Superior antibacterial activity of gallium based liquid metals due to Ga3+ induced intracellular ROS generation†
Received
18th January 2020
, Accepted 16th September 2020
First published on 17th September 2020
Abstract
Gallium-based liquid metals have increasing applications in a wide variety of emerging areas and they are involved more in frontier studies, the energy industry and additive manufacturing production, and even in daily life. When exposed to open air, large amounts of microorganisms may interact with liquid metals. However, the research of the relationship between pure gallium-based liquid metals and bacterial cells is still limited. In this study, the antibacterial properties of eutectic gallium–indium (EGaIn) alloys were tested against the typical Gram-negative bacteria—Escherichia coli and the Gram-positive bacteria—Staphylococcus aureus and the experimental results displayed that the antibacterial rates reached 100%. We also explored the mechanism of the anti-bacterial properties of EGaIn alloys by measuring the surface composition of the EGaIn film and the concentration of dissolved metal ions. The morphology of the bacterial cells showed that the cell growth and division were influenced by exposure to EGaIn. We also found that the synergistic antibacterial effect came along with the production of reactive oxygen species (ROS). Moreover, the EGaIn film showed enhanced antibacterial activity compared to gallium nitrate at the same initial ion concentration in the solution. This study shows the enormous potential of the anti-bacterial effect of liquid metals.
1. Introduction
Gallium-based liquid metals (LMs), such as eutectic gallium–indium alloys (EGaIn) and gallium–indium–tin alloys (Galinstan), show excellent prospects as highly versatile materials.1 The use of intrinsically liquid-state metals with superior thermal and electrical conductivity, low viscosity, favorable flexibility, and biocompatibility has been considered as an ideal solution to problems encountered in diverse fields such as printed electronics,2–8 soft robots, biomedicine,1,9–11 and energy management.12–23 Among various applications, LMs have shown great practical value in the field of printed electronics, which is also called additive manufacturing. Compared to subtractive manufacturing, LM based printed electronics can achieve one-step printing in a few minutes and large-scale production, which provides an innovative way for electronic field and fast manufacturing. Recently, Gao et al. proposed a method to directly writing circuit on various substrates using liquid metal ink.24 And Zheng et al. developed the world's first-ever liquid metal printer to fabricate complex circuits, which has been used in practical operation.25 Guo et al. proposed LM transfer printing technology, which suggests a universal fabrication of flexible electronics for fast production of complicated and multilayer circuits.26 LM printed electronics exhibit advantages that other conductive inks do not possess, such as high conductivity, deformability, robustness, softness, and stretchability. Because of this, the stability of the LM circuit in open air is particularly important. As a matter of fact, the anti-bacterial performance of bulk LMs has rarely been reported and investigated. On the other hand, the widespread use of gallium-based LMs in research and industrial applications has led to their release into the environment at elevated concentrations with potential effects on living organisms and environmental health. Bacteria are the most abundant of all organisms; however, the interaction between pure gallium-based LMs and bacteria is still poorly studied.
One of the main LMs with melting point around room temperature is gallium. The main purpose of adding other metals, such as indium or tin, is to further reduce its melting point. It is worth noting that the anti-bacterial activity of gallium (Ga) ions or gallium-based nanocomposites has been well investigated. For example, Kurtjak et al. developed a biocompatible nanocomposite (Ga@HAp) containing antibacterial nanospheres of Ga (16 wt%) and bioactive hydroxyapatite nanorods (HAp, 84 wt%).27 Adding Ga@HAp to bacterial culture dishes could sharply decrease the number of bacterial cells (P. aeruginosa in this case). The mainstream view is that Ga3+ ions released from nanoparticles can inhibit the bacterial growth or kill the bacteria. Choi et al. synthesized six different types of Ga3+ ions and rifampin nanoparticles to inhibit the growth of virulent Mycobacterium tuberculosis.28 Moreover, Ga3+ ion-based compounds also illustrated their potent inhibitory activity on the growth of both Mycobacteria and HIV in co-infected human macrophages.29 Overall, there are a number of direct and indirect proofs suggesting the critical role of Ga3+ ions as an antimicrobial agent.30,31 However, a reasonable and systematic explanation for the antibacterial mechanism of Ga is still limited. What can be determined is the critical role of Ga3+ ions, but whether Ga2O3 or other factors (such as reactive products or surface roughness) are also effective against bacterial growth should be further investigated.
This study aimed to assess the antibacterial ability of EGaIn for the typical Gram-negative bacteria—Escherichia coli and the Gram-positive bacteria—Staphylococcus aureus and explore the anti-bacterial mechanism. The results of the anti-bacterial test showed that the antibacterial rates reached 100%. Abnormal cell morphologies were observed by using a scanning electron microscope (SEM) and a transmission electron microscope (TEM). From abundant experimental evidence, we confirmed that LMs could generate reactive oxygen species (ROS) in bacteria through gallium ion substituting for iron and found that EGaIn films demonstrated enhanced antibacterial activity compared to gallium nitrate with the same gallium ion concentration. Continuously generated and substantial free Ga3+ ions play a vital role in the anti-bacterial behavior of EGaIn.
2. Material and methods
2.1 Bacterial culture
Two bacterial strains were used in this study, Gram-positive Staphylococcus aureus (ATCC 6538) and Gram-negative Escherichia coli (DH5α). The E. coli and S. aureus strains were kindly provided by Dr Yong Nie at Peking University and Dr Ye Tian at TIPC, respectively, and were maintained in our laboratory. Tryptone and yeast extract were purchased from Oxoid Ltd. NaCl and agar were purchased from Sinopharm Chemical Reagent Co. Ltd (China) and Becton Dickinson and Company, respectively. Strains were grown on LB agar plates at 37 °C for 12–16 h. Monoclonal colonies were inoculated into 15 ml centrifuge tubes containing 3 ml of LB medium and then grown on a shaker at 37 °C. Bacterial cultures were harvested at the exponential phase and the viable cells were calculated by quantifying the CFUs with the spread-plate method.
2.2 Fabrication of the GaIn alloy film and XPS surface analysis
Gallium with a purity of 99.99% and indium with a purity of 99.995% were purchased from Shanxi Zhaofeng Gallium Co. Ltd (China) and Zhuzhou Yilong New Materials Co. Ltd (China), respectively. Gallium and indium were weighted with a ratio of 75.5
:
24.5 and put in a beaker. The metals in the beaker were heated and then stirred together at 80 °C for 30 min to make the eutectic gallium–indium alloy (EGaIn)-GaIn24.5 (melting point: ∼15.5 °C).
EGaIn was poured into the container of the airbrush, atomized into microdroplets by airflow produced by 0.25 MPa nitrogen and sprayed uniformly on the surface of the polyvinyl chloride (PVC) plastic pieces (size of 2 cm × 2 cm) through a fluid nozzle (Fig. 1a). The distance between the PVC piece and the nozzle was about 10 cm and the spray time lasted for 3 s. The details of the atomization mechanism and the manipulation of the airbrush can be found in the previous studies of our group.32 The weight of EGaIn on each piece was 64.28 ± 5.42 mg and the average thickness of the EGaIn film was 25.31 ± 2.13 μm. Before the anti-bacterial test, the EGaIn pieces (Fig. 1b) were sterilized by exposing them to ultraviolet light for 1 h.
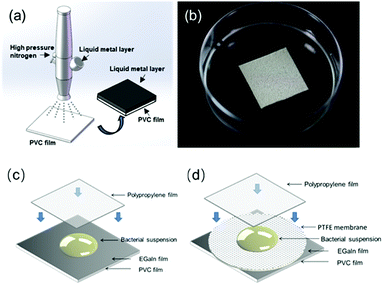 |
| Fig. 1 Fabrication of the EGaIn film. (a) Schematic diagram of spraying EGaIn on the PVC film through the airbrush. (b) Photo of the EGaIn covered PVC film. (c) Schematic diagram of anti-bacterial test on the EGaIn piece and (d) anti-bacterial test on the PTFE membrane separated EGaIn piece. | |
To determine the surface composition of the EGaIn covered PVC piece, we performed the X-ray photoelectron spectroscopy (XPS) surface analysis by using an electron spectrometer (ESCALab220i-XL, VG Scientific, UK) with 300 W Al-Kα radiation. The base pressure was about 3 × 10−9 mbar and the binding energies were calibrated using the C 1s peak at 284.6 eV from adventitious carbon.
2.3 Anti-bacterial tests
To evaluate the antibacterial properties of the EGaIn film, we performed the experiments based on the Japanese Industrial Standard (JIS) Z 2801:2010 test method.33 Bacterial culture was diluted to 2.5 × 105–10 × 105 CFU ml−1 with LB broth and used as inoculum. 100 μl of the inoculum was dropwise added onto the EGaIn pieces. Then the sterile polypropylene (PP) film (cut into a size of 1.6 cm × 1.6 cm) was carefully placed over the inoculum so that the inoculum can spread evenly on the metal surface (Fig. 1c). Then these pieces were placed in Petri dishes and put into the incubator at 37 °C with a saturated humidity environment.
To show the number of live bacterial cells on the EGaIn piece over time, the EGaIn pieces with bacterial cultures were washed with 2 ml of D-PBS at 2, 4, 6, 8, 10, 12, 16, 20, and 24 h, and the number of live bacterial cells in solution was determined by the spread-plate method. All experiments were carried out in triplicate and bare PVC pieces were used as the control group.
To determine whether the EGaIn surface is a contact-active antibacterial surface, we place a 0.4 μm pore PTFE membrane (the insert of the Transwell-6 well plates, Corning, Sigma-Aldrich) on the EGaIn piece (Fig. 1d) and performed the same anti-bacterial test as that for the EGaIn piece. For E. coli cells, 12 h of incubation was involved and for S. aureus cells, 20 h of incubation was involved.
To distinguish the antibacterial properties of the EGaIn film and gallium salts, we first measured the concentrations of the dissolved metal ions from EGaIn after 4, 8 and 24 h of incubation, respectively. Ion concentrations were measured by using a 710-ES inductively coupled plasma atomic emission spectrometer (ICP-AES, Varian, USA). Then we diluted the bacterial cells to 105 CFU ml−1 by Ga(NO3)3·xH2O (crystalline, 99.9% trace metals basis, Shanghai Macklin Biochemical Co Ltd, China) solutions, in which the measured concentrations of Ga ions were higher than that from EGaIn, and used as inoculum. 100 μl of the inoculum was added dropwise onto the PVC piece, covered by the PP film, incubated for 10 h, and washed with 2 ml of D-PBS. The number of live bacteria was determined by the spread-plate method.
2.4 SEM and TEM observation
The morphology of the bacterial cells after 12 h of exposure to EGaIn was observed by using an environmental scanning electron microscope (QUANTA 250 FEG, FEI Company, Hillsboro, OR, USA). Bacterial cells incubated on the PVC film were used as the controls. After being removed from the pieces by washing, bacterial cells were fixed in 2.5% glutaraldehyde (Beijing Leagene Biotech Co Ltd, China), washed and resuspended, and dehydrated with graded ethanol series of 50%, 70%, 80%, 90%, 95%, and 100% ethanol. Then the cells were added dropwise on a silicon wafer, air-dried and covered with a thin gold film by using an Ion Sputter Coater (MC1000, Hitachi, Japan) before taking images.
For the preparation of the samples for TEM, bacterial cells were fixed in 2.5% glutaraldehyde, 1% osmic acid, dehydrated with graded ethanol series of 30%, 50%, 70%, 85%, 95%, and 100% ethanol, and embedded in LR White resin (Sigma-Aldrich). Then the samples were sliced by using an ultramicrotome (Leica EM UC7, USA), placed on the grids with an ultra-thin carbon support film, and stained with uranyl acetate and lead citrate before imaging by using a JEM-1400 transmission electron microscope (JEOL Ltd, Japan).
2.5 Measurement of reactive oxygen species (ROS)
For in vitro detection of ROS, bacterial cells were diluted to 107 CFU ml−1 in D-PBS, stained by using 10 μM 2′-7′-dichlorofluorescein diacetate (DCFH-DA, λem = 530 nm, λex = 480 nm, Beijing Solarbio Science & Technology Co Ltd, China) at 37 °C for 25 min. 100 μl of the bacterial solution was added dropwise onto EGaIn and blank PVC pieces (control group), respectively. We also performed two groups of parallel experiments: (I) gallium nitrate was added to the stained bacterial solution with a concentration of 0.7 mM and then added dropwise onto the PVC piece to test whether ROS will generate in Ga(NO3)3·xH2O solution and (II) H2O2 was added to the stained bacterial solution with a concentration of 0.1 mM and then added dropwise onto the PVC piece, which acted as the positive control. After 15 min of exposure, the bacterial solutions were added dropwise on glass slides and observed by using an inverted fluorescence microscope (Axio Vert A1, Carl Zeiss Microscopy GmbH, Germany) with an appropriate filter (BP: 470/40, FT: 495, BP: 525/50).
Moreover, to determine whether the generation of ROS is one of the main factors for the antibacterial properties of the EGaIn film, an ROS inhibitor was used to test if the antibacterial ability was decreased. L-Ascorbic acid (Xilong Scientific Co Ltd, China) was added to the bacterial solution of 105 CFU ml−1 to prepare a series of inoculum with L-ascorbic acid concentrations of 0, 0.15, 0.3, 0.5, 1, 2 mM. 100 μl of the inoculum was added dropwise onto the EGaIn and PVC piece, covered by the PP film, incubated for 4 h, and washed with 2 ml of D-PBS. The number of live bacteria in the solution was determined by the spread-plate method.
3. Results and discussion
3.1 Gallium in the metallic state is the main composition in EGaIn films
We analyzed the surface composition of the EGaIn film by XPS. The spectra of Ga 3d and In 3d5/2 from the EGaIn pieces with and without 1 h of UV irradiation are shown in Fig. 2. In Fig. 2(a) and (b), the peaks located at 16.5 ± 0.1 and 17.3 ± 0.1 eV correspond to indium in the metallic state and In2O3, whereas three others at 18.4 ± 0.1, 20.0 ± 0.1, and 20.7 ± 0.1 eV are due to the gallium in the metallic state and oxidation of gallium, Ga2O and Ga2O3, respectively. In Fig. 2(c) and (d), the peaks located at 443.8 or 444 eV correspond to indium in the metallic state, whereas two others at 445.3 ± 0.1 or 445.7 ± 0.1 eV are due to In2O3.34,35 The results show that the peaks of the gallium oxide in the UV irradiated samples were higher than that those in the samples without UV radiation, whereas there was no obvious difference of indium's spectra between the two samples. We calculated the composition of the surfaces according to the XPS peak intensity and the results are shown in Table S1 (ESI†). The result indicates that the UV irradiation mainly resulted in an increase of gallium oxide on the surface. However, considering that the depth of XPS detection was only several nanometers and the intensity of the peak for gallium in the metallic state was still strong, the main composition of the surface was still gallium and indium in the metallic state.34,36
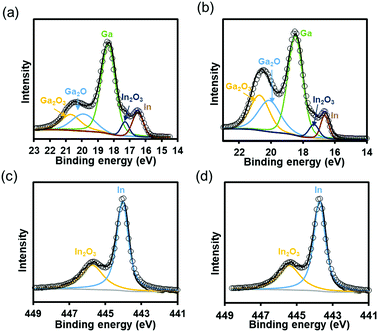 |
| Fig. 2 The spectra of Ga 3d from the samples (a) without and (b) with the 1 hour UV irradiation, and the spectra of In 3d5/2 (c) without and (d) with UV irradiation. | |
3.2 EGaIn films showed superior antibacterial activity against E. coli and S. aureus
The bacteria-killing kinetics of the EGaIn pieces against the bacterial strains and their control groups (PVC pieces) are shown in Fig. 3(a) and (b) (E. coli) and Fig. 3(c) and (d) (S. aureus). The viable counts of bacterial cells decreased sharply when exposed to EGaIn. For E. coli, a decrease of one order of magnitude of the CFUs was found after 2 hours of incubation and there were no viable cells at 10 h. For S. aureus, it takes 20 h to kill all the cells on the EGaIn pieces. The dynamic growth trends of the two bacteria on the PVC pieces were the typical growth curves. Representative results of the spread-plate method were also shown in the images. For the agar plates, photos of subimages (a) and (c), bacterial cultures on the EGaIn pieces were washed with 2 ml of D-PBS and then 100 μl of the solutions were spread over the surface of the agars. After 12–16 h of incubation, no colony can be found on the agar plates. For the agar plate photos of subimages (b) and (d), bacterial cultures on the PVC pieces were washed with 2 ml of D-PBS, diluted 105 times, and then 100 μl of the diluted solution was spread over the surface of the agars. After 12–16 h of incubation, hundreds of colonies can be found on the agar plates. The relative sterilizing rate of the bacteria was calculated by using the following equation: | R (%) = (C − A)/C × 100% | (1) |
where R is the relative sterilizing rate, C is the mean number of bacteria on the PVC piece, and A is the mean number of bacteria on the EGaIn piece. According to JIS Z 2801:2010, the relative sterilizing rate was calculated by using the results at 24 h. For the EGaIn piece, since the viable counts of E. coli and S. aureus cells were zero after 10 h and 20 h, respectively, the relative sterilizing rates of E. coli and S. aureus were both 100%, respectively.
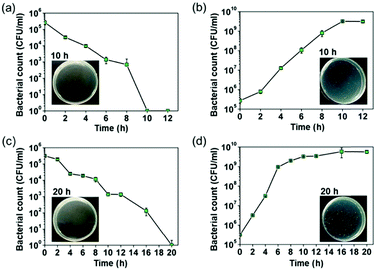 |
| Fig. 3 The growth curve of the bacterial cells with and without EGaIn treatment. (a) and (b) The viable count of E. coli cells on EGaIn pieces and PVC pieces at different incubation times. (c) and (d) The viable count of S. aureus cells on EGaIn pieces and PVC pieces at different incubation times. The count numbers were shown on a LOG scale with a base of 10. | |
3.3 EGaIn film interrupts proliferation of E. coli and S. aureus
The SEM and TEM image analyses were performed to visualize the ultrastructural damage caused by EGaIn treatment to bacterial cells. The electron micrographs of E. coli and S. aureus cells after 12 h of exposure to EGaIn are shown in Fig. 4. Bacterial cells cultured on the PVC film for the same period acted as a control. From the SEM images of E. coli cells, the cells in the control group showed typical characters of rod shape and regular sizes (see Fig. 4a), while in the EGaIn group, the number of cells decreased sharply, and we can find some very long E. coli cells (see Fig. 4b). This abnormal cell size suggests that exposure to EGaIn may influence the cycle regulation and division of E. coli cells. From the SEM images of S. aureus cells, the cells in the control group were smooth and intact (see Fig. 4c), while the cells exposed to EGaIn looked dehydrated (see Fig. 4d). More importantly, we also found that the amitosis of S. aureus was seriously affected. In the control group, the bacteria in the division phase were symmetrically distributed and the chromatin filaments could be clearly observed (Fig. 4e). However, in the EGaIn film treatment group, the size and shapes of the splitting progenies were different, and the chromatin filament in the smaller progeny disappeared (Fig. 4f). We speculate that exposure to EGaIn can influence the growth and division of the S. aureus cells, which causes cell death.
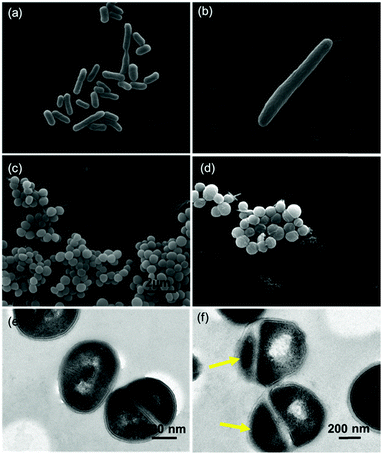 |
| Fig. 4 SEM image of E. coli cells after 12 h of culture on the PVC film (a) and EGaIn film (b), and SEM and TEM image of S. aureus cells after 12 h of culture on the PVC film (c and e) and EGaIn film (d and f). E. coli cells exposed to EGaIn showed abnormal length, and the number of cells decreased sharply. S. aureus cells exposed to EGaIn looked dehydrated. The yellow arrows point the anucleate cells suggesting the abnormal cell division. | |
3.4 EGaIn film showed higher antibacterial activity compared to gallium salt with equivalent initial ion concentration
Dissolved ions are the main reason for the antibacterial abilities of metals, such as Ag, Zn, and Cu.37,38 Gallium and indium ions have also been proved to have significant inhibitory activity against numerous bacteria.39–41 To determine whether the antibacterial activity of the EGaIn film is mainly due to the release of Ga and In ions, we first design a non-contact antibacterial test. We put a PTFE membrane with 0.4 μm pores on the EGaIn piece to prevent direct contact between the bacterial cells and EGaIn. The results showed that the CFUs of E. coli cells on the EGaIn pieces with and without the PTFE membrane were both zero after 12 h of incubation (see Fig. S1, ESI†). For the S. aureus cells, the number of viable cells on the PTFE-covered EGaIn piece became zero after 20 h of incubation, which was also consistent with the results of the uncovered EGaIn piece. Because the size of bacterial cells is larger than the PTFE pore size, we may exclude that Ga ions or In ions penetrate the PTFE membrane and affect the growth of the cells.
Then we measured their concentrations in the solution on the EGaIn pieces at different contact times. The results of Ga were 0.377 ± 0.015, 0.390 ± 0.026, and 0.483 ± 0.025 μmol ml−1 for 4, 8, and 24 h, respectively, while the concentration of dissolved In was considered far less than 1 ppm since it was too low to be readout. Thus, Ga was considered as the main active metal. Indium may devote negligible antibacterial activity in the experiment. We also tested the antibacterial effect of pure Ga films and the results are shown in Fig. S2 and Table S2 (ESI†). Compared with the results of the EGaIn film and the pure Ga film, the trends of the viable count of bacterial cells on both films were almost the same. The pure Ga film showed similar anti-bacterial activity to the EGaIn film, which could kill all the E. coli and S. aureus cells within 10 h and 20 h, respectively. The results also demonstrated that it is gallium, and not indium that plays a vital role in the antibacterial activity.
In the previous study, Ga(NO3)3 has been shown to reduce the bacterial growth rate significantly.42 In this study, we prepared three Ga(NO3)3·xH2O solutions in which concentrations of Ga were accurately identified as 0.5, 0.6, and 0.7 μmol ml−1 by using ICP-AES. These three concentrations were all higher than the measured highest concentration of dissolved Ga from the EGaIn film. However, after 10 h of incubation, the CFUs in Ga(NO3)3·xH2O solutions were still at 108 and 109 levels (shown in Fig. 5), which showed significant difference with EGaIn films (Fig. 3a and c). Therefore, we found that the antibacterial performance of the gallium metal and gallium salt with the same Ga concentration was different.
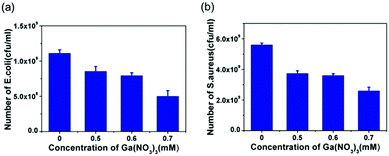 |
| Fig. 5 The number of viable (a) E. coli cells and (b) S. aureus cells in Ga(NO3)3·xH2O solution. | |
3.5 Antibacterial activity of EGaIn film is due to increased intracellular ROS generation
ROS generation was confirmed with the help of 2′-7′-dichlorofluorescein diacetate (DCFH-DA), a ROS marker. Hydrogen peroxide (H2O2), a well-known potent oxidant and a non-radical ROS, was used as the positive control. For both E. coli and S. aureus cells, the results showed that ROS fluorescence emissions were observed from bacterial cells treated with EGaIn and H2O2, while gallium nitrate and PVC groups (without EGaIn exposure) did not emit fluorescence (Fig. 6(a)–(h)), which indicated that EGaIn exposure could produce ROS.
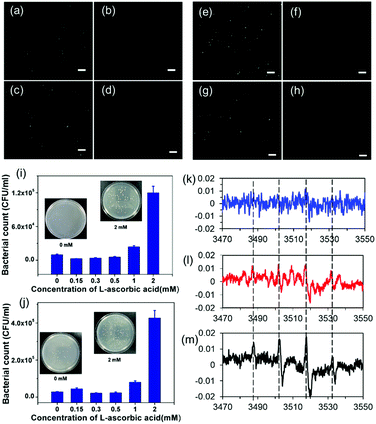 |
| Fig. 6 ROS staining images of E. coli cells from (a) EGaIn piece, (b) PVC piece, (c) treated with 0.1 mM of H2O2 and (d) 0.7 mM of Ga(NO3)3·xH2O, respectively. The DCFH-DA (green emission, λex = 480 nm, λem = 525 nm) fluorescence dyes were used for ROS detection. Scale bar = 10 μm. ROS staining images of S. aureus cells from (e) EGaIn piece, (f) PVC piece, (g) treated with 0.1 mM of H2O2 and (h) 0.7 mM of Ga(NO3)3·xH2O, respectively. The DCFH-DA (green emission, λex = 480 nm, and λem = 525 nm) fluorescence dyes were used for ROS detection. Scale bar = 10 μm. The viable bacterial count of (i) E. coli cells and (j) S. aureus cells in different concentrations of L-ascorbic acid solution on the EGaIn pieces after 4 h of incubation. Representative results of the spread-plate method were also shown in the images. For the agar plate photos, bacterial cultures on the EGaIn pieces were washed with 2 ml of D-PBS, diluted 10 times, and then 100 μl of the solutions were spread over the surface of the agars for incubation and CFU counting. For E. coli and S. aureus cells, the numbers of visible colonies on the agar plates increased to about 10 times and 20 times from 0 mM to 2 mM of L-ascorbic acid solution, respectively. (k) Influence of the presence of bacterial cells on ROS generation. Relative amounts of ROS produced in LB medium on the EGaIn film, (l) LB medium with E. coli cells on the EGaIn film was assessed by electron paramagnetic resonance (EPR) spectra, and (m) 10% H2O2 solution after 10 min UV, respectively. | |
To further confirm the antibacterial activity of ROS, different concentrations of L-ascorbic acid were added to the bacterial solution and cell viability on the EGaIn film was tested. L-Ascorbic acid is an antioxidant agent, which can be used as a ROS inhibitor. L-Ascorbic acid was added to the bacterial solution to prepare a series of inoculum with different L-ascorbic acid concentrations. The number of colonies in the solutions after 4 h of incubation is shown in Fig. 6(i) and (j). Compared with CFUs in the bacterial solution without L-ascorbic acid, the viability of E. coli and S. aureus cells on the EGaIn film increased sharply, especially when adding more than 1 mM of L-ascorbic acid to the solution. Adding antioxidants reduced the antibacterial activity of EGaIn, which indicated that ROS generation could be one of the main mechanisms of the antibacterial activity for EGaIn.
As we know, ROS include a number of reactive molecules and free radicals derived from molecular oxygen, which include hydrogen peroxide (H2O2), superoxide anions (˙O2−), hydroxyl radicals (˙OH), singlet oxygen (1O2), and alpha-oxygen (α-O). These molecules show the potential to cause cellular damage.43 ROS are effective in vitro against most Gram-positive and Gram-negative organisms44,45 and can also prevent and break down the biofilm.46,47 In addition, ROS generation has been proved to be the antimicrobial mechanism of metal nanoparticles.48–50
To further investigate the resources of ROS, we performed electron paramagnetic resonance (EPR) detection by using the LB medium with and without E. coli cells incubated on the EGaIn film. EPR, also referred to as electron spin resonance (ESR), is the most definitive method for the detection, quantification and possible identification of radicals in complex systems.51 The 5,5-dimethyl-1-pyrroline N-oxide (DMPO) was used as a spin trap. The detailed methods and results of this assay are shown in the ESI.† As the signal in the ESR spectrum indicates (Fig. 6(k)), LB medium on the EGaIn film did not display an apparent response, while LB medium with E. coli cells on the EGaIn film showed a characteristic ESR signal of 1:2:2:1 quartet ((Fig. 6(l) and (m))), which is considered to be the DMPO-OH adduct,52,53 relatively stable paramagnetic species. In our experimental system, incubating the EGaIn with culture medium did not produce ROS, and ROS could only be generated with the involvement of bacterial cells.
3.6 The addition of exogenous iron increases bacterial growth on LM films
A well-known anti-bacterial mechanism of gallium is that gallium can disrupt bacterial iron metabolism because it substitutes for iron when taken up by bacteria.42 To verify whether this phenomenon occurs on the EGaIn film, exogenous iron was added to test if the antibacterial ability of the EGaIn film was decreased. Before the test, we measured the concentration of Fe in the medium before and after the EGaIn film was exposed by using ICP-AES. The concentration of Fe in the LB medium was 5.46 ± 0.04 μM and the concentration of Fe in the LB medium exposed to EGaIn for 4 h was 5.51± 0.06 μM (Fig. S3, ESI†). There is no significant difference between the two groups.
Iron addition in the form of iron trichloride (FeCl3), purchased from Shanghai Macklin Biochemical Co., Ltd (China), was performed in the bacterial solution of 105 CFU ml−1 to prepare a series of inoculum with FeCl3 concentrations of 0, 50, 100, and 200 μM for the E. coli cells, and 0, 5, 10, 20, 60, 100, and 200 μM for the S. aureus cells. 100 μl of the inoculum was added dropwise onto the EGaIn, covered by the PP film, incubated for 4 h, and washed with 2 ml of D-PBS. The number of live bacteria in the solution was determined by the spread-plate method. We also performed this experiment in 0, 50, and 200 μM of FeCl3 solution on PVC films as control and the results are shown in Fig. S4 (ESI†).
The number of colonies in the solutions after 4 h of incubation is shown in Fig. 7(a) and (b). For E. coli cells, the viable count slightly increased with 50 μM and 100 μM of FeCl3 addition. When adding 200 μM of FeCl3, the viable count increased to about 1000 times compared with the sample without FeCl3. For S. aureus cells, with only 5 μM of FeCl3 addition, the viable count increased 10-fold. When the added FeCl3 concentration was increased to 100 μM, the number of bacteria increased in the same order of magnitude as the bacteria on the PVC sheet.
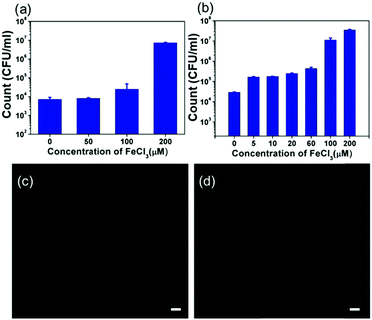 |
| Fig. 7 The viable count of (a) E. coli cells and (b) S. aureus cells in different concentrations of FeCl3 solution on the EGaIn pieces after 4 h of incubation. ROS staining images of (c) E. coli cells on the EGaIn film with 100 μM FeCl3 added, (d) S. aureus cells on the EGaIn film with 100 μM FeCl3 added, respectively. The DCFH-DA (green emission, λex = 480 nm, λem = 525 nm) fluorescence dyes were used for ROS detection. Scale bar = 10 μm. | |
We also performed the ROS detection involving FeCl3. For both E. coli and S. aureus cells, the results showed that no fluorescence emission was observed from bacterial cells treated with EGaIn and 100 μM of FeCl3 (Fig. 7(c) and (d)), which indicated that FeCl3 addition inhibited the ROS generation, therefore, the antibacterial effect of the EGaIn exposure was reduced.
3.7 The possible mechanism of antibacterial activity of LM films
As far as we know, there is no explicit investigation concerning the anti-bacterial activity of the EGaIn film. According to the above results, a schematic illustration is provided to explain the anti-bacteria effect of the EGaIn film (Fig. 8). It is shown that EGaIn exposure can disrupt bacterial growth by substituting for iron (Fig. 7), and enhanced production of ROS in the cells (Fig. 6) may be due to the generation of Ga3+ ion penetration and intracellular diffusion, which inhibits bacterial division and proliferation and consequently inhibits specific essential biological processes (Fig. 4).
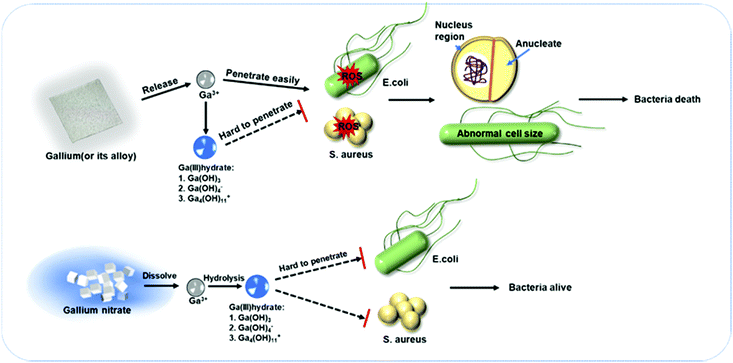 |
| Fig. 8 Schematic illustration of enhanced anti-bacterial mechanism of the EGaIn film compared to gallium salt. | |
The generation of ROS induces oxidative damage to DNA, including strand breaks and nucleotide modifications, and improved guanosine content.54 The cell division is arrested by the expression change of more than 40 genes. Particularly, SOS-related gene SulA is upregulated as one of the important factors to mediate inhibition of cell division in E. coli.55,56 Its product binds to FtsZ that is a key protein for septum formation when cells are in the dividing state, which inhibits FtsZ assembly followed by influencing Z-ring formation,57 and finally induces cell elongation.58
It is worth noting that gallium based liquid metal films also showed superior antibacterial behavior compared to gallium salts with the same or even higher gallium ion concentration (Fig. 5). It is assumed that there is a difference in the release rate of Ga3+ between gallium (or gallium alloys) and gallium nitrate in the medium. When gallium nitrate was added to the medium, the dissolution process is completed in a short time, so gallium ions are immediately released into the medium. The pH value of the LB medium was measured as 6.97. At this pH value, gallium ions will be hydrolyzed and exist as hydrated ions or molecules,59–61 and the reaction for the hydrolysis can be written as follows:
| pGa3+ + qH2O ↔ Gap(OH)q3p−q + qH+ | (2) |
where
p and
q are positive integers. According to
ref. 59–61, gallium ions mainly give rise to monomeric hydroxide Ga(OH)
3 at around pH 7. During addition of gallium nitrate, all gallium ions are exposed to a neutral water environment, and there is sufficient time to completely hydrolyze the gallium ion during the preparation of the solution to the antibacterial test. In contrast, the gallium metal or its alloys release gallium ions throughout the antibacterial test. Therefore, a number of gallium ions may be captured by bacteria before being hydrolyzed, and these fresh unhydrolyzed gallium ions exhibit stronger antibacterial activity. However, it is quite difficult to detect these unhydrolyzed gallium ions in a solution environment with an ultralow total gallium concentration (<10
−5 M).
Compared to organic antimicrobial agents, the critical advantages of using metals as anti-bacterial materials include stability, robustness, and long shelf-life. It is also worth noting that the fabrication of the EGaIn film is facile and simple, and the film itself exhibits anti-bacterial activity which could increase the stability as well as prolong the life-span of the EGaIn-based printed circuit.
4. Conclusions
In summary, a facile EGaIn film was fabricated and proved to exhibit a strong anti-bacterial effect. This paper established for the first time the mechanistic route underlying the antibacterial activity of the EGaIn film. It is demonstrated that the generation of ROS from gallium ions played an important role in inhibiting bacterial proliferation. The exposure of bacteria to ROS causes abnormal fission of bacteria, resulting in mutations and cell death. As a promising electronic ink in addictive manufacturing, EGaIn exhibits intrinsic anti-bacterial behavior that is beneficial for its stable applications in soft electronics.
Conflicts of interest
There are no conflicts to declare.
Acknowledgements
This work was supported by the National Natural Science Foundation of China (No. 31770107, 21874116, and 51706236). The authors wish to thank the Testing Center of Antimicrobial Materials, Technical Institute of Physics and Chemistry (TCAM, TIPC), CAS for issuing test report (ESI†) and Dr Jun Liu (TCAM, TIPC, CAS) for his insightful discussion and help.
References
-
J. Liu and L. Yi, Liquid Metal Biomaterials: Principles and Applications, Springer, Singapore, 2018 Search PubMed.
- L. Wang and J. Liu, ECS J. Solid State Sci. Technol., 2015, 4, P3057–P3062 CrossRef CAS.
- S. Tang, K. Khoshmanesh, V. Sivan, P. Petersen, A. P. O’Mullane, D. Abbott, A. Mitchell and K. Kalantar-zadeh, Proc. Natl. Acad. Sci. U. S. A., 2014, 111, 3304–3309 CrossRef CAS.
- S. H. Jeong, A. Hagman, K. Hjort, M. Jobs, J. Sundqvist and Z. Wu, Lab Chip, 2012, 12, 4657–4664 RSC.
- S. Cheng and Z. Wu, Adv. Funct. Mater., 2011, 21, 2282–2290 CrossRef CAS.
- Y. Zheng, Z. He, Y. Gao and J. Liu, Sci. Rep., 2013, 3, 1786 CrossRef.
- H. Ota, K. Chen, Y. Lin, D. Kiriya, H. Shiraki, Z. Yu, T. J. Ha and A. Javey, Nat. Commun., 2014, 5, 5032 CrossRef CAS.
- E. Palleau, S. Reece, S. C. Desai, M. E. Smith and M. D. Dickey, Adv. Mater., 2013, 25, 1589–1592 CrossRef CAS.
- Q. Wang, Y. Yu, K. Pan and J. Liu, IEEE Trans. Biomed. Eng., 2014, 61, 2161 Search PubMed.
- X. Sun, B. Yuan, W. Rao and J. Liu, Biomaterials, 2017, 146, 156–167 CrossRef CAS.
- Y. Liu, K. Hu and Y. Wang, Polymers, 2017, 9, 215 CrossRef.
- H. Ge, H. Li, S. Mei and J. Liu, Renewable Sustainable Energy Rev., 2013, 21, 331–346 CrossRef CAS.
- Y. Ding, Z. Deng, C. Cai, Z. Yang, Y. Yang, J. Lu, Y. Gao and J. Liu, Int. J. Thermophys., 2017, 38, 91 CrossRef.
- S. Mei, Y. Gao, Z. Deng and J. Liu, J. Electron. Package, 2014, 136, 011009 CrossRef.
- Q. Zhang and J. Liu, Nano Energy, 2013, 2, 863–872 CrossRef CAS.
- S. Mei, Y. Gao, H. Y. Li and Z. Deng, Appl. Phys. Lett., 2013, 102, 041905 CrossRef.
- J. Liu, Y. X. Zhou, Y. G. Lv and T. Li, Mech. Eng., 2005, 5, 501–510 Search PubMed.
- Y. Deng and J. Liu, Heat Mass Transfer, 2010, 46, 1327–1334 CrossRef CAS.
- Y. Deng and J. Liu, J. Therm. Sci. Eng. Appl., 2012, 4, 024501 CrossRef.
- L. I. Haiyan and J. Liu, Front. Energy, 2011, 5, 20–42 CrossRef.
- X. H. Yang, S. C. Tan, Y. J. Ding, L. Wang, J. Liu and Y. X. Zhou, Int. Commun. Heat Mass Transfer, 2017, 87, 118–124 CrossRef CAS.
- Y. Gao, X. Wang, J. Liu and Q. Fang, J. Electron. Package, 2017, 139, 011002 CrossRef.
- S. C. Tan, Y. X. Zhou, L. Wang and J. Liu, Sci. China: Technol. Sci., 2016, 59, 301–308 CrossRef CAS.
- Y. X. Gao, H. Y. Li and J. Liu, PLoS One, 2012, 7, e45485 CrossRef CAS.
- Y. Zheng, Z. Z. He, J. Yang and J. Liu, Sci. Rep., 2014, 4, 4588 CrossRef.
- R. Guo, J. B. Tang, S. J. Dong, J. Lin, H. Z. Wang, J. Liu and W. Rao, Adv. Mater. Technol., 2018, 3, 1800265 CrossRef.
- M. Kurtjak, M. Vukomanović, L. Kramer and D. Suvorov, J. Mater. Sci.: Mater. Med., 2016, 27, 170 CrossRef.
- S. R. Choi, B. E. Britigan, D. M. Moran and P. Narayanasamy, PLoS One, 2017, 12, e0177987 CrossRef.
- P. Narayanasamy, B. L. Switzer and B. E. Britigan, Sci. Rep., 2015, 5, 8824 CrossRef CAS.
- S. R. Choi, B. E. Britigan and P. Narayanasamy, Antimicrob. Agents Chemother., 2017, 61, 02505 Search PubMed.
- E. R. Soto, O. O'Connell, F. Dikengil, P. J. Peters, P. R. Clapham and G. R. Ostroff, J. Drug Delivery, 2016, 2016, 8520629 Search PubMed.
- Q. Zhang, Y. X. Gao and J. Liu, Appl. Phys. A: Mater. Sci. Process., 2014, 116, 1091–1097 CrossRef CAS.
- T. Lu, L. Finkenauer, J. Wissman and C. Majidi, Adv. Funct. Mater., 2014, 24, 3351–3356 CrossRef CAS.
- F. Scharmann, G. Cherkashinin, V. Breternitz, C. Knedlik, G. Hartung, T. Weber and J. A. Schaefer, Surf. Interface Anal., 2004, 36, 981–985 CrossRef CAS.
- J. W. Boley, E. L. White, G. T. C. Chiu and R. K. Kramer, Adv. Funct. Mater., 2014, 24, 3501–3507 CrossRef CAS.
- A. Zavabeti, J. Z. Ou, B. J. Carey, N. Syed, R. Orrell-Trigg, E. L. H. Mayes, C. Xu, O. Kavehei, A. P. O'Mullane, R. B. Kaner, K. Kalantar-Zadeh and T. Daeneke, Science, 2017, 358, 332–335 CrossRef CAS.
- C. Espirito Santo, E. W. Lam, C. G. Elowsky, D. Quaranta, D. W. Domaille, C. J. Chang and G. Grass, Appl. Environ. Microbiol., 2011, 77, 794–802 CrossRef.
- M. Yasuyuki, K. Kunihiro, S. Kurissery, N. Kanavillil, Y. Sato and Y. Kikuchi, Biofouling, 2010, 26, 851–858 CrossRef CAS.
- L. C. Antunes, F. Imperi, F. Minandri and P. Visca, Antimicrob. Agents Chemother., 2012, 56, 5961–5970 CrossRef CAS.
- M. Vaidya, A. J. McBain, C. E. Banks and K. A. Whitehead, Int. Biodeterior. Biodegrad., 2019, 141, 39–43 CrossRef CAS.
- A. A. Oliveira, G. M. Perdigao, L. E. Rodrigues, J. G. da Silva, E. M. Souza-Fagundes, J. A. Takahashi, W. R. Rocha and H. Beraldo, Dalton Trans., 2017, 46, 918–932 RSC.
- C. H. Goss, Y. Kaneko, L. Khuu, G. D. Anderson, S. Ravishankar, M. L. Aitken, N. Lechtzin, G. Zhou, D. M. Czyz, K. McLean, O. Olakanmi, H. A. Shuman, M. Teresi, E. Wilhelm, E. Caldwell, S. J. Salipante, D. B. Hornick, R. J. Siehnel, L. Becker, B. E. Britigan and P. K. Singh, Sci. Transl. Med., 2018, 10, aat7520 CrossRef.
- G. Pizzino, N. Irrera, M. Cucinotta, G. Pallio, F. Mannino, V. Arcoraci, F. Squadrito, D. Altavilla and A. Bitto, Oxid. Med. Cell. Longev., 2017, 2017, 8416763 Search PubMed.
- F. F. Sperandio, Y. Y. Huang and M. R. Hamblin, Recent Pat Antiinfect Drug Discovery, 2013, 8, 108–120 CrossRef CAS.
- Q. Chen, J. Li, Y. Wu, F. X. Shen and M. S. Yao, RSC Adv., 2013, 3, 13835–13842 RSC.
- K. Gozzi, C. Ching, S. Paruthiyil, Y. Zhao, V. Godoy-Carter and Y. Chai, npj Biofilms Microbiomes, 2017, 3, 8 CrossRef.
- M. S. Dryden, J. Cooke, R. J. Salib, R. E. Holding, T. Biggs, A. A. Salamat, R. N. Allan, R. S. Newby, F. Halstead, B. Oppenheim, T. Hall, S. C. Cox, L. M. Grover, Z. Al-Hindi, L. Novak-Frazer and M. D. Richardson, J. Glob Antimicrob Resist., 2017, 8, 186–191 CrossRef.
- A. Joe, S.-H. Park, D.-J. Kim, Y.-J. Lee, K.-H. Jhee, Y. Sohn and E.-S. Jang, J. Solid State Chem., 2018, 267, 124–133 CrossRef CAS.
- H. Xu, F. Qu, H. Xu, W. Lai, Y. Andrew Wang, Z. P. Aguilar and H. Wei, Biometals, 2012, 25, 45–53 CrossRef CAS.
- Y. N. Slavin, J. Asnis, U. O. Hafeli and H. Bach, J. Nanobiotechnol., 2017, 15, 65 CrossRef.
- G. D'Errico, G. Vitiello, G. De Tommaso, F. K. Abdel-Gawad, M. V. Brundo, M. Ferrante, A. De Maio, S. Trocchia, A. R. Bianchi, G. Ciarcia and G. Guerriero, Environ. Res., 2018, 165, 11–18 CrossRef.
- Q. Wu, N. Xia, D. Long, L. Tan, W. Rao, J. Yu, C. Fu, X. Ren, H. Li, L. Gou, P. Liang, J. Ren, L. Li and X. Meng, Nano Lett., 2019, 19, 5277–5286 CrossRef CAS.
- G. Applerot, J. Lellouche, A. Lipovsky, Y. Nitzan, R. Lubart, A. Gedanken and E. Banin, Small, 2012, 8, 3326–3337 CrossRef CAS.
- S. Burney, J. C. Niles, P. C. Dedon and S. R. Tannenbaum, Chem. Res. Toxicol., 1999, 12, 513–520 Search PubMed.
- O. Huisman and R. Dari, Nature, 1981, 290, 797–799 CrossRef CAS.
- D. Trusca, S. Scott, C. Thompson and D. Bramhill, J. Bacteriol., 1998, 180, 3946–3953 CrossRef CAS.
- E. J. Harry, Mol. Microbiol., 2001, 40, 795–803 CrossRef CAS.
- Y. Chen, S. L. Milam and H. P. Erickson, Biochemistry, 2012, 51, 3100–3109 CrossRef CAS.
- B. Hacht, Bull. Korean Chem. Soc., 2008, 29, 372–376 CrossRef CAS.
- T. Moeller and G. L. King, J. Phys. Colloid Chem., 1950, 54, 999–1011 CrossRef CAS.
- Y. F. Orlov, E. I. Maslov and E. I. Belkina, Russ. J. Inorg. Chem., 2013, 58, 1306–1314 CrossRef CAS.
Footnotes |
† Electronic supplementary information (ESI) available. See DOI: 10.1039/d0tb00174k |
‡ Equal contribution. |
|
This journal is © The Royal Society of Chemistry 2021 |