Coassembly of nucleus-targeting gold nanoclusters with CRISPR/Cas9 for simultaneous bioimaging and therapeutic genome editing†
Received
10th August 2020
, Accepted 11th November 2020
First published on 12th November 2020
Abstract
The clustered regularly interspaced short palindromic repeats (CRISPR)/associated protein 9 (CRISPR/Cas9) technology enables genome editing with high precision and versatility and has been widely utilized to combat viruses, bacteria, cancers, and genetic diseases. Nonviral nanocarriers can overcome several limitations of viral vehicles, including immunogenicity, inflammation, carcinogenicity, and low versatility, and thus represent promising platforms for CRISPR/Cas9 delivery. Herein, we for the first time develop the application of protamine-capped gold nanoclusters (protamine–AuNCs) as an effective nanocarrier for Cas9–sgRNA plasmid transport and release to achieve efficient genome editing. The protamine–AuNCs integrate the merits of AuNCs and protamine: AuNCs are able to promptly assemble with Cas9–sgRNA plasmids to allow efficient cellular delivery, while the cationic protamine facilitates the effective release of Cas9–sgRNA plasmids into the cellular nucleus. The AuNCs/Cas9–gRNA plasmid nanocomplexes can not only achieve successful gene editing in cells but also knock out the oncogenic gene for cancer therapy. Moreover, the AuNCs with excellent photoluminescence characteristics endow our nanoplatform with the functionality of bioimaging. Overall, our study provides strong evidence that demonstrates protamine–AuNCs as an effective CRISPR/Cas9 delivery tool for gene therapy.
Introduction
The bacterially-derived CRISPR/Cas9 (clustered regularly interspaced short palindromic repeats (CRISPR)/associated protein 9) genome editing technology is directed by an engineered single-guide RNA (sgRNA) for recognizing the target genomic locus to introduce DNA double-strand breaks. The repair of the breaks through precise homology-directed repair or nonhomologous end-joining with high specificity1–5 leads to the completion of gene editing. CRISPR/Cas9 offers a versatile and powerful toolbox for gene editing applications in broad fields crossing agriculture, biotechnology, regenerative medicine, and pharmacology.6–8 Various CRISPR/Cas9 formulations have been developed for gene editing, including the plasmid encoding Cas9/sgRNA sequence, Cas9 mRNA, and a separate sgRNA, as well as ribonucleoprotein consisting of Cas9 endonuclease and sgRNA complexes.9–11 Owing to the disadvantages of tedious synthesis procedures and instabilities of the Cas9 mRNA and protein, plasmids encoding Cas9 and sgRNA are more convenient choices in terms of practical genome editing bioapplications.12,13 Nevertheless, great challenges remain for the effective, targeted and safe transportation of CRISPR/Cas9 plasmids into cells or tissues due to the large gene size (frequently exceeds 10 Kb).13,14 Although viral vectors exhibit good efficiency of gene transfection, they may provoke mutagenesis, carcinogenesis, and immunogenicity complications.15–18 As an important alternative, nonviral delivery vehicles, such as multifunctional polymers,19–24 lipid nanoparticles,13,25–27 exosomes,28,29 and gold nanoparticles,13,30 could help deliver Cas9–sgRNA plasmids for intracellular gene editing. However, the unsatisfactory delivery efficiency or/and the potential cytotoxicity of the nonviral vehicles often limits gene regulation efficacy.31–34 Consequently, the fabrication of highly effective and safe gene carriers to realize the delivery of Cas9–sgRNA plasmids is still highly desirable.13,17,35,36
Gold nanoclusters (AuNCs) with a core size approaching the Fermi wavelength of conduction electrons (typically less than 2 nm) consist of a few to hundreds of gold atoms.37,38 Due to their excellent biocompatibility, chemical inertness, strong fluorescence emission, and tunable surface functionalization, AuNCs have attracted special interest in the field of bioimaging and biolabeling.39–41 Recent investigations have explored a growing number of AuNC nanocomposites for disease diagnostic and therapeutic applications, including CRISPR/Cas9 system delivery.17,42,43 For example, Jiang's group prepared nanocarriers with AuNC cores and lipid shells, which could efficiently deliver Cas9 proteins and sgRNA plasmids for melanoma therapy.17 Ju et al. reported nanohybrids of Cas9 proteins and AuNCs for efficient inactivation of virus oncogenes.9 Nevertheless, to the best of our knowledge, no reports have explored AuNC nanocomponents as nanocarriers to deliver Cas9–sgRNA plasmids effectively for genome editing.
Herein, we develop a nanoplatform for Cas9–sgRNA plasmid delivery based on protamine-functionalized AuNCs for effective genome editing (Scheme 1). Protamines are highly cationic nuclear proteins with rich arginine residues (up to 67%), and they are natural chromatin components in sperm.44–47 The cationic protamines are well known to densely package anionic DNA molecules to assist in forming a compact structure during spermatogenesis and deliver DNA to the egg nucleus following fertilization.48,49 Therefore, protamines with cell-penetrating properties and nucleus-targeting abilities have great potential for effective gene delivery into cells.50,51 In addition, previous research indicated that the attachment of protamines to gold nanomaterials could significantly enhance the resistance of the protamines to protease degradation.52 These characteristics of protamines, combined with their good water solubility and excellent natural biocompatibility, make them ideal candidates for synthesis of protamine-templated AuNCs with highly positive charges for efficient Cas9/sgRNA plasmid delivery.44,51,53 In this study, we find that the synthesized protamine–AuNCs can efficiently complex with Cas9/sgRNA plasmids and deliver the plasmids into target cells, which can effectively knock out the target HPV gene, making protamine–AuNCs attractive candidates for gene therapy. Moreover, the protamine–AuNCs with excellent cell nucleus-targeting ability and photoluminescence characteristics endow our nanoplatform with the functionality of bioimaging. To the best of our knowledge, this is the first example of applying protamine-templated AuNCs as gene nanocarriers to deliver Cas9–sgRNA plasmids. The proposed work can help to expand the novel applications of AuNCs in biomedicine and biotechnology.
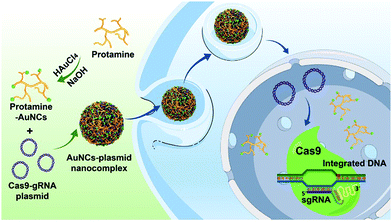 |
| Scheme 1 Schematic representation of the preparation of protamine–AuNCs and assembly of the AuNCs/plasmid nanocomplex for gene editing. | |
Experimental
Materials and measurements
All chemicals, including thiazolyl blue tetrazolium bromide (MTT), protamine, and HAuCl4·4H2O, were obtained from Sigma-Aldrich unless otherwise specified and were used as received. DNA oligonucleotides were purchased from Integrated DNA Technologies Inc. All reagents were of analytical reagent grade and utilized as received. All samples were prepared using ultrapure water (18.2 MΩ; Millipore Co.).
Transmission electron microscopy (TEM) images were captured with a FEI Titan S/TEM system at 200 kV. The size of the protamine–AuNCs was analyzed by Nano Measurer 1.2 software using the TEM images. Scanning electron microscopy (SEM) images were acquired using a Hitachi S-4700 Instrument. Particle sizes and zeta potentials were determined by a ZetaSizer NanoZS-90 (Malvern Instruments). Fluorescence measurements were carried out by using a GeminiXPS microplate spectrofluorometer. Absorbance measurements were measured with a Spectramax Plus 384 microplate reader. Flow cytometry data were collected using an LSRII (BD) and analyzed with FlowJo. An A1RMP confocal microscope (Nikon Instruments) was used for all confocal imaging.
Preparation of protamine–AuNCs
Protamine–AuNCs were synthesized by a one-step process.43,44,54,55 Firstly, HAuCl4 (25.0 mL, 10.0 mM) was added into protamine (25.0 mL, 2.5 mg mL−1). The mixture was subsequently stirred at 37 °C for 2 min. Then, NaOH (2.5 mL, 1.0 M) was added into the mixture. The reaction was then conducted at 37 °C for 12 h with strong stirring. The solution color gradually varied from light yellow to yellowish-brown. The prepared AuNCs were stored at 4 °C in the dark.
Fabrication of protamine–AuNCs/Cas9–gRNA plasmid nanocomplexes
The protamine–AuNCs/Cas9–gRNA plasmid nanocomplexes were prepared by self-assembly through electrostatic interactions. The nanocomplexes were prepared at protamine–AuNCs
:
Cas9–gRNA plasmid ratios (w/w) of 0, 0.2, 0.5, 0.8, 1, 2, 5, 10, 25, 50, and 100. The Cas9–gRNA plasmids were added dropwise into the protamine–AuNC solution under continuous stirring. After that, the mixture was aged for 30 min at room temperature. The obtained protamine–AuNCs/Cas9–gRNA plasmid nanocomplexes were stored at 4 °C in the dark for further studies.
Cellular imaging
Cells were seeded overnight in glass microscopy dishes (50
000 cells) at 37 °C. After that, the cells were incubated with the protamine–AuNCs/plasmid nanocomplexes and control groups for 4 h respectively. The plasmids were labeled with FITC. Then, the cells were washed three times with PBS to remove the unbound nanocomplexes. Subsequently, cellular imaging was carried out using an A1RMP confocal microscope.
Gene disruption assay
Cells were cultured overnight in a 24-well plate (50
000 cells per well). The media were then replaced with Opti-MEM medium (0.5 mL) containing the protamine–AuNCs/Cas9–gRNA plasmid nanocomplexes. After treatment for 4 h, the medium was changed to full serum medium and the cells were further incubated for 48 h. The cells were analyzed using a confocal microscope and quantified by flow cytometry.
T7 endonuclease 1 (T7E1) assay
Genomic DNA was isolated utilizing a QIAamp DNA Mini Kit following the manufacturer's instructions. The genomic target region encoding E7 gene was prepared by applying a PCR amplicon. Afterward, the PCR products were purified, annealed and treated with T7E1. Finally, the digested DNA was analyzed utilizing polyacrylamide gel electrophoresis to determine the indel efficiency.
Cell proliferation assays
The cells were cultured at a density of 10
000 cells per well in 96-well plates and incubated for 24 h. The protamine–AuNCs/Cas9–gRNA plasmid nanocomplexes and control groups were then incubated with the cells for 48 h. After that, the cell proliferations were evaluated by applying the MTT assay. Briefly, MTT was directly added to each well for 4 h incubation at 37 °C. After the addition of DMSO, the absorbances were determined at a wavelength of 570 nm.
Western blot assay
Cells were collected and lysed with RIPA buffer. Protein extracts were then separated by SDS–PAGE and electrophoretically transferred to nitrocellulose membranes. After that, the membranes were treated with primary antibodies against HPV18 E7 (Santa Cruz, #SC-365035, 1
:
500) and β-actin (Santa Cruz, #SC-47778, 1
:
500). HRP-conjugated anti-mouse secondary antibodies (1
:
5000) were further applied and signals were detected using an ECL system.
Results and discussion
Construction and characterization of protamine–AuNCs
The protamine templated-AuNCs were prepared according to a previous method with slight modifications.43,44,54,55 The fluorescence and absorbance properties of the protamine templated-AuNCs were firstly monitored. As illustrated in Fig. 1a, protamine–AuNCs revealed remarkably strong fluorescence with a maximum emission wavelength at 600 nm (Fig. S1, ESI†), which was observed to remain stable at 4 °C for at least 6 months (data not shown). Moreover, no plasmon peak at 520 nm was detected, suggesting that there was no large Au nanoparticle formation.9 In addition, the AuNCs exhibited significant positive effects on the biological stability of the protamine (Fig. S2, ESI†). Next, the successful construction of protamine–AuNCs was also verified using transmission electron microscopy (TEM) measurement. The TEM imaging (Fig. 1b) and the homologous size distribution histogram (Fig. S3, ESI†) showed that AuNCs exhibited good dispersity and a homogeneous size distribution, with an average size of about 1.56 nm. The average hydration diameter obtained by the dynamic light scattering (DLS) experiment was about 3.5 nm (Fig. 1c). This slightly larger size as compared to the diameter obtained from TEM measurement could be attributed to the hydration layers of protein-laced surfaces of the gold nanoclusters in the solution.56 The zeta potential measurement confirmed the highly positive charge of protamine–AuNCs (Fig. 1c), which would facilitate ionic self-assembly of ultrasmall AuNCs and negatively charged DNA. Moreover, the energy-dispersive X-ray spectroscopy (EDS) spectrum demonstrated the primary Au signals, further implying the successful construction of the AuNCs (Fig. 1d).
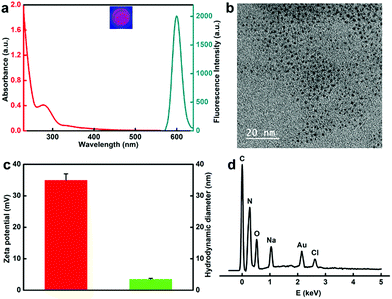 |
| Fig. 1 (a) The optical absorption spectrum and emission fluorescence spectrum of protamine–AuNCs. (b) TEM image of protamine–AuNCs. Scale bar: 20 nm. (c) The zeta potential (red) and the hydrodynamic diameter (green) of protamine–AuNCs. (d) EDS spectrum of the as-synthesized protamine–AuNCs. | |
Preparation and characterization of the AuNCs/pDNA nanocomplexes
In the following, we explored whether the protamine–AuNCs could serve as scaffolds for efficient Cas9–gRNA plasmid DNA (pDNA) delivery. Firstly, the capacity of protamine–AuNCs to condense pDNA at distinct ratios was monitored by agarose gel electrophoresis and zeta potential characterization. Fig. 2a shows that no free pDNA band could be observed with weight ratios of AuNCs/pDNA above 1, revealing that the protamine–AuNCs could effectively package pDNA due to electrostatic interactions, which is an important precondition for effective gene transfection.48 After that, the surface charge of the AuNCs/pDNA assembly was investigated, as shown in Fig. 2b, which exhibited a monotonically increasing trend along with the raising weight ratio from 0.2 to 10 and a plateau of about 35 mV. Therefore, the AuNCs/pDNA complex with the weight ratio of 10 was chosen in the following experiments. The highly positively charged AuNCs/pDNA complexes would enhance the ability of pDNA to get access into cells. Following surface charge characterization, the morphology of the prepared AuNCs/pDNA complexes was then determined by using scanning electron microscopy (SEM), as can be seen in Fig. 2c. The SEM result showed that pDNA and AuNCs assembled into spheroid and compact nanosized particles with an average diameter of about 100 nm. Moreover, the AuNCs/pDNA particles remained stable over 7 days (Fig. S4, ESI†). The strong pDNA compaction capability of protamine–AuNCs was attributed to the positive charge of protamine and the rigid core of the AuNCs, which could help maintain the spherical structural integrity and greatly improve the cellular uptake and gene delivery.57,58 We then evaluated the cytotoxicities of protamine, AuNCs and AuNCs/pDNA nanocomplexes before the AuNC vectors were applied for transfection study (Fig. 2d and Fig. S5–S7, ESI†). In the cell viability MTT and LDH assays, both AuNCs and AuNCs/pDNA nanocomplexes were non-toxic to cells at tested concentrations from 0 to 200 μg mL−1 AuNCs. All these phenomena indicated that the protamine–AuNCs had high pDNA condensing ability and excellent biocompatibility. The low acute toxicity nature and enhanced permeability and retention (EPR) effect of the AuNCs/pDNA nanocomplexes make protamine–AuNCs an excellent candidate as an effective CRISPR/Cas9 delivery tool for gene cancer therapy for both in vitro and in vivo applications.
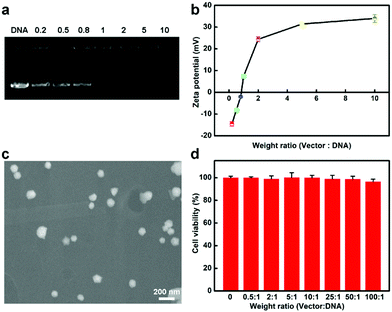 |
| Fig. 2 (a) Agarose gel electrophoresis of protamine–AuNCs/pDNA complexes with weight ratios of 0, 0.2, 0.5, 0.8, 1, 2, 5, and 10. The loading amount of pDNA was kept constant at 0.5 μg. (b) Zeta potentials of protamine–AuNCs/pDNA complexes with distinct weight ratios. (c) The SEM image of the protamine–AuNCs/pDNA complex with a weight ratio of 10 : 1. Scale bar: 200 nm. (d) In vitro cytotoxicities of protamine–AuNCs/pDNA complexes with a constant pDNA amount of 2 μg mL−1 in U2OS cells. The values represent percentage cellular viability (meaning ± SD, n = 3). | |
Efficient delivery of the AuNCs/pDNA nanocomplexes into cells
Encouraged by the high biocompatibility, as well as the appropriate dimensions and positive surface charge, we next assessed the ability of protamine–AuNCs to deliver Cas9–gRNA plasmids into cultured cells. Confocal laser scanning microscopy (CLSM) was utilized to determine the localization of the protamine–AuNCs/plasmid nanocomplexes with FITC-labeled plasmids (Fig. 3a). The U2OS cells incubated with FITC-labeled plasmid DNA alone showed a negligible fluorescence signal, which was in good agreement with the fact that naked plasmid DNA alone has difficulty in penetrating the cytoplasmic membrane and is susceptible to nuclease degradation.59 Remarkably, bright red and green fluorescence respectively representing protamine–AuNCs and plasmids was co-localized in the cytoplasm and nuclei after treatment with the protamine–AuNCs/plasmid nanocomplexes.60 In addition, a high intracellular uptake was further confirmed by flow cytometry analysis (Fig. 3b and c). In consistence with the fluorescence imaging results, no evident red or green fluorescence signals were detected in the blank cells without treatment. In contrast, cells treated with protamine–AuNCs/FITC-labeled plasmid nanocomplexes exhibited a significant emission intensity increase in both the red channel and green channel, which indicated the effective accumulation of protamine–AuNCs and FITC-labeled plasmids inside the cells. Collectively, all these phenomena strongly indicated the effectiveness of utilizing protamine–AuNCs for nucleic delivery of Cas9–gRNA plasmids.
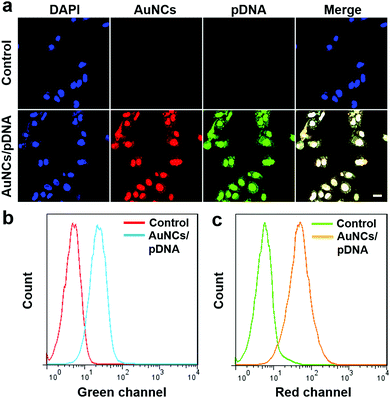 |
| Fig. 3 (a) Confocal fluorescence images of U2OS cells incubated with the pDNA (control) and protamine–AuNCs/pDNA complexes for 4 h. The pDNA was labeled with FITC. (b and c) Flow cytometry analysis of U2OS cells incubated with protamine–AuNCs/pDNA nanocomplexes. Cells were analyzed using the red channel for protamine–AuNCs and the green channel for FITC-labeled plasmids. | |
Effective gene editing in cancer cells
Based on the evidence that the Cas9–gRNA plasmids would effectively get into the cellular nucleus, we further evaluated the gene-editing efficiency of the Cas9–gRNA plasmid DNA by the formation of insertion/deletion (indel) mutations through the introduction of site-specific genomic breaks and subsequent repair via nonhomologous end joining. As a model, we investigated the genome-editing efficacy of the EGFP gene locus in U2OS.EGFP cells.61 To target the EGFP gene, we built two types of all-in-one Cas9–gRNA plasmids, encoding both the Cas9 gene and two specific EGFP gRNAs (gRNA1EGFP and gRNA2EGFP), according to previously reported methods.4 The successful construction of the Cas9–gRNA plasmids and cloned sequences of gRNAs were verified by gel electrophoresis analysis and the Sanger sequencing technique, respectively (Fig. 4a–c).19 After that, we quantitatively determined the impact of protamine–AuNCs/Cas9–gRNAEGFP plasmid nanocomplexes on EGFP expression in the cells using the CLSM image, flow cytometry analysis, and T7 endonuclease 1 (T7E1) assay. As can be seen in Fig. 4d and e, treatment with the AuNCs/Cas9–gRNAEGFP plasmid nanocomplexes converted approximately 30% of the EGFP-positive U2OS cells to EGFP-negative cells, while the Cas9–gRNAEGFP plasmids alone showed negligible influences on EGFP expression in comparison with no treatment, confirming the important role of the AuNC nanocarriers in mediating intracellular delivery of Cas9–gRNA plasmids and subsequent effective GFP knockout.62 To assess whether EGFP genome disruption could be attributed to indel mutations, we carried out the T7E1 assay to quantify the frequency of indel formation at the targeted genome site.4,63 As illustrated in Fig. S8 (ESI†), clear DNA cleavage bands could be observed in the targeted gene locus after treatment with the AuNCs/Cas9–gRNAEGFP plasmid nanocomplexes following the T7E1 assay. Quantitative analysis revealed an indel rate of about 29% in cells incubated with the AuNCs/Cas9–gRNAEGFP plasmid nanocomplexes, closely paralleling the data of flow cytometry. In contrast, no indels could be detected in the Cas9–gRNAEGFP plasmid-alone treated and control cells. Taken together, all these data suggested that the AuNCs/Cas9–gRNA plasmid nanocomplexes achieved significant genome disruption at the precise target gene site.
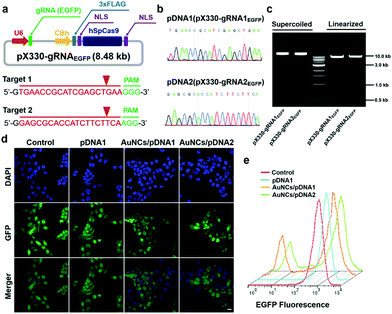 |
| Fig. 4 GFP disruption using the protamine–AuNCs/pDNA nanocomplexes. (a and b) pX330–gRNAEGFP design and synthesis. (c) Gel electrophoresis analysis of pX330–gRNAEGFP. (d) CLSM images of U2OS.EGFP cells receiving nanocomplex treatments. Scale bar represents 20 μm. (e) Flow cytometry analysis of U2OS.EGFP cells incubated with the protamine–AuNCs/pDNA nanocomplexes. | |
HPV oncogene disruption using the AuNCs/Cas9–gRNA plasmid nanocomplexes
To further evaluate the potential of the AuNCs/Cas9–gRNA plasmid nanocomplexes for gene therapeutic applications, human papillomavirus (HPV) type 18 (HPV18)-E7 oncogene was selected as the therapeutic model. HPV18-E7 is well known to enhance aberrant cellular proliferation and thence HPV pathogenesis.19,64 In cancer transformation and progression, E7 proteins straightly bind to retinoblastoma protein tumor suppressors, leading to retinoblastoma protein degradation. Previous reports suggested that the disruption of HPV18-E7 could suppress cancer cell proliferation and result in tumor cell apoptosis.17 We used two gRNAs, one as a control and one targeting HPV18-E7 oncogene (Fig. 5a), to test the inhibition efficiency of HeLa cellular proliferation. As illustrated in Fig. 5b, the group incubated with the AuNCs/Cas9–gRNAE7 plasmid nanocomplexes was effective in inhibiting tumor cell proliferation, reaching 72.8% cell viability as compared to the control groups.
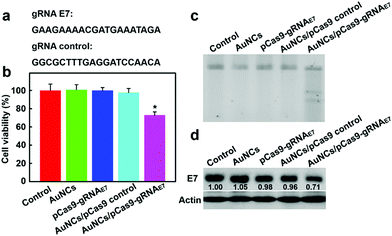 |
| Fig. 5 E7 gene disruption by protamine–AuNCs/pDNA nanocomplexes. (a) The sgRNA sequence of HPV-18 E7. (b) Cell proliferation determined 48 h post-transfection. Data are reported as the mean ± standard deviation (SD) from three independent experiments with three replicates in each. Statistical differences were evaluated using Student's t-test and the asterisk indicates statistically significant differences (*P < 0.005). (c) T7EI assay to verify E7 gene disruption. (d) Examination of HPV E7 protein in HeLa cells incubated with protamine–AuNCs/pDNA complexes by western blot analysis. | |
We next analyzed the indel mutation induced by the AuNCs/Cas9–gRNAE7 plasmid nanocomplexes with T7E1 assay. As presented in Fig. 5c, the mutation frequency reaching approximately 27.5% was detected in the HeLa cells incubated with the AuNCs/Cas9–gRNAE7 plasmid nanocomplexes. In contrast, the Cas9–gRNAE7 plasmid alone and the AuNCs/Cas9 with the control gRNA plasmid nanocomplexes as negative controls did not affect the proliferation of HeLa cells or HPV gene disruption. These results indicated that the AuNCs/Cas9–gRNAE7 plasmid nanocomplexes could effectively inhibit the target gene expression.65 We also carried out western blot analysis to further identify whether the AuNCs/Cas9–gRNAE7 plasmid nanocomplex-mediated HPV gene depletion would cause a decrease in the expression level of HPV18-E7 protein. Consistent with the findings of the T7E1 assay, western blotting assay in Fig. 5d indicated that the treatment of AuNCs/Cas9–gRNAE7 plasmid nanocomplexes induced about 29% down-regulation of HPV expression at the protein level. The control groups, including the AuNCs, Cas9–gRNAE7 plasmid alone and the AuNCs/Cas9 with the control gRNA plasmid nanocomplexes, had almost no effect on HPV expression. More importantly, this technology indicated high specificity and no significant off-target effects (Fig. S9, ESI†). Collectively, all these results confirmed again that AuNCs assembled with Cas9–gRNAE7 plasmids could accurately disrupt the HPV E7 oncogene, confirming their great application potential in cancer gene therapy.
Conclusions
By taking advantage of both the dense DNA packaging ability of protamine and the high biocompatibility of AuNCs, we here develop protamine-templated AuNCs assembled with all-in-one Cas9–gRNA plasmids to achieve targeted gene disruption while simultaneously acting as an imaging nanoagent. To the best of our knowledge, this protamine–AuNC-based novel delivery vehicle represents the first example of AuNC nanocomponents as nanocarriers to deliver Cas9–sgRNA plasmids for genome editing. The utilization of protamine–AuNCs achieves several unprecedented merits. Firstly, the protamine–AuNCs can facilitate intranuclear delivery of the Cas9–sgRNA plasmids, which is a precondition for realizing efficient CRISPR/Cas9-based gene editing. Secondly, protamine–AuNCs with excellent fluorescence properties endow our nanoplatform with great potential for imaging tracking. In addition, the AuNCs/Cas9–gRNA plasmid nanocomplexes are able to efficiently achieve genome disruption in different cancer cells, which may greatly broaden their further applications in cancer therapeutics. Moreover, as a proof of concept, the AuNCs/Cas9–gRNAE7 plasmid nanocomplexes can effectively knock out the oncogenic E7 gene, which suppressed the proliferation of HeLa cancer cells, validating the successful use of CRISPR/Cas9-based gene editing for therapeutics. Our study provides powerful evidence that protamine–AuNCs can be applied as effective CRISPR/Cas9 delivery nanoagents for genomic therapy.
Conflicts of interest
There are no conflicts to declare.
Acknowledgements
This work is supported by the National Key Research and Development Program of China (2019YFA0111300), the Guangdong Province Science and Technology Innovation Special Fund (International Scientific Cooperation, 2018A050506035), and the National Natural Science Foundation of China (51903256 and 21907113).
Notes and references
- F. A. Ran, P. D. Hsu, C. Y. Lin, J. S. Gootenberg, S. Konermann, A. E. Trevino, D. A. Scott, A. Inoue, S. Matoba, Y. Zhang and F. Zhang, Cell, 2013, 154, 1380–1389 CrossRef CAS
.
- P. D. Hsu, E. S. Lander and F. Zhang, Cell, 2014, 157, 1262–1278 CrossRef CAS
.
- O. Shalem, N. E. Sanjana, E. Hartenian, X. Shi, D. A. Scott, T. Mikkelson, D. Heckl, B. L. Ebert, D. E. Root, J. G. Doench and F. Zhang, Science, 2014, 343, 84–87 CrossRef CAS
.
- F. A. Ran, P. D. Hsu, J. Wright, V. Agarwala, D. A. Scott and F. Zhang, Nat. Protoc., 2013, 8, 2281–2308 CrossRef CAS
.
- S. Konermann, M. D. Brigham, A. E. Trevino, J. Joung, O. O. Abudayyeh, C. Barcena, P. D. Hsu, N. Habib, J. S. Gootenberg, H. Nishimasu, O. Nureki and F. Zhang, Nature, 2015, 517, 583–588 CrossRef CAS
.
- J. A. Doudna and E. Charpentier, Science, 2014, 346, 1258096 CrossRef
.
- S. Q. Tsai, N. Wyvekens, C. Khayter, J. A. Foden, V. Thapar, D. Reyon, M. J. Goodwin, M. J. Aryee and J. K. Joung, Nat. Biotechnol., 2014, 32, 569–576 CrossRef CAS
.
- P. Mali, L. Yang, K. M. Esvelt, J. Aach, M. Guell, J. E. DiCarlo, J. E. Norville and G. M. Church, Science, 2013, 339, 823 CrossRef CAS
.
- E. Ju, T. Li, S. Ramos da Silva and S.-J. Gao, ACS Appl. Mater. Interfaces, 2019, 11, 34717–34724 CrossRef CAS
.
- C. Liu, L. Zhang, H. Liu and K. Cheng, J. Controlled Release, 2017, 266, 17–26 CrossRef CAS
.
- Z. Tan, Y. Jiang, M. S. Ganewatta, R. Kumar, A. Keith, K. Twaroski, T. Pengo, J. Tolar, T. P. Lodge and T. M. Reineke, Macromolecules, 2019, 52, 8197–8206 CrossRef CAS
.
- L. Li, L. Song, X. Liu, X. Yang, X. Li, T. He, N. Wang, S. Yang, C. Yu, T. Yin, Y. Wen, Z. He, X. Wei, W. Su, Q. Wu, S. Yao, C. Gong and Y. Wei, ACS Nano, 2017, 11, 95–111 CrossRef CAS
.
- P. Wang, L. Zhang, W. Zheng, L. Cong, Z. Guo, Y. Xie, L. Wang, R. Tang, Q. Feng, Y. Hamada, K. Gonda, Z. Hu, X. Wu and X. Jiang, Angew. Chem., Int. Ed., 2018, 57, 1491–1496 CrossRef CAS
.
- R. J. Platt, S. Chen, Y. Zhou, M. J. Yim, L. Swiech, H. R. Kempton, J. E. Dahlman, O. Parnas, T. M. Eisenhaure, M. Jovanovic, D. B. Graham, S. Jhunjhunwala, M. Heidenreich, R. J. Xavier, R. Langer, D. G. Anderson, N. Hacohen, A. Regev, G. Feng, P. A. Sharp and F. Zhang, Cell, 2014, 159, 440–455 CrossRef CAS
.
- J.-C. Nault, S. Datta, S. Imbeaud, A. Franconi, M. Mallet, G. Couchy, E. Letouzé, C. Pilati, B. Verret, J.-F. Blanc, C. Balabaud, J. Calderaro, A. Laurent, M. Letexier, P. Bioulac-Sage, F. Calvo and J. Zucman-Rossi, Nat. Genet., 2015, 47, 1187–1193 CrossRef CAS
.
- X. Han, Z. Liu, M. C. Jo, K. Zhang, Y. Li, Z. Zeng, N. Li, Y. Zu and L. Qin, Sci. Adv., 2015, 1, e1500454 CrossRef
.
- P. Wang, L. Zhang, Y. Xie, N. Wang, R. Tang, W. Zheng and X. Jiang, Adv. Sci., 2017, 4, 1700175 CrossRef
.
- Y. Pan, J. Yang, X. Luan, X. Liu, X. Li, J. Yang, T. Huang, L. Sun, Y. Wang, Y. Lin and Y. Song, Sci. Adv., 2019, 5, eaav7199 CrossRef CAS
.
- Y. H. Lao, M. Li, A. Gao Madeleine, D. Shao, C. W. Chi, D. Huang, S. Chakraborty, T. C. Ho, W. Jiang, H. X. Wang, S. Wang and W. Leong Kam, Adv. Sci., 2018, 5, 1700540 CrossRef
.
- X. Zhang, C. Xu, S. Gao, P. Li, Y. Kong, T. Li, Y. Li, F.-J. Xu and J. Du, Adv. Sci., 2019, 6, 1900386 CrossRef
.
- Y. Lyu, S. He, J. Li, Y. Jiang, H. Sun, Y. Miao and K. Pu, Angew. Chem., Int. Ed., 2019, 58, 18197–18201 CrossRef CAS
.
- Y. Liu, G. Zhao, C.-F. Xu, Y.-L. Luo, Z.-D. Lu and J. Wang, Biomater. Sci., 2018, 6, 1592–1603 RSC
.
- H.-X. Wang, Z. Song, Y.-H. Lao, X. Xu, J. Gong, D. Cheng, S. Chakraborty, J. S. Park, M. Li, D. Huang, L. Yin, J. Cheng and K. W. Leong, Proc. Natl. Acad. Sci. U. S. A., 2018, 115, 4903 CrossRef CAS
.
- J. S. Chin, W. H. Chooi, H. Wang, W. Ong, K. W. Leong and S. Y. Chew, Acta Biomater., 2019, 90, 60–70 CrossRef CAS
.
- C. Liang, F. Li, L. Wang, Z.-K. Zhang, C. Wang, B. He, J. Li, Z. Chen, A. B. Shaikh, J. Liu, X. Wu, S. Peng, L. Dang, B. Guo, X. He, D. W. T. Au, C. Lu, H. Zhu, B.-T. Zhang, A. Lu and G. Zhang, Biomaterials, 2017, 147, 68–85 CrossRef CAS
.
- L. Zhang, P. Wang, Q. Feng, N. Wang, Z. Chen, Y. Huang, W. Zheng and X. Jiang, NPG Asia Mater., 2017, 9, e441 CrossRef CAS
.
- Z. Chen, F. Liu, Y. Chen, J. Liu, X. Wang, A. T. Chen, G. Deng, H. Zhang, J. Liu, Z. Hong and J. Zhou, Adv. Funct. Mater., 2017, 27, 1703036 CrossRef
.
- Y. Lin, J. Wu, W. Gu, Y. Huang, Z. Tong, L. Huang and J. Tan, Adv. Sci., 2018, 5, 1700611 CrossRef
.
- S. M. Kim, Y. Yang, S. J. Oh, Y. Hong and M. Jang, J. Controlled Release, 2017, 266, 8–16 CrossRef CAS
.
- X. Chen, Y. Chen, H. Xin, T. Wan and Y. Ping, Proc. Natl. Acad. Sci. U. S. A., 2020, 117, 2395 CrossRef CAS
.
- X.-Y. He, X.-H. Ren, Y. Peng, J.-P. Zhang, S.-L. Ai, B.-Y. Liu, C. Xu and S.-X. Cheng, Adv. Mater., 2020, 32, 2000208 CrossRef CAS
.
- T. Wan, D. Niu, C. Wu, F.-J. Xu, G. Church and Y. Ping, Mater. Today, 2019, 26, 40–66 CrossRef CAS
.
- H.-X. Wang, M. Li, C. M. Lee, S. Chakraborty, H.-W. Kim, G. Bao and K. W. Leong, Chem. Rev., 2017, 117, 9874–9906 CrossRef CAS
.
- T.-C. Yang, C.-Y. Chang, A. A. Yarmishyn, Y.-S. Mao, Y.-P. Yang, M.-L. Wang, C.-C. Hsu, H.-Y. Yang, D.-K. Hwang, S.-J. Chen, M.-L. Tsai, Y.-H. Lai, Y. Tzeng, C.-C. Chang and S.-H. Chiou, Acta Biomater., 2020, 101, 484–494 CrossRef CAS
.
- X. Xu, T. Wan, H. Xin, D. Li, H. Pan, J. Wu and Y. Ping, J. Gene Med., 2019, 21, e3107 CrossRef
.
- Y. Tao, E. Ju, J. Ren and X. Qu, Chem. Commun., 2013, 49, 9791–9793 RSC
.
- Y. Du, H. Sheng, D. Astruc and M. Zhu, Chem. Rev., 2020, 120, 526–622 CrossRef CAS
.
- Y. Tao, M. Li, J. Ren and X. Qu, Chem. Soc. Rev., 2015, 44, 8636–8663 RSC
.
- L. Han, J.-M. Xia, X. Hai, Y. Shu, X.-W. Chen and J.-H. Wang, ACS Appl. Mater. Interfaces, 2017, 9, 6941–6949 CrossRef CAS
.
- D. Chen, B. Li, S. Cai, P. Wang, S. Peng, Y. Sheng, Y. He, Y. Gu and H. Chen, Biomaterials, 2016, 100, 1–16 CrossRef CAS
.
- H. Zhu, J. Li, J. Wang and E. Wang, ACS Appl. Mater. Interfaces, 2019, 11, 36831–36838 CrossRef CAS
.
- A. Yahia-Ammar, D. Sierra, F. Mérola, N. Hildebrandt and X. Le Guével, ACS Nano, 2016, 10, 2591–2599 CrossRef CAS
.
- Y. Xie, Y. Xianyu, N. Wang, Z. Yan, Y. Liu, K. Zhu, S. Hatzakis Nikos and X. Jiang, Adv. Funct. Mater., 2018, 28, 1702026 CrossRef
.
- Y.-Q. Huang, S. Fu, Y.-S. Wang, J.-H. Xue, X.-L. Xiao, S.-H. Chen and B. Zhou, Anal. Bioanal. Chem., 2018, 410, 7385–7394 CrossRef CAS
.
- L. R. Brewer, M. Corzett and R. Balhorn, Science, 1999, 286, 120 CrossRef CAS
.
- N. H. Kim, C. Provoda and K.-D. Lee, Mol. Pharmaceutics, 2015, 12, 342–350 CrossRef CAS
.
- T. Z. Jia, A. C. Fahrenbach, N. P. Kamat, K. P. Adamala and J. W. Szostak, Nat. Chem., 2017, 9, 1286 CrossRef CAS
.
- V. Biju, A. Anas, H. Akita, E. S. Shibu, T. Itoh, H. Harashima and M. Ishikawa, ACS Nano, 2012, 6, 3776–3788 CrossRef CAS
.
- S. S. Priya, M. R. Rekha and C. P. Sharma, Carbohydr. Polym., 2014, 102, 207–215 CrossRef CAS
.
- R. Mo, T. Jiang and Z. Gu, Angew. Chem., Int. Ed., 2014, 53, 5815–5820 CrossRef CAS
.
- Y.-Q. Huang, L.-N. Yang, Y.-S. Wang, J.-H. Xue and S.-H. Chen, Microchim. Acta, 2018, 185, 483 CrossRef
.
- R. K. DeLong, U. Akhtar, M. Sallee, B. Parker, S. Barber, J. Zhang, M. Craig, R. Garrad, A. J. Hickey and E. Engstrom, Biomaterials, 2009, 30, 6451–6459 CrossRef CAS
.
- J.-H. Xue, L. Liu, Y.-S. Wang, J.-Q. Li, M. Li, Y.-N. Qu and L. Li, Microchim. Acta, 2019, 186, 700 CrossRef CAS
.
- S.-N. Ding, C.-M. Li and N. Bao, Biosens. Bioelectron., 2015, 64, 333–337 CrossRef CAS
.
- J. H. Xue, K. P. Xiao, Y. S. Wang, L. Liu, J. Q. Li, M. Li, Y. N. Qu and X. L. Xiao, Colloids Surf., B, 2020, 189, 110873 CrossRef CAS
.
- Y. N. Wang, X. J. Wang, X. Q. Ma, Q. Chen, H. He, W. M. Nau and F. Huang, Part. Part. Syst. Charact., 2019, 36, 1900281 CrossRef CAS
.
- Y. Tao, Z. Li, J. Ren and X. Qu, Nanoscale, 2013, 5, 6154–6160 RSC
.
- Y. Shan, T. Luo, C. Peng, R. Sheng, A. Cao, X. Cao, M. Shen, R. Guo, H. Tomás and X. Shi, Biomaterials, 2012, 33, 3025–3035 CrossRef CAS
.
- Y. Tao, Y. Zhang, E. Ju, H. Ren and J. Ren, Nanoscale, 2015, 7, 12419–12426 RSC
.
- W. Sun, W. Ji, J. M. Hall, Q. Hu, C. Wang, C. L. Beisel and Z. Gu, Angew. Chem., Int. Ed., 2015, 54, 12029–12033 CrossRef CAS
.
- Y. Fu, J. A. Foden, C. Khayter, M. L. Maeder, D. Reyon, J. K. Joung and J. D. Sander, Nat. Biotechnol., 2013, 31, 822–826 CrossRef CAS
.
- M. Hansen-Bruhn, B. E.-F. de Ávila, M. Beltrán-Gastélum, J. Zhao, D. E. Ramírez-Herrera, P. Angsantikul, K. V. Gothelf, L. Zhang and J. Wang, Angew. Chem., Int. Ed., 2017, 57, 2657–2661 CrossRef
.
- Q. Cheng, T. Wei, L. Farbiak, L. T. Johnson, S. A. Dilliard and D. J. Siegwart, Nat. Nanotechnol., 2020, 15, 313–320 CrossRef CAS
.
- C. A. Moody and L. A. Laimins, Nat. Rev. Cancer, 2010, 10, 550–560 CrossRef CAS
.
- L. Li, Z. Yang, S. Zhu, L. He, W. Fan, W. Tang, J. Zou, Z. Shen, M. Zhang, L. Tang, Y. Dai, G. Niu, S. Hu and X. Chen, Adv. Mater., 2019, 31, 1901187 CrossRef
.
Footnote |
† Electronic supplementary information (ESI) available. See DOI: 10.1039/d0tb01925a |
|
This journal is © The Royal Society of Chemistry 2021 |
Click here to see how this site uses Cookies. View our privacy policy here.