DOI:
10.1039/D0TB02094J
(Review Article)
J. Mater. Chem. B, 2021,
9, 23-34
Biomaterial-assisted drug delivery for interstitial cystitis/bladder pain syndrome treatment
Received
28th August 2020
, Accepted 9th October 2020
First published on 14th October 2020
Abstract
Interstitial cystitis/bladder pain syndrome (IC/BPS) is a chronic and painful bladder condition afflicting patients with increased urinary urgency and frequency as well as incontinence. Owing to the elusive pathogenesis of IC/BPS, obtaining effective therapeutic outcomes remains challenging. Current administrational routes such as intravesical-bladder injection improve the treatment efficacy and reduce systemic side effects. However, the bladder permeability barrier hinders drug penetration into the bladder wall to meet the desired therapeutic expectation. These issues can be addressed by encapsulating drugs into biomaterials. When appropriately exploited, they would increase the drug dwelling time in the bladder, enhance the penetration of mucosa and improve the therapeutic response of IC/BPS. In this review, we first elucidate the pathogenesis and animal models of IC/BPS. Then, we highlight recent representative biomaterial-assisted drug delivery systems for IC/BPS treatment. Finally, we discuss the challenges and outlook for further developing biomaterial-based delivery systems for IC/BPS management.
1. Introduction
Interstitial cystitis, also known as bladder pain syndrome (IC/BPS), is a chronic disease leading to major detrimental impacts on a patient's quality of life.1,2 Symptoms of IC/BPS include pelvic pain and urinary storage dysfunctions such as urinary frequency, urgency, and nocturia.3 The cases of the IC/BPS have been skyrocketing in recent years. It has been estimated that over 10 million individuals (3–7% in women and 2–4% in men) suffer from IC/BPS.4,5 In terms of the etiology, IC/BPS involves alterations of epithelium via interacting with glycosaminoglycan, activating mast cells, reposing autoimmunity, and interacting with central sensitization.6–9 Unfortunately, the treatments of IC/BPS are still based on empirical studies and suffer from low efficacy.
Oral administration and intravesical drug instillations are the main therapeutic strategies, along with physical/behavior therapy for IC/BPS treatment clinically.10 Specifically, pentosan polysulfate sodium (PPS) is an oral drug approved by the US Food and Drug Administration (FDA), but its therapeutic effect is insignificant compared to the placebo.11 Besides drug delivery through the oral route, intradetrusor injection is another administration method. Intradetrusor injection of botulinum toxin A (BTX-A) provides the fourth-line therapy of IC/BPS, but it may lead to infection, hemorrhage and pelvic discomfort.12 As the treatment options for IC/BPS are limited and ineffective, the development of effective and efficient treatment strategies for IC/BPS is demanding.
Biomaterials with desired biological features and functions gain much attention as the drug delivery platform for IC/BPS treatment in recent years.1,13,14 The drug delivery platform is an approach for prolonged contact between the targeted tissue and the available drug.15 Exploiting biomaterials for IC/BPS treatment can improve the therapeutic outcome in many ways. Firstly, biomaterial carriers can protect drugs from degradation and prevent premature drug release. Secondly, targeted drug delivery can improve therapeutic efficacy and minimize undesired side-effects. Thirdly, controllable drug release can prolong the drug residence time, which decreases the frequency of repeated administration and improve patient compliance.15,16 Thus, biomaterial-assisted drug delivery is a promising strategy for treating IC/BPS.
In this review, we summarized recent progress in the pathogenesis and animal models of IC/BPS. Then, we discussed the feasibility and advantages of biomaterial-assisted drug delivery for IC/BPS therapy (Fig. 1).
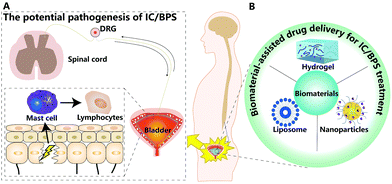 |
| Fig. 1 Schematic illustration of (A) potential pathogenesis and (B) biomaterial-assisted drug delivery for treatment in IC/BPS. (A) Initial damage destroys the bladder permeability barrier and induces inflammatory response to trigger autoimmunity response, activation of MCs and neurogenic inflammation in the central nervous system. (B) Liposomes, nanoparticles and hydrogels have been developed as delivery systems for IC/BPS treatment. MCs: mast cells. DRG: dorsal root ganglia. | |
2. Pathogenesis of IC/BPS
The underlying cause of IC/BPS is not fully understood and the disease mechanism remains elusive.17 In the clinical analysis of IC/BPS patients, the possible etiologies accounting for IC/BPS include reduction of the glycosaminoglycan (GAG) layer on the urothelium, activation of the mast cell, proliferation of nerve fiber, progression of post-infection autoimmune disease and development of neurogenic inflammation.6,18,19 In this section, we discuss possible etiological factors that play roles in the development of IC/BPS.
2.1 Epithelial paracellular permeability
The bladder urothelium consists of the basal cell layer, intermediate cell layer, and umbrella cell layer. The bladder urothelium separates the urinary space from underlying tissue and withholds the entry of harmful substances and pathogens. The outmost umbrella cell layer is formed by interconnecting with tight junctions (TJs) and adheren junctions (AJs).20 The barrier integrity of TJs, AJs, and urothelium is essential to prevent the solutes from moving into the intercellular space.20 Nowadays, there is an increasing number of reports on the mechanism of dysfunction of TJs and AJs associated with the IC/BPS.21–24 For example, Jhang et al. discovered that the lateral interstitial space of umbrella cells was widened in IC/BPS patients.25 Moreover, Carattino and coworkers reported that the overexpression of the pore-forming TJ-associated protein claudin-2 (CLDN-2) in the umbrella cells enhanced the permeability of the paracellular route.26 Meanwhile, Monastyrskaya et al. indicated that the microRNA miR-199a-5p, as a crucial regulator of urothelial integrity, was closely related to the control of urothelial permeability in IC/BPS.20 Generally, the symptoms in IC/BPS could be ascribed to the increase of urothelial paracellular permeability, thereby leaking the irritants into intermediated layers and eliciting inflammatory responses (Fig. 2).
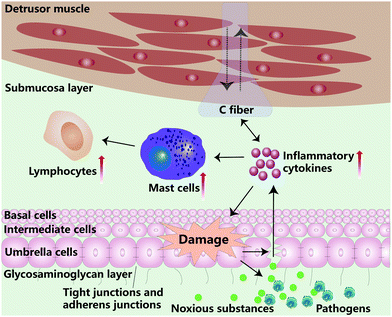 |
| Fig. 2 Schematic illustration of epithelial paracellular permeability and glycosaminoglycan theory in IC/BPS. The primary damage destroys the bladder permeability barrier leading to the entry of noxious substances through interstitial space, which triggers inflammation responses. | |
2.2 Glycosaminoglycan layer impairment
The bladder permeability barrier (BPB) is an impenetrable barrier between the blood and the urine, and its function is similar to the blood–brain barrier. The BPB is comprised of the urothelium and GAG layer.15 GAGs are made of hyaluronate, chondroitin sulfate (CS), dermatan sulfate, heparin and heparan sulfate, which are important biologics for anti-adhesion of particles from urine.6 Research has shown that impaired GAG degradation could lead to frequent micturition and pain in the pelvic region.27 Janssen et al. found that the removal of the GAG layer would lead to increased urothelial permeability.28 Meanwhile, in a clinical study, intravesical therapy with hyaluronic acid (HA) and CS could mitigate patient bladder pain, reduce the urination frequency and urgency of some IC/BPS patients.29 CS located on the urothelial luminal surface could facilitate regulation of the barrier function of the urothelium.28 Moreover, in an in vitro IC/BPS study, exogenous replenishment of both HA and CS achieved anti-inflammatory activity by down-regulating the secretion of interleukin-6 (IL-6), interleukin-8 (IL-8) and nuclear factor kappa-B (NF-κB) under the tumour necrosis factor-α (TNF-α) rich environment.30 This result suggested that utilizing biologics like HA/CS have an anti-inflammatory effect and facilitate bladder barrier reconstruction.
The loss of the GAG layer attracts irritants from urine to attach onto the epithelium. An increase in urothelial permeability could trigger chronic inflammatory responses and eventually damages BPB (Fig. 2). Thus, GAG replenishment leads to the restoration of the normal bladder barrier function.
2.3 Mast cell activation
Mast cells (MCs) are immune cells that originated from hematopoietic stem cells in the bone marrow. MCs are crucial in regulating immune responses. In a clinical study, MC accumulation (mastocytosis) was present in the bladder tissue of IC/BPS patients.9 In another animal study, inflammatory cytokines such as TNF-α, interleukin-1β (IL-1β), and IL-6 were over-expressed in the IC/BPS rat model, when the number of MCs increased.31 Recent studies have indicated that MCs could be activated by multiple biological substances, such as drug molecules, chemokines, bacteria, viral components, IgE, and other biologics.32,33 The activated MCs secrete a variety of bioactive compounds, including biogenic amines, enzymes, proteoglycans, growth factors, cytokines and chemokines.33 Among these secreted factors, histamine specifically targets and activates histamine receptor 3 (H3R), which subsequently leads to hyper-inflammation in the bladder (Fig. 3).34 Also, tryptase activates the protease-activated receptor-2 (PAR-2), which evokes sustained pain hypersensitivity through TRPV1 channel activation for neuropeptide release from nerve terminals.35 The nerve growth factor (NGF) interacts with its receptor TrkA, which in turn activates TRPV1 channels to sense pain (Fig. 3). Interestingly, one recent study found that MC infiltration in the bladder is related to the reduction of E-cadherin and P-glycoprotein expression, positively modulating the uroepithelial layer permeability.36 Of note, cromolyn, as an MC membrane stabilizer could suppress MC infiltration in the bladder and restored voiding function in a cystitis model.37
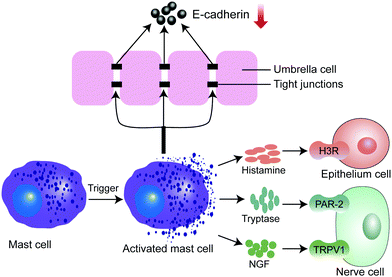 |
| Fig. 3 Schematic illustration of MC theory in IC/BPS. The activated MC release bioactive compounds, including histamine, tryptase, and NGF, that activate the target tissue. Moreover, MC infiltration in bladder decreases the expression of E-cadherin. MC: mast cell. NGF: never growth factor. H3R: histamine receptor 3. PAR-2: protease-activated receptor-2. TRPV1: transient receptor potential vanilloid 1. | |
In a word, the infiltration and activation of MCs can induce the inflammatory response, disrupt the BPB and trigger the signaling pathway in pathological pain during the development of IC/BPS.
2.4 Autoimmunity response
There is mounting evidence that IC/BPS might be an autoimmune disease.38 A higher prevalence in females may provide researchers clues on autoimmune pathogenesis.12 Clinical evidence suggests a strong association between IC/BPS and autoimmune disease such as Sjögren syndrome (SS), ulcerative colitis, systemic lupus erythematosus (SLE), and rheumatoid arthritis (RA).39–41 In addition, antibodies that recognize uroepithelial cells were largely found in the urine of IC/BPS patients.42 There's increasing evidence showing that the bladder tissue of IC/BPS patients has an abundance of CD4+, CD8+ and γδ T cells, as well as IgA, IgG and IgM plasma cells.43,44 Moreover, another clinical investigation illustrated that IC/BPS patients had considerably higher levels of nitric oxide, lymphocytes, and MCs in histopathological examination.45In vitro studies revealed that the obvious distribution of CD3+ T cells was also found in the bladder of the cystitis model.46 Importantly, the level of inflammatory cytokine TNF-α was significantly elevated.46 Recently, a phase II clinical trial has shown that the initial of 80 mg of adalimumab via subcutaneous administration followed by 40 mg every two weeks for 12 weeks into IC/BPS patients had significant improvements in mitigating unwanted symptoms.47 Meanwhile, another study also confirmed certolizumab pegol in alleviating the symptoms of women with moderate to severe refractory IC/BPS.48 Despite all these promising studies, the clinical trials have solely performed in the theory of autoimmunity response and the conclusion drawn from these studies may be subjective and biased. Further research is required to fully understand the detailed pathway of autoimmunity responses in IC/BPS.
2.5 Central sensitization
As is well known, pelvic pain is one of the typical symptoms of IC/BPS.49 Recently, central sensitization has been demonstrated to lead to chronic pain.50 Central sensitization is a pathologic state wherein the central nervous system augments the signaling in response to the peripheral stimulus.50 In the peripheral nervous system, to respond to the stimulus of an extracellular environment, urothelial cells would release transmitters such as adenosine triphosphate (ATP), acetylcholine (ACh), and prostaglandin (PG).51 Then, these transmitters activate the afferent nerves, which convey the sensory information to the central nervous system. Persistent peripheral insults may lead to the alteration in the peripheral nervous system.51 We found that there was a high density of immunoreactive nerve fibre in the bladder tissue of IC/BPS patients than healthy ones, suggesting that the inflammatory reaction may cause alterations in the periphery.52 In the central nervous system, a preclinical study found that activated microglial cells with neurogenic inflammation persistently cause visceral pain in rats.8 Neuroinflammation caused by the interaction between glial and neuroimmune cells can drive chronic pain through central sensitization.53 Clinically, transcranial magnetic stimulation (TMS) is a non-invasive neurophysiological technique, which induces transient electricity in the precise position of the cerebrum to modify excitability. In a clinical crossover study, the TMS technique had significant effects on pain and lowered urinary tract symptoms (LUTS).54 These studies suggested that the central nervous system was involved in the pelvic pain progression and could be activated through numbers of external physicochemical stimuli. Moreover, several neuropeptides, including substance P (SP) and calcitonin gene-related peptide (CGRP), were found in activated bladder afferent neurons triggering inflammatory responses.50 These findings show that the effect of central sensitization can act as a vicious cycle leading to hyperexcitability during the progression of IC/BPS (Fig. 4).
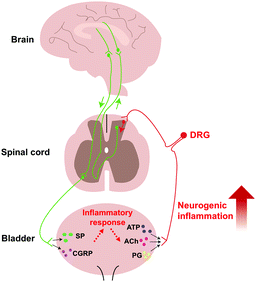 |
| Fig. 4 Schematic illustration of central sensitization in IC/BPS. Irritants such as ATP, ACh, and PG activate afferent nerves leading to neurogenic inflammation. Efferent nerve releases neuropeptides that trigger inflammatory response exacerbating the release of irritants. DRG: dorsal root ganglia. | |
IC/BPS is a chronic condition. This refractory disease is caused by multifactorial involvement such as urothelial and GAG defect, BPB breakage with increased permeability, MC infiltration, autoimmunity response, and central sensitization (Fig. 5).55
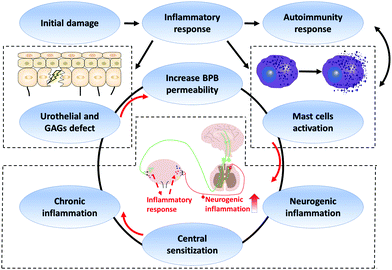 |
| Fig. 5 Schematic representation of IC/BPS pathogenesis. GAGs: glycosaminoglycans. BPB: bladder permeability barrier. | |
3. Animal model of IC/BPS
To better understand the occurrence and progression of IC/BPS, suitable animal models have been exploited to investigate the pathological mechanism and therapeutic approach of IC/BPS. So far, various IC/BPS animal models have been established to mimic the physiological environment of IC/BPS patients.56–60 In this section, we summarize the commonly used animal models of IC/BPS and discussed the pros and cons of each model (Table 1).
Table 1 Comparison of different types of IC/BPS animal models
Animal model |
Drug administration route |
Pathological changes of bladder |
Advantages |
Disadvantages |
Ref. |
CYP induced cystitis model |
Intraperitoneal injection of CYP |
Submucosal edema, hemorrhage, and inflammatory cell invasion |
It is widely used due to economy, good reproducibility and convenience. It can elicit not only the acute haemorrhagic cystitis but also the chronic cystitis. |
The proliferation of urothelium in mice bladder is contrary to the phenotype of IC/BPS patients. |
8, 31, 56 and 72–74
|
LPS induced cystitis model |
Intravesical administration of LPS |
MCs infiltration and urothelial denudation |
It is convenient to establish a chronic cystitis model. It can well represent the IC/BPS bladder conditions in humans. |
Repeated intubation may lead to the injury of the urethra and the infection. Anaesthesia is required for the operation. |
69–71, 75 and 76
|
EAC model |
Subcutaneous injection of bladder homogenate or UPK3A 65–84 peptide |
Infiltration of MCs and lymphocyte |
The symptoms are stable and lasting, facilitating the therapeutic effect evaluation. |
It is costly and time consuming. |
38, 43 and 77–81
|
WAS model |
Non-drug |
Infiltration and activation of MCs |
The symptoms are lasting without drug administration. It provides a novel way to study the role of psychological disorders in the pathogenesis of IC/BPS. |
The stability of symptoms should be further confirmed. |
82–85
|
Neurogenic cystitis model |
Injecting PRV into the abductor caudalis dorsalis muscle |
Infiltration of MCs and elevation of inflammatory cytokines |
This model can be rapidly established. The symptom of the visceral pain is typical. |
The symptoms last only a short time. |
59 and 86–90
|
3.1 Cyclophosphamide-induced cystitis model
Cyclophosphamide (CYP)-induced cystitis rodent models are commonly used to study IC/BPS. CYP can be metabolized to acrolein in the liver. The acrolein later accumulates in the bladder and causes bladder tissue damage.61 The CYP-induced cystitis model can also be used to simulate typical IC/BPS features such as activation of inflammation, pain-related behaviors, and bladder overactivity. In one study, the chronic cystitis was induced via repeated CYP intraperitoneal injection on rats. After that, H&E staining of bladder tissue showed submucosal oedema, vascular structure destruction, hemorrhage and inflammatory cell invasion in the cystitis tissue.31 Moreover, an acute cystitis rat model can also be established by a single high dose injection of CYP, which leads to severe oedema and hyperaemia in rat lamina propria.62 Interestingly, in the CYP-induced mice model of chronic cystitis, repeated CYP administration also induces urothelium hyperplasia, which is not consistent with the pathology of IC/BPS.63–65
In addition, visceral pain is another crucial aspect of evaluating the CYP-induced cystitis model. In the chronic cystitis rat model, the symptom of visceral pain lasted at least 7 days.8 The variation of visceral pain in this rat model helps researchers better evaluate the therapeutic efficacy and offers researchers insight into understanding the underlying pathology.
The protocol for the CYP-induced cystitis model is easy to operate on animals and it has good reproducibility.60 A high-dose CYP-induced cystitis model can elicit more severe oedema, hyperaemia and produce a higher level of inflammation cytokine in bladder tissue, which is inclined to haemorrhagic cystitis.62,66 Correspondingly, the chronic cystitis model induced by a repeated low dose of CYP is characterized by visceral pain and LUTS.31,62
3.2 Intravesical drug infusion on the cystitis model
Over the last few decades, despite researchers establishing the cystitis model to imitate the pelvic pain and LUTS of IC/BPS on rats, these models tend to simulate acute cystitis with a short life period.67,68 In order to obtain the long-lasting effect, the protamine sulphate and lipopolysaccharide (PS/LPS)-induced cystitis model was developed. LPS binds to the Toll-like receptor 4 receptor complex and could promote the secretion of multiple cytokines by inflammatory cells and produce inflammatory responses in tissues.69 In one study, Choi et al. found that MC degranulation in the bladder promotes the progression of inflammatory response in a PS/LPS-induced cystitis rat model.70 Moreover, a higher density of lymphoplasmacytic infiltration was also found in the PS/LPS-induced cystitis model than the healthy ones. These results suggest that MC infiltration and autoimmune response in the cystitis model can be correlated to IC/BPS patients' histopathology findings. Overall, the intravesical infusion cystitis model on rats can well represent the IC/BPS bladder conditions in humans with the advantage of easy operation.57,71
3.3 Experimental autoimmune cystitis model
Autoimmunity responses play important roles in the initiation and progression of IC/BPS as discussed above.40,44 Various experimental autoimmune cystitis (EAC) models have been developed.43,79
The EAC models can be categorized into nonspecific and specific ones. The nonspecific EAC model was induced by subcutaneous injection of bladder homogenate on the back of a C57BL/6 mouse.38 This nonspecific EAC model mouse showed lower abdominal pain threshold, more micturition frequency, and a higher expression of MCs and inflammatory cytokines in the bladder tissue than the healthy ones. However, a systemic elevation in the expressions of IL-1β, interferon-γ (IFN-γ), and TNF-α was observed within kidneys, liver, and lungs in this model.38
On the other hand, the specific EAC model is mainly induced by bladder-specific uroplakin. In one example, BALB/c mice were injected with an uroplakin 3A-derived immunogenic peptide (UPK3A 65–84).91 It was observed that the symptom of visceral pain remained at least 30 days, and the expression levels of MCs and inflammatory cytokines were upregulated in the bladder tissue.
Both nonspecific and specific EAC models induced by subcutaneous injection can provide long-term inflammatory response, which offers researchers opportunities to evaluate the therapeutic effect in a better way.
3.4 Water avoidance stress model
Numerous studies have shown that psychological disorders, such as anxiety disorder or stress, aggravate symptoms of IC/BPS.84,92 Therefore, to investigate the role of stress in IC/BPS, the water avoidance stress (WAS) model has been developed to evaluate the correlation of the potent psychological stress with LUTS. The WAS model can be induced in Wistar–Kyoto female rats placed on a water-filled container 1 hour per day for 10 days to develop visceral hyperalgesia and frequent micturition.82 In this model, pathological changes include the increase of the total number of activated MCs and destruction of vascular structures. However, the absence of inflammatory markers is also the hallmark of the WAS model.93 In another study, the symptoms of visceral hyperalgesia lasted for more than 1 month in the WAS rat model generated without instillation of chemically toxic substances or introduce of irritation.94
In short, continuous psychological disorders are related to the pathophysiology progression of the bladder.85,95 The psychological disorder can induce central neuronal alterations and LUTS, which is involved in the pathogenesis of IC/BPS.85 The WAS model with psychological alteration provides an innovative platform for clinical diagnosis and treatment of IC/BPS.
3.5 Neurogenic cystitis model
Central sensitization is related to the progression of IC/BPS.50 IC/BPS patients with visceral nociception often have histopathological changes in their bladder tissue and nervous system. Neurogenic cystitis model has been developed to study the aetiology of IC/BPS. The neurogenic cystitis model is firstly produced by injecting Bartha's strain of pseudorabies virus (PRV) into the abductor caudalis dorsalis (tail base) muscle.86 On day 3 post-infection, MCs were significantly increased in the bladder and the symptoms of pelvic pain also occurred.87 In addition, the PRV-induced cystitis model produced the symptom of allodynia and showed the pathological alterations in the spinal cord, suggesting that the central nervous system may be involved in maintaining pelvic pain.86 PRV promotes MC infiltration in bladder lamina propria and stimulates the inflammation of the central system which also leads to pelvic pain.59 However, the study revealed that symptoms disappeared quickly 5 days after virus injection.90 Taken together, the PRV-induced cystitis model is suitable for exploring the mechanism of neurogenic inflammation and the pathology of pelvic pain in IC/BPS, but within a short period.
4. Biomaterial approaches for IC/BPS treatment
Traditional treatments for IC/BPS have an obvious limitation with an unsatisfactory therapeutic effect. For instance, based on the GAG theory of IC/BPS, oral administration of FDA-approved PPS for IC/BPS treatment is used to restore the damaged GAG layer.13 However, recent clinical trials do not corroborate the aforementioned findings/conclusion.11 Intravesical drug delivery (IDD), is another promising therapeutic strategy. It can reduce systemic side effects and improve the treatment effect by maintaining local drug concentration.2 Nevertheless, IDD treatment has an issue with a low permeability of the urothelium. Another limitation of IDD is the periodical voiding which washes out the drug from the bladder and leads to drug loss and ineffective treatment.105
Biomaterial-based strategies have gained much attention in treating IC/BPS disease, such as liposomes and hydrogels with promising therapeutic outcomes. Recently, biomaterial-based drug delivery platforms have been developed to prolong drug retention in the bladder, enhance drug stability in the bladder environment, improve drug penetration, and control drug release.105 In this section, we highlight various emerging biomaterials for IC/BPS treatment (Table 2).
Table 2 Recent studies using biomaterial carriers in IDD for the treatment of IC/BPS
Biomaterial carriers |
Material |
Drug |
Properties |
Ref. |
Liposomes |
Carrier made of lipid bilayers |
BoNT-A/tacrolimus/antisense oligonucleotide |
Endocytosed by urothelium, it can carry both hydrophilic and hydrophobic agents |
96–101
|
Mucoadhesive nanoparticulate system |
Chitosan-TGA NPs |
Trimethoprim |
Greater stability, more superior mucoadhesion and more sustained and controlled drug release |
102
|
Thermosensitive hydrogel |
PEG–PLGA–PEG |
Misoprostol |
Simple formulation, biocompatibility, biodegradability |
103
|
Floating hydrogel |
P407 |
Heparin |
Floating in urine with microbubbles production without urinal obstruction, controllable release kinetics |
104
|
Thermosensitive hydrogel |
SELP |
Semisynthetic glycosaminoglycan ethers |
Controllable release kinetics, lower gelation, biocompatibility, biodegradability |
1
|
Thermosensitive hydrogel |
TC-3 gel |
BoNT-A |
It is feasible, safe in clinical research, biocompatibility, biodegradability |
14
|
4.1 Liposomes
Liposomes are the self-assembled vesicles composed of phospholipid bilayers surrounding the aqueous core.13 Liposomes have been applied for investigation of the treatment effect in IC/BPS because they act not only as the therapeutic agent but also as a drug delivery carrier.13 The clinical efficacy and safety of vesical liposomes were evaluated in 14 IC/BPS patients. Symptoms including bladder pain and urgency were relieved, and treatment-related adverse events were not observed.106 More importantly, as the drug delivery platform, liposomes with a unique structural feature can encapsulate both hydrophilic and hydrophobic agents, and reduce systemic side-effects of medication to improve the therapeutic effect. In addition, the mechanism of mucosa penetration for liposomes has been elucidated.107 Endocytosis mediated by clathrin plays an essential role in the uptake of liposomes by urothelium (Fig. 6).107 Liposome mediated drug delivery can be endocytosed to the urothelial cell interior.106 Thus, IDD of therapeutic drugs encapsulated in liposomes suggests an attractive approach for the enhancement of therapeutic efficacy. In the following parts, some representative agents encapsulated into liposomes for IC/BPS treatment are introduced.
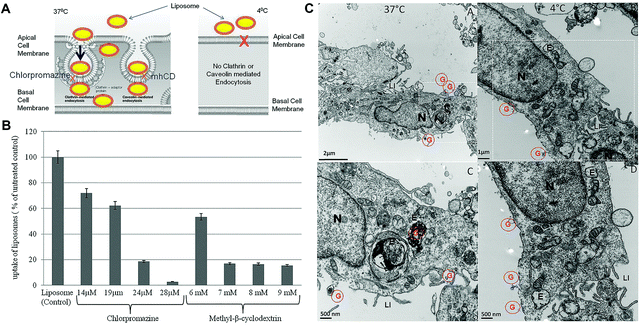 |
| Fig. 6 Bladder uptake of liposomes after intravesical administration occurs by endocytosis. (A) Schematic illustration of temperature dependent uptake of liposomes. (B) Effect of different endocytic inhibitors on cellular uptake of liposomes. (C) Transmission electron microscopy (TEM) micrographs showing endocytosis mediated uptake of the liposome encapsulated gold marker at 37 °C and 4 °C. Red colored G: gold encapsulated in liposomes. N: nucleus. E: endocytic vesicles. LI: finger-like projections called as lateral interdigitation. Reproduced with permission.107 Copyright 2015, PLOS. | |
4.1.1 Liposomes for botulinum toxin delivery.
According to the European Society for the Study of IC/PBS, pelvic pain is the hallmark symptom for IC/BPS.108 The mechanism of pelvic pain involves various neurotransmitters.109 Botulinum toxin (BoNT), which is produced by Clostridium botulinum with a molecular weight of 150 kDa, inhibits the release of neurotransmitters such as ACh, ATP, and SP. BoNT serotype A (BoNT-A) is the most common serotype of BoNT.109,110 After the injection of BoNT-A into the bladder wall, the release of neurotransmitters including calcitonin, CGRP, glutamate, ATP and SP from neurons was blocked (Fig. 7).109 Cystoscopic injection of BoNT-A has been listed as the fourth-line therapy of IC/BPS by AUA guideline.12 Nevertheless, the cystoscopic injection is a painful and invasive procedure with the risks of infection and hemorrhage.110 Thus, IDD of BoNT-A is worth exploring as an alternative IC/BPS treatment. However, the high molecular weight of BoNT-A limits urothelium penetration. Therefore, based on the carrier capacity of liposomes, liposome-encapsulated BoNT-A (lipotoxin) was applied to IC/BPS treatment to improve mucosal penetration. In a preclinical study, lipotoxin was prepared via a modified dehydration–rehydration vesicle method and administered into the bladder in a cystitis rat model induced by acetic acid.96 The lipotoxin treatment group showed significant decreases in the continuous cystometrogram parameters. More importantly, the inflammatory reaction was alleviated, and the expression levels of SNAP-25 and CGRP were down-regulated in the lipotoxin treatment group. It is suggested that liposomes could deliver BoNT-A through epithelium to improve the treatment effect (Fig. 7). In a later clinical study, lipotoxin (200 U onabotulinumtoxinA plus 80 mg sphingomyelin) was instilled into the bladder of IC/BPS patients.111 In the lipotoxin treatment group, O’Leary-Sant symptom scores (OSS) and visual analog scale (VAS) of pain scale were significantly decreased compared to the baseline.
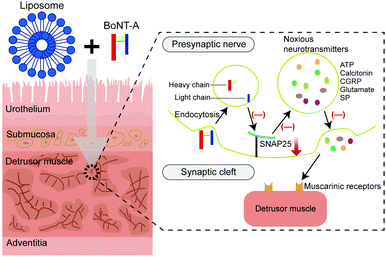 |
| Fig. 7 Mechanisms of the BoNT-A encapsulated liposome for IC/BPS treatment. BoNT-A cleaves the SNAP-25 protein and blocks the release of noxious neurotransmitters that activate the muscarinic receptors leading to muscle pain. ATP: adenosine triphosphate. CGRP: calcitonin gene-related peptide. SP: substance P. | |
4.1.2 Liposomes for tacrolimus delivery.
Tacrolimus can inhibit the secretion of proinflammatory cytokines in the T immune cells.112,113 However, systemic administration of tacrolimus leads to side effects such as nephrotoxicity and hypertension.114 Moreover, the low water solubility of tacrolimus limits its intravesical delivery for IC/BPS treatment.98,114 In a recent clinical trial, tacrolimus dissolved in 50% dimethyl sulfoxide (DMSO) was instilled into the bladder of 24 IC/BPS patients. Symptoms were relieved in 13 patients, but the toxicity of DMSO may hinder the further translation of this treating method.115 To overcome this problem, Nirmal et al. used liposomes to encapsulate tacrolimus for bladder delivery in a cystitis rat.98 The author compared the pharmacokinetics of intravesical instillation of liposome-tacrolimus with an intraperitoneal injection of plain tacrolimus. The data indicated that the tacrolimus serum level in the intravesical instillation group was significantly lower than that in the intraperitoneal counterpart. More importantly, the accumulative level of tacrolimus in the bladder was not significantly different within the two groups. Taken together, these results illustrate that liposome formulation is effective in alleviating the systemic side-effects of tacrolimus in IC/BPS treatment.
4.1.3 Liposomes for oligonucleotide delivery.
Recent clinical evidence revealed the pathological synthesis and release conditions of NGF in the urothelium of IC/BPS patients, and the strategy of down-regulation of NGF has been investigated for IC/BPS treatment.84,101 However, direct instillation of the NGF antisense oligonucleotide (OND) into the bladder is subject to rapid deactivation. Liposomes loaded with nucleic acids potentially increase the uptake of the urothelium for combating IC/BPS.13,99 In a cystitis rat model induced by hydrogen peroxide, OND-loaded liposomes effectively penetrate mucosal barriers to relieve hypersensitivity, thereby improving the therapeutic effect of IC/BPS.100
4.2 Nanoparticles
Nanoparticulate systems have been widely used for the treatment of bladder diseases owing to the advantages of their high cargo loading rate, controllable release kinetics and prolonged drug retention.116 For instance, chitosan-functionalized poly(lactic-co-glycolic acid) (PLGA) nanoparticles (NPs) were used to encapsulate small interfering RNA (siRNA) for improved cellular uptake and the therapeutic effect.116 Drugs can penetrate the bladder wall via the NP platform without structural damage to the urothelium.117,118 Strategies were proposed on constructing drug-laden nanoparticles for bladder instillation to improve IC/BPS treatment.
The BPB effect restricts therapeutic efficacy of the drug instilled into the bladder. It is essential to regulate mucoadhesion to enhance the penetration of the drug. The combination of chitosan and halloysite nanotubes (HNTs) was demonstrated to improve mucoadhesion and control drug release.119 Barthelmes et al. developed chitosan modified NPs for mucoadhesive sustained drug release.102 Compared to the unmodified chitosan NPs, chitosan-thioglycolic acid (chitosan-TGA) NPs exhibited better stability and superior mucoadhesion. Trimethoprim (TMP) was also incorporated in the chitosan-TGA NPs to improve the therapeutic outcome of IC/BPS. Specifically, the adhesion of chitosan-TGA NPs on the urinary bladder mucosa was 14-fold higher than the unmodified counterpart. Thus, chitosan-TGA NPs act as the enhanced nanoparticulate platform for bladder delivery with the controlled and prolonged drug-releasing profile. The improved therapeutic effect for IC/BPS was well achieved.
4.3 Hydrogel
Hydrogel-based drug delivery systems with prolonged drug retention in the bladder have been reported for IC/BPS treatment.1,14,104,120 A thermosensitive hydrogel presents a sol state at room temperature for easy injection and then converts into a gel state at body temperature, which extends the period of drug action to improve the therapeutic efficacy.14 For example, the misoprostol-loaded thermosensitive poly(ethylene glycol)–poly(lactic acid-co-glycolic acid)–poly(ethylene glycol) (PEG–PLGA–PEG) hydrogel was easily instilled into the bladder through PE-50 tubing to treat the CYP-induced cystitis rats.103 Fluorescein was encapsulated in PEG–PLGA–PEG to detect the kinetics of FITC excretion in the urine, demonstrating the prolonged drug retention time of the PEG–PLGA–PEG system in the bladder. In addition, in the misoprostol–PEG–PLGA–PEG treatment group, voiding frequency was significantly reduced, and bladder urothelium showed less-inflamed morphology based on histological examination. Consequently, PEG–PLGA–PEG is a promising drug delivery platform for IDD with sustained drug release to alleviate the symptoms of IC/BPS.
However, the thermosensitive hydrogel exhibits gel-forming properties at elevated temperature, and it poses a high risk of urinary obstruction. As a potential solution, Lin et al. developed Poloxamer 407 (P407) with many micro-bubbles that can float in urine to avoid urinary obstruction (Fig. 8).104 Micro-bubbles of P407 solution were produced by shaking with hand to avoid damage of hamper. Moreover, in the bladder of the PS-induced acute cystitis rabbit, heparin embedded P407, as a floating hydrogel platform with micro-bubbles, can extend the resident time in the bladder without urethral obstruction.
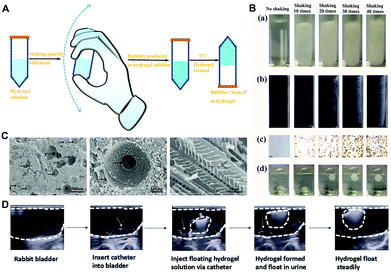 |
| Fig. 8 The characteristics of a floating hydrogel (Poloxamer 407). (A) Schematic illustration of bubble generation in Poloxamer 407. (B) Effect of bubble generation was recorded at different shaking times by (a) photos, (b) ultrasound image, (c) microscope image, and (d) photos of the floating state. (C) The floating hydrogel morphology under SEM. (D) Ultrasound image showing insertion of Poloxamer 407 into the rabbit bladder floating in urine without urinal obstruction. Reproduced with permission.104 Copyright 2016, MDPI. | |
Recently, Jensen et al. reported a temperature-responsive silk-elastin-like protein polymer (SELP) for IC/BPS treatment (Fig. 9).1 This study introduced four polymers including SELP 415K, SELP 815K, PLGA–PEG–PLGA and P407. Two kinds of SELP had a lower viscosity than P407 and a better-controlled drug release profile than PLGA–PEG–PLGA that fit the zero-order release. An in vivo experiment showed that semisynthetic glycosaminoglycan ethers (SAGE), a class of therapeutic GAGs with anti-inflammation and analgesic effects, was encapsulated in the four polymers for IDD in the cystitis mice model. The results showed that both SELP 415K and SELP 815K improved the SAGE accumulation in the bladder. Intravesical delivery of SAGE by SELP 415K exhibited the greatest therapeutic efficacy of analgesic and anti-inflammation effects.
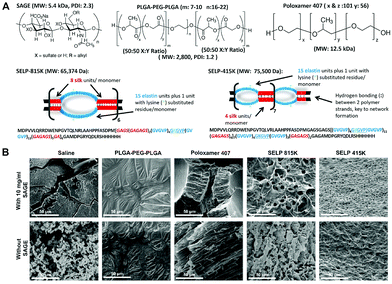 |
| Fig. 9 Structures and characteristics of SAGE and 4 types of temperature-responsive polymers. (A) Structures of SAGE, PLGA–PEG–PLGA, Poloxamer 407, SELP-815K and SELP-415K. (B) SEM images of lyophilized polymers, demonstrating SELP network formation. Reproduced with permission.1 Copyright 2019, Elsevier. | |
Furthermore, thermosensitive hydrogels have been applied in many clinical studies. TC-3 gel, an FDA approved biologically inactive ingredient, is a novel reverse-thermal hydrogel with the liquid state at approximately 5 °C and solidifies at body temperature. The BoNT-A encapsulated TC-3 gel can treat IC/BPS patients with a single injection in the patient bladder. The outcome of this clinical trial suggested sterling performance of hydrogel-based therapy in treating VAS, relieving interstitial cystitis symptoms and lowering interstitial cystitis problem index (ICPI) scores.14
5. Conclusion and outlook
Owing to the elusive pathogenesis of IC/BPS, the therapeutic efficacy is still dissatisfactory. Various animal models have been developed to investigate the potential aetiology of IC/BPS. For IC/BPS treatment, biomaterials have made significant progress in the past few decades, and they have been applied for intravesical drug delivery systems to extend the drug dwelling time in the bladder, increase mucosa penetration and reduce systemic side effects. The intravesical administration of drugs encapsulated in biomaterials has not yet been the main strategy for IC/BPS therapy. Fortunately, both animal studies and clinical trials have shown positive therapeutic effects in IC/BPS treatment. Thus, more biocompatible and functional biomaterials should be developed to improve the treatment of IC/BPS and facilitate clinical translation.
Recently, mesenchymal stem cell (MSC) therapy has attracted significant attention in various diseases and disorders thanks to its superior ability to self-renew and differentiate into multiple cell lineages. To date, MSC therapies have been applied to IC/BPS treatment through systemic administration or direct injection in the bladder wall, but it is limited by the short viability of MSCs. Biocompatible scaffolds, including natural, synthetic and conductive polymers, have also been employed in tissue engineering. Thus, biomaterials that encapsulate MSCs, for cellular transplantation, could be proposed for IC/BPS treatment and regeneration of the damaged bladder. In addition, MSCs have been characterized for paracrine potency of bioactive molecules, also known as secretome, in the surrounding conditioned media (CM). MSC-derived secretome can improve the treatment for various diseases with the effect on the promotion of tissue generation. Taken together, the synergic effect of cell or cell-free therapy and biomaterial-based nanoplatforms could enhance the transport of the therapeutic agents and treatment efficiency.
In the future, more studies of biomaterial-assisted IDD for IC/BPS treatment should be explored. Existing biomaterials can be further optimized for translation, and novel biomaterial-based IDD systems are expected to improve the therapeutic effect in IC/BPS.
Conflicts of interest
There are no conflicts to declare.
Acknowledgements
This work was supported by the National Key Research and Development Program of China (2019YFA0111300), the Guangdong Province Science and Technology Innovation Special Fund (International Scientific Cooperation, 2018A050506035), the National Natural Science Foundation of China (51903256, 21907113, 81670688, and 81800666), the Guangdong Province Natural Science Foundation of China (2016A030313192, 2017A030310414, and 2018A0303130330), and the Fundamental Research Funds for the Central Universities (19ykpy33).
Notes and references
- M. M. Jensen, W. Jia, A. J. Schults, K. J. Isaacson, D. Steinhauff, B. Green, B. Zachary, J. Cappello, H. Ghandehari and S. Oottamasathien, Biomaterials, 2019, 217, 119293 CrossRef CAS.
- F. G. Almeida, N. Batezini, R. S. Simões and W. M. Bernardo, Rev. Assoc. Med. Bras., 1992, 2019(65), 535–540 Search PubMed.
- Y. Akiyama and P. Hanno, Int. J. Urol., 2019, 26, 17–19 CrossRef.
- T. Ogawa, O. Ishizuka, T. Ueda, P. Tyagi, M. B. Chancellor and N. Yoshimura, Expert Rev. Clin. Pharmacol., 2018, 11, 495–505 CrossRef CAS.
- J. Q. Clemens, C. Mullins, A. L. Ackerman, T. Bavendam, A. van Bokhoven, B. M. Ellingson, S. E. Harte, J. J. Kutch, H. H. Lai, K. T. Martucci, R. Moldwin, B. D. Naliboff, M. A. Pontari, S. Sutcliffe and J. R. Landis, Nat. Rev. Urol., 2019, 16, 187–200 CrossRef.
- J. J. J. Wyndaele, C. Riedl, R. Taneja, S. Lovasz, T. Ueda and M. Cervigni, Neurourol. Urodyn., 2019, 38, 535–544 CrossRef.
- S. S. Patnaik, A. S. Lagana, S. G. Vitale, S. Buttice, M. Noventa, S. Gizzo, G. Valenti, A. M. C. Rapisarda, V. L. La Rosa, C. Magno, O. Triolo and V. Dandolu, Arch. Gynecol. Obstet., 2017, 295, 1341–1359 CrossRef CAS.
- J. L. Chen, X. Zhou, H. L. Ding, H. L. Zhan, F. Yang, W. B. Li, J. C. Xie, X. G. Liu, Y. C. Xu, M. Z. Su, B. L. Liu and X. F. Zhou, Neurourol. Urodyn., 2019, 38, 1250–1260 CrossRef CAS.
- S. T. Malik, B. R. Birch, D. Voegeli, M. Fader, V. Foria, A. J. Cooper, A. F. Walls and B. A. Lwaleed, J. Clin. Pathol., 2018, 71, 840–844 CrossRef.
- L. H. Tseng, Expert Opin. Drug Discovery, 2014, 9, 423–432 CrossRef CAS.
- J. C. Nickel, S. Herschorn, K. E. Whitmore, J. B. Forrest, P. Hu, A. J. Friedman and A. S. Baseman, J. Urol., 2015, 193, 857–862 CrossRef CAS.
- P. M. Hanno, D. Erickson, R. Moldwin and M. M. Faraday, J. Urol., 2015, 193, 1545–1553 CrossRef.
- P. Tyagi, M. Kashyap, T. Majima, N. Kawamorita, T. Yoshizawa and N. Yoshimura, Int. J. Urol., 2017, 24, 262–271 CrossRef CAS.
- Y. H. Rappaport, A. Zisman, M. Jeshurun-Gutshtat, T. Gerassi, G. Hakim, Y. Vinshtok and K. Stav, Urology, 2018, 114, 60–65 CrossRef.
- S. GuhaSarkar and R. Banerjee, J. Controlled Release, 2010, 148, 147–159 CrossRef CAS.
- D. T. Martin, C. J. Hoimes, H. Z. Kaimakliotis, C. J. Cheng, K. Zhang, J. Liu, M. A. Wheeler, W. K. Kelly, G. N. Tew, W. M. Saltzman and R. M. Weiss, Nanomedicine, 2013, 9, 1124–1134 CrossRef CAS.
- Z. Fang and K. Xu, Curr. Bladder Dysfunct. Rep., 2016, 11, 391–398 CrossRef.
- L. A. Birder, Int. J. Urol., 2019, 26, 12–15 CrossRef.
- B. L. Liu, F. Yang, H. L. Zhan, Z. Y. Feng, Z. G. Zhang, W. B. Li and X. F. Zhou, Urol. Int., 2014, 92, 202–208 CrossRef CAS.
- K. Monastyrskaya, V. Sanchez-Freire, A. Hashemi Gheinani, D. J. Klumpp, E. B. Babiychuk, A. Draeger and F. C. Burkhard, Am. J. Pathol., 2013, 182, 431–448 CrossRef CAS.
- N. Montalbetti, A. C. Rued, S. N. Taiclet, L. A. Birder, F. A. Kullmann and M. D. Carattino, eNeuro, 2017, 4, ENEURO.0381-16.2017 CrossRef.
- P. J. Hauser, S. B. VanGordon, J. Seavey, T. M. Sofinowski, M. Ramadan, S. Abdullah, C. A. Buffington and R. E. Hurst, J. Urol., 2015, 194, 571–577 CrossRef CAS.
- P. J. Hauser, M. G. Dozmorov, B. L. Bane, G. Slobodov, D. J. Culkin and R. E. Hurst, J. Urol., 2008, 179, 764–769 CrossRef CAS.
- N. Montalbetti, J. G. Rooney, A. C. Rued and M. D. Carattino, J. Neurophysiol., 2019, 122, 1136–1146 CrossRef CAS.
- J. F. Jhang, H. C. Ho, Y. H. Jiang, C. L. Lee, Y. H. Hsu and H. C. Kuo, PLoS One, 2018, 13, e0198816 CrossRef.
- N. Montalbetti, A. C. Rued, D. R. Clayton, W. G. Ruiz, S. I. Bastacky, H. S. Prakasam, A. F. Eaton, F. A. Kullmann, G. Apodaca and M. D. Carattino, Am. J. Physiol.: Renal. Physiol., 2015, 309, F1070–F1081 CrossRef CAS.
- M. Cervigni, Transl. Androl. Urol., 2015, 4, 638–642 Search PubMed.
- D. A. W. Janssen, X. M. R. v. Wijk, K. C. F. J. Jansen, T. H. v. Kuppevelt, J. P. F. A. Heesakkers and J. A. Schalken, J. Urol., 2013, 189, 336–342 CrossRef CAS.
- M. Cervigni, F. Natale, L. Nasta and A. Mako, Int. Urogynecol. J., 2012, 23, 1187–1192 CrossRef CAS.
- A. Stellavato, A. V. A. Pirozzi, P. Diana, S. Reale, V. Vassallo, A. Fusco, G. Donnarumma, M. De Rosa and C. Schiraldi, PLoS One, 2019, 14, e0218475 CrossRef CAS.
- J. Xie, B. Liu, J. Chen, Y. Xu, H. Zhan, F. Yang, W. Li and X. Zhou, Biochem. Biophys. Res. Commun., 2018, 495, 546–552 CrossRef CAS.
- R. Weiskirchen, S. K. Meurer, C. Liedtke and M. Huber, Cells, 2019, 8, 1429 CrossRef CAS.
- P. Conti, C. E. Gallenga, A. Caraffa, G. Ronconi and S. K. Kritas, Dermatol. Ther., 2020, 33, e13191 CrossRef.
- H. Shan, E. W. Zhang, P. Zhang, X. D. Zhang, N. Zhang, P. Du and Y. Yang, BMC Urol., 2019, 19, 115 CrossRef.
- K. Gupta and I. T. Harvima, Immunol. Rev., 2018, 282, 168–187 CrossRef CAS.
- W. I. Mohamaden, R. Hamad and H. I. Bahr, Pak. Vet. J., 2019, 39, 151–156 CrossRef CAS.
- X. Wang, W. Liu, M. O’Donnell, S. Lutgendorf, C. Bradley, A. Schrepf, L. Liu, K. Kreder and Y. Luo, PLoS One, 2016, 11, e0168772 CrossRef.
- X. W. Jin, B. K. Liu, X. Zhang, Z. H. Zhao and Y. Shao, Inflammation, 2017, 40, 861–870 CrossRef CAS.
- D. Liang, J. Lu and A. Guo, Clin. Rheumatol., 2014, 33, 1189–1193 CrossRef.
- J. Y. Wen, T. S. Lo, Y. C. Chuang, C. H. Ho, C. Y. Long, K. S. Law, Y. C. Tong and M. P. Wu, Int. J. Urol., 2019, 26, 897–902 CrossRef.
- J. J. Keller, S. P. Liu and H. C. Lin, Neurourol. Urodyn., 2013, 32, 980–985 CrossRef.
- S. Keay, C. O. Zhang, A. L. Trifillis, J. R. Hebel, S. C. Jacobs and J. W. Warren, J. Urol., 1997, 157, 1083–1087 CrossRef CAS.
- K. Izgi, C. Z. Altuntas, F. Bicer, A. Ozer, C. Sakalar, X. Li, V. K. Tuohy and F. Daneshgari, PLoS One, 2013, 8, e72067 CrossRef CAS.
- I. Mykoniatis, I. Katafigiotis, S. Sfoungaristos and V. Yutkin, Expert Opin. Biol. Ther., 2017, 17, 1471–1480 CrossRef.
- Y. Logadottir, D. Delbro, C. Lindholm, M. Fall and R. Peeker, Int. J. Urol., 2014, 21, 75–78 CrossRef CAS.
- L. Zhang, A. U. Ihsan, Y. Cao, F. U. Khan, Y. Cheng, L. Han and X. Zhou, Inflammation, 2017, 40, 2033–2041 CrossRef CAS.
- P. C. Bosch, J. Urol., 2014, 191, 77–82 CrossRef CAS.
- P. C. Bosch, Eur. Urol., 2018, 74, 623–630 CrossRef CAS.
- K. M. Hellman, I. Y. Patanwala, K. E. Pozolo and F. F. Tu, Am. J. Obstet. Gynecol., 2015, 213, 827.e1–827.e9 CrossRef.
- L. A. Birder and F. A. Kullmann, Semin. Immunopathol., 2018, 40, 261–279 CrossRef.
- L. Birder and K. E. Andersson, Physiol. Rev., 2013, 93, 653–680 CrossRef CAS.
- B. Liu, M. Su, S. Tang, X. Zhou, H. Zhan, F. Yang, W. Li, T. Li and J. Xie, Mol. Pain, 2016, 12, 1–12 CAS.
- R. R. Ji, A. Nackley, Y. Huh, N. Terrando and W. Maixner, Anesthesiology, 2018, 129, 343–366 CrossRef.
- M. Cervigni, E. Onesti, M. Ceccanti, M. C. Gori, G. Tartaglia, G. Campagna, G. Panico, L. Vacca, C. Cambieri, L. Libonati and M. Inghilleri, Neurourol. Urodyn., 2018, 37, 2678–2687 CrossRef CAS.
- K. B. Tudrej, T. Piecha and M. Kozlowska-Wojciechowska, Ther. Adv. Urol., 2019, 11, 1–10 Search PubMed.
- H. H. Chang, J. C. Yeh, J. Mao, D. A. Ginsberg, G. Ghoniem and L. V. Rodriguez, Neurourol. Urodyn., 2019, 38, 116–122 CrossRef CAS.
- J. Li, H. Luo, X. Dong, Q. Liu, C. Wu, T. Zhang, X. Hu, Y. Zhang, B. Song and L. Li, Stem Cell Res. Ther., 2017, 8, 107 CrossRef.
- W. C. Lee, Y. L. Tain, Y. C. Chuang, C. N. Tsai, C. C. Yu and C. H. Su, Neurourol. Urodyn., 2019, 38, 2159–2169 CrossRef CAS.
- W. Yang, R. E. Yaggie, M. C. Jiang, C. N. Rudick, J. Done, C. J. Heckman, J. M. Rosen, A. J. Schaeffer and D. J. Klumpp, Am. J. Physiol.: Regul., Integr. Comp. Physiol., 2018, 314, R353–R365 CrossRef.
- W. A. Kwon, Int. Neurourol. J., 2018, 22, S1–S2 CrossRef.
- A. Coelho, A. S. Wolf-Johnston, S. Shinde, C. D. Cruz, F. Cruz, A. Avelino and L. A. Birder, Br. J. Pharmacol., 2015, 172, 1691–1699 CrossRef CAS.
- Q. Liu, B. Sun, J. Zhao, Q. Wang, F. An, X. Hu, Z. Yang, J. Xu, M. Tan and L. Li, Exp. Mol. Med., 2018, 50, 1–16 CrossRef.
- Q. Liu, Z. Long, X. Dong, T. Zhang, J. Zhao, B. Sun, J. Zhu, J. Li, Q. Wang, Z. Yang, X. Hu and L. Li, Exp. Mol. Med., 2017, 49, e319 CrossRef CAS.
- M. Boudes, P. Uvin, S. Kerselaers, R. Vennekens, T. Voets and D. De Ridder, Neurourol. Urodyn., 2011, 30, 1659–1665 CrossRef.
- A. V. Golubeva, A. V. Zhdanov, G. Mallel, T. G. Dinan and J. F. Cryan, Physiol. Rep., 2014, 2, e00260 CrossRef.
- A. Wróbel, Ł. Zapała, P. Zapała, T. Piecha and P. Radziszewski, Eur. J. Pharmacol., 2020, 882, 173321 CrossRef.
- M. Yilmaz, T. Cakmak, A. Yenilmez, B. Baseskioglu and S. Metin, Undersea Hyperbaric Med., 2016, 43, 181–188 Search PubMed.
- N. Kawamorita, S. Yoshikawa, M. Kashyap, P. Tyagi, Y. Arai, M. B. Chancellor and N. Yoshimura, J. Urol., 2016, 195, 1920–1926 CrossRef CAS.
- J. M. Rosen and D. J. Klumpp, Int. J. Urol., 2014, 21, 26–32 CrossRef.
- B. H. Choi, L. H. Jin, K. H. Kim, J. Y. Han, J. H. Kang, S. M. Yoon, C. S. Park and T. Lee, Mol. Med. Rep., 2014, 10, 670–676 CrossRef CAS.
- G. Berger, N. Arora, I. Burkovskiy, Y. Xia, A. Chinnadurai, R. Westhofen, G. Hagn, A. Cox, M. Kelly, J. Zhou and C. Lehmann, Molecules, 2019, 24, 4239 CrossRef CAS.
- Q. Liu, Z. Wu, Y. Liu, L. Chen, H. Zhao, H. Guo, K. Zhu, W. Wang, S. Chen, N. Zhou, Y. Li and B. Shi, Neurourol. Urodyn., 2020, 39, 158–169 CrossRef CAS.
- J. C. Yeh, R. Do, H. Choi, C. T. Lin, J. J. Chen, X. Zi, H. H. Chang and G. Ghoniem, Int. Urol. Nephrol., 2019, 51, 53–59 CrossRef CAS.
- C. P. Huang, C. C. Chen, Y. T. Tsai, C. C. Wu and C. R. Shyr, Cell Transplant., 2019, 28, 296–305 Search PubMed.
- C. M. Ryu, J. H. Shin, H. Y. Yu, H. Ju, S. Kim, J. Lim, J. Heo, S. Lee, D. M. Shin and M. S. Choo, Sci. Rep., 2019, 9, 8134 CrossRef.
- J. Kamei, N. Aizawa, T. Nakagawa, S. Kaneko, H. Kume, Y. Homma and Y. Igawa, Sci. Rep., 2018, 8, 15622 CrossRef.
- H. Li, Z. Zhang, J. Peng, Z. Xin, M. Li, B. Yang, D. Fang, Y. Tang and Y. Guo, Investig. Clin. Urol., 2019, 60, 359–366 CrossRef.
- M. A. Matuszewski, K. Tupikowski, L. Dolowy, B. Szymanska, J. Dembowski and R. Zdrojowy, Cent. Eur. J. Urol., 2016, 69, 252–257 CAS.
- B. K. Liu, X. W. Jin, H. Z. Lu, X. Zhang, Z. H. Zhao and Y. Shao, Inflammation, 2019, 42, 246–254 CrossRef CAS.
- U. P. Singh, N. P. Singh, H. Guan, V. L. Hegde, R. L. Price, D. D. Taub, M. K. Mishra, M. Nagarkatti and P. S. Nagarkatti, PLoS One, 2013, 8, e79751 CrossRef.
- C. Z. Altuntas, F. Daneshgari, C. Sakalar, E. Goksoy, M. F. Gulen, M. Kavran, J. Qin, X. Li and V. K. Tuohy, Eur. Urol., 2012, 61, 193–200 CrossRef CAS.
- M. T. Sanford, J. C. Yeh, J. J. Mao, Y. Guo, Z. Wang, R. Zhang, D. P. Holschneider and L. V. Rodriguez, Neurourol. Urodyn., 2020, 39, 603–612 CrossRef.
- F. A. Kullmann, B. M. McDonnell, A. S. Wolf-Johnston, A. J. Kanai, S. Shiva, T. Chelimsky, L. Rodriguez and L. A. Birder, Neurourol. Urodyn., 2019, 38, 572–581 CrossRef CAS.
- B. Dias, P. Serrao, F. Cruz and A. Charrua, Sci. Rep., 2019, 9, 14113 CrossRef.
- Z. Wang, H. H. Chang, Y. Gao, R. Zhang, Y. Guo, D. P. Holschneider and L. V. Rodriguez, PLoS One, 2017, 12, e0182976 CrossRef.
- W. Yang, C. N. Rudick, E. Hoxha, S. A. Allsop, J. D. Dimitrakoff and D. J. Klumpp, Am. J. Physiol.: Renal. Physiol., 2012, 303, F350–F356 CrossRef CAS.
- C. N. Rudick, V. I. Pavlov, M. C. Chen and D. J. Klumpp, J. Urol., 2012, 187, 715–724 CrossRef.
- C. N. Rudick, A. J. Schaeffer and D. J. Klumpp, BMC Urol., 2009, 9, 16 CrossRef.
- C. N. Rudick, P. J. Bryce, L. A. Guichelaar, R. E. Berry and D. J. Klumpp, PLoS One, 2008, 3, e2096 CrossRef.
- C. N. Rudick, M.
C. Chen, A. K. Mongiu and D. J. Klumpp, Am. J. Physiol.: Regul., Integr. Comp. Physiol., 2007, 293, R1191–R1198 CrossRef CAS.
- F. Bicer, C. Z. Altuntas, K. Izgi, A. Ozer, M. Kavran, V. K. Tuohy and F. Daneshgari, Am. J. Physiol.: Renal. Physiol., 2015, 308, F103–F113 CrossRef CAS.
- B. D. Naliboff, A. J. Stephens, N. Afari, H. Lai, J. N. Krieger, B. Hong, S. Lutgendorf, E. Strachan and D. Williams, Urology, 2015, 85, 1319–1327 CrossRef.
- A. L. Smith, J. Leung, S. Kun, R. Zhang, I. Karagiannides, S. Raz, U. Lee, V. Glovatscka, C. Pothoulakis, S. Bradesi, E. A. Mayer and L. V. Rodriguez, Urology, 2011, 78, 967 CrossRef.
- U. J. Lee, A. L. Ackerman, A. Wu, R. Zhang, J. Leung, S. Bradesi, E. A. Mayer and L. V. Rodriguez, Physiol. Behav., 2015, 139, 541–548 CrossRef CAS.
- K. Yamamoto, T. Takao, J. Nakayama, H. Kiuchi, H. Okuda, S. Fukuhara, I. Yoshioka, Y. Matsuoka, Y. Miyagawa, A. Tsujimura and N. Nonomura, Int. J. Urol., 2012, 19, 155–162 CrossRef CAS.
- Y. C. Chuang, P. Tyagi, C. C. Huang, N. Yoshimura, M. Wu, J. Kaufman and M. B. Chancellor, J. Urol., 2009, 182, 786–792 CrossRef CAS.
- Y. C. Chuang, P. Tyagi, H. Y. Huang, N. Yoshimura, M. Wu, J. Kaufman and M. B. Chancellor, Neurourol. Urodyn., 2011, 30, 421–427 CrossRef CAS.
- J. Nirmal, P. Tyagi, M. B. Chancellor, J. Kaufman, M. Anthony, D. D. Chancellor, Y. T. Chen and Y. C. Chuang, J. Urol., 2013, 189, 1553–1558 CrossRef CAS.
- M. Kashyap, N. Kawamorita, V. Tyagi, Y. Sugino, M. Chancellor, N. Yoshimura and P. Tyagi, J. Urol., 2013, 190, 757–764 CrossRef CAS.
- T. Majima, P. Tyagi, K. Dogishi, M. Kashyap, Y. Funahashi, M. Gotoh, M. B. Chancellor and N. Yoshimura, Hum. Gene Ther., 2017, 28, 598–609 CrossRef CAS.
- J. M. Beckel, S. L. Daugherty, P. Tyagi, A. S. Wolf-Johnston, L. A. Birder, C. H. Mitchell and W. C. de Groat, J. Physiol., 2015, 593, 1857–1871 CrossRef CAS.
- J. Barthelmes, G. Perera, J. Hombach, S. Dunnhaupt and A. Bernkop-Schnurch, Int. J. Pharm., 2011, 416, 339–345 CrossRef CAS.
- P. Tyagi, Z. Li, M. Chancellor, W. C. De Groat, N. Yoshimura and L. Huang, Pharm. Res., 2004, 21, 832–837 CrossRef CAS.
- T. Lin, X. Zhao, Y. Zhang, H. Lian, J. Zhuang, Q. Zhang, W. Chen, W. Wang, G. Liu, S. Guo, J. Wu, Y. Hu and H. Guo, Materials, 2016, 9, 1005 CrossRef.
- P. Tyagi, M. Kashyap, H. Hensley and N. Yoshimura, Expert Opin. Drug Delivery, 2016, 13, 71–84 CrossRef CAS.
- K. M. Peters, D. Hasenau, K. A. Killinger, M. B. Chancellor, M. Anthony and J. Kaufman, Int. Urol. Nephrol., 2014, 46, 2291–2295 CrossRef CAS.
- B. R. Rajaganapathy, M. B. Chancellor, J. Nirmal, L. Dang and P. Tyagi, PLoS One, 2015, 10, e0122766 CrossRef.
- D. S. Engeler, A. P. Baranowski, P. Dinis-Oliveira, S. Elneil, J. Hughes, E. J. Messelink, A. van Ophoven and A. C. Williams, Eur. Urol., 2013, 64, 431–439 CrossRef.
- J. F. Jhang and H. C. Kuo, Toxins, 2018, 10, 260 CrossRef.
- D. Eldred-Evans and P. Dasgupta, Transl. Androl. Urol., 2017, 6, 234–251 CrossRef.
- Y. C. Chuang and H. C. Kuo, J. Urol., 2017, 198, 376–382 CrossRef CAS.
- J. C. Gardenier, R. P. Kataru, G. E. Hespe, I. L. Savetsky, J. S. Torrisi, G. D. Nores, D. K. Jowhar, M. D. Nitti, R. C. Schofield, D. C. Carlow and B. J. Mehrara, Nat. Commun., 2017, 8, 14345 CrossRef CAS.
- J. C. Gardenier, J. S. Torrisi, I. L. Savetsky, G. D. G. Nores, D. K. Jowhar, G. E. Hespe, M. D. Nitti, R. P. Kataru and B. J. Mehrara, J. Am. Coll. Surg., 2015, 221, S120 CrossRef.
- B. R. Rajaganapathy, J. J. Janicki, P. Levanovich, P. Tyagi, J. Hafron, M. B. Chancellor, S. Krueger and B. Marples, J. Urol., 2015, 194, 578–584 CrossRef CAS.
- N. N. Mishra, C. Riedl, S. Shah and N. Pathak, Int. J. Urol., 2019, 26, 68–72 CrossRef CAS.
- D. T. Martin, J. M. Steinbach, J. Liu, S. Shimizu, H. Z. Kaimakliotis, M. A. Wheeler, A. B. Hittelman, W. Mark Saltzman and R. M. Weiss, Mol. Cancer Ther., 2014, 13, 71–81 CrossRef CAS.
- C. C. Hsu, Y. C. Chuang and M. B. Chancellor, Int. J. Urol., 2013, 20, 552–562 CrossRef CAS.
- L.-C. Chang, S.-C. Wu, J.-W. Tsai, T.-J. Yu and T.-R. Tsai, Int. J. Pharm., 2009, 376, 195–203 CrossRef CAS.
- S. Sharif, G. Abbas, M. Hanif, A. Bernkop-Schnürch, A. Jalil and M. Yaqoob, Colloids Surf., B, 2019, 184, 110527 CrossRef CAS.
- J. Krhut, M. Navratilova, R. Sykora, M. Jurakova, M. Gartner, D. Mika, L. Pavliska and P. Zvara, Scand. J. Urol., 2016, 50, 200–205 CrossRef CAS.
Footnote |
† These authors contributed equally to this work. |
|
This journal is © The Royal Society of Chemistry 2021 |