–1301 RSC.
- D. H. Hu, Z. H. Sheng, G. H. Gao, F. M. Siu, C. B. Liu, Q. Wan, P. Gong, H. R. Zheng, Y. F. Ma and L. T. Cai, Biomaterials, 2016, 93, 10–19 CrossRef CAS.
- W. Zhang, Y. Wang, X. Sun, W. Wang and L. Chen, Nanoscale, 2014, 6, 14514–14522 RSC.
- M. J. Jiang, R. T. K. Kwok, X. S. Li, C. Gui, J. W. Y. Lam, J. A. Qu and B. Z. Tang, J. Mater. Chem. B, 2018, 6, 2557–2565 RSC.
- R. R. Nasaruddin, T. K. Chen, N. Yan and J. P. Xie, Coord. Chem. Rev., 2018, 368, 60–79 CrossRef CAS.
- Y. Su, T. T. Xue, Y. X. Liu, J. X. Qi, R. C. Jin and Z. K. Lin, Nano Res., 2019, 12, 1251–1265 CrossRef CAS.
- W. W. Wang, C. L. Hao, M. Z. Sun, L. G. Xu, C. L. Xu and H. Kuang, Adv. Funct. Mater., 2018, 28, 1800310 CrossRef.
- Z. Y. Hou, K. R. Deng, C. X. Li, X. R. Deng, H. Z. Lian, Z. Y. Cheng, D. Y. Jin and J. Lin, Biomaterials, 2016, 101, 32–46 CrossRef CAS.
- Y. Huang, N. He, Y. Wang, D. Shen, Q. Kang, R. Zhao and L. Chen, J. Mater. Chem. B, 2019, 7, 1149–1159 RSC.
- X. M. Li, Z. Z. Guo, T. C. Zhao, Y. Lu, L. Zhou, D. Y. Zhao and F. Zhang, Angew. Chem., Int. Ed., 2016, 55, 2464–2469 CrossRef CAS.
- F. Lu, L. Yang, Y. J. Ding and J. J. Zhu, Adv. Funct. Mater., 2016, 26, 4778–4785 CrossRef CAS.
- H. Cheng, J. Y. Zhu, S. Y. Li, J. Y. Zeng, Q. Lei, K. W. Chen, C. Zhang and X. Z. Zhang, Adv. Funct. Mater., 2016, 26, 7847–7860 CrossRef CAS.
- T. Liu, C. Wang, W. Cui, H. Gong, C. Liang, X. Z. Shi, Z. W. Li, B. Q. Sun and Z. Liu, Nanoscale, 2014, 6, 11219–11225 RSC.
- K. R. Deng, Z. Y. Hou, X. R. Deng, P. P. Yang, C. X. Li and J. Lin, Adv. Funct. Mater., 2015, 25, 7280–7290 CrossRef CAS.
- R. C. Lv, C. N. Zhong, R. M. Li, P. P. Yang, F. He, S. L. Gai, Z. Y. Hou, G. X. Yang and J. Lin, Chem. Mater., 2015, 27, 1751–1763 CrossRef CAS.
- H. Z. Chen, X. W. Zeng, H. P. Tham, S. Z. F. Phua, W. Cheng, W. F. Zeng, H. R. Shi, L. Mei and Y. L. Zhao, Angew. Chem., Int. Ed., 2019, 58, 7641–7646 CrossRef CAS.
- T. Yang, Y. A. Tang, L. Liu, X. Y. Lv, Q. L. Wang, H. T. Ke, Y. B. Deng, H. Yang, X. L. Yang, G. Liu, Y. L. Zhao and H. B. Chen, ACS Nano, 2017, 11, 1848–1857 CrossRef CAS.
- M. W. Ji, M. Xu, W. Zhang, Z. Z. Yang, L. Huang, J. J. Liu, Y. Zhang, L. Gu, Y. X. Yu, W. C. Hao, P. F. An, L. R. Zheng, H. S. Zhu and J. T. Zhang, Adv. Mater., 2016, 28, 3094–3101 CrossRef CAS.
- R. C. Lv, P. P. Yang, B. Hu, J. T. Xu, W. T. Shang and J. Tian, ACS Nano, 2017, 11, 1064–1072 CrossRef CAS.
- R. Mohan, Nat. Chem., 2010, 2, 336 CrossRef CAS.
- D. Lee, S. H. Jeong and E. Kang, J. Ind. Eng. Chem., 2018, 65, 205–212 CrossRef CAS.
- G. Y. Chen, J. Damasco, H. L. Qiu, W. Shao, T. Y. Ohulchanskyy, R. R. Valiev, X. Wu, G. Han, Y. Wang, C. H. Yang, H. Agren and P. N. Prasad, Nano Lett., 2015, 15, 7400–7407 CrossRef CAS.
- G. Y. Chen, W. Shao, R. R. Valiev, T. Y. Ohulchanskyy, G. S. He, H. Agren and P. N. Prasad, Adv. Opt. Mater., 2016, 4, 1760–1766 CrossRef CAS.
- W. Q. Zou, C. Visser, J. A. Maduro, M. S. Pshenichnikov and J. C. Hummelen, Nat. Photonics, 2012, 6, 560–564 CrossRef CAS.
- X. Wu, H. Lee, O. Bilsel, Y. W. Zhang, Z. J. Li, T. Chen, Y. Liu, C. Y. Duan, J. Shen, A. Punjabi and G. Han, Nanoscale, 2015, 7, 18424–18428 RSC.
- Q. Y. Shao, X. S. Li, P. Y. Hua, G. T. Zhang, Y. Dong and J. Q. Jiang, J. Colloid Interface Sci., 2017, 486, 121–127 CrossRef CAS.
- B. X. Wei, X. J. Zhang, C. Zhang, Y. Jiang, Y. Y. Fu, C. S. Yu, S. K. Sun and X. P. Yan, ACS Appl. Mater. Interfaces, 2016, 8, 12720–12726 CrossRef CAS.
- P. P. Lei, P. Zhang, Q. H. Yuan, Z. Wang, L. L. Dong, S. Y. Song, X. Xu, X. L. Liu, J. Feng and H. J. Zhang, ACS Appl. Mater. Interfaces, 2015, 7, 26346–26354 CrossRef CAS.
Footnote |
† Electronic supplementary information (ESI) available. See DOI: 10.1039/d0tb02378g |
|
This journal is © The Royal Society of Chemistry 2021 |