DOI:
10.1039/D0SM01581D
(Paper)
Soft Matter, 2021,
17, 83-89
Membrane reinforcement in giant hybrid polymer lipid vesicles achieved by controlling the polymer architecture†
Received
1st September 2020
, Accepted 13th October 2020
First published on 14th October 2020
Abstract
The physical properties of membranes of hybrid polymer lipid vesicles are so far relatively unknown. Since their discovery a decade ago, many studies have aimed to show their great potential in many fields of application, but so far, few systematic studies have been carried out to decipher the relationship between the molecular characteristics of the components (molar mass, chemical nature, and architecture of the copolymer), the membrane structure and its properties. In this work, we study the association of 1-palmitoyl-2-oleoyl-sn-glycero-3-phosphocholine (POPC) and poly(dimethylsiloxane)-b-poly(ethylene oxide) (PDMS-b-PEO) diblock copolymers of different molar masses in giant hybrid vesicles and establish a complete phase diagram of the membrane structure. We also measured the mechanical properties of the giant hybrid unilamellar vesicle (GHUV) through micropipette aspiration at different lipid/polymer compositions. Thanks to a previous work using triblock PEO-b-PDMS-b-PEO copolymers, we were able to reveal the effect of the architecture of the block copolymer on membrane structure and properties. Besides, the association of diblock copolymers PDMS-b-PEO and POPC leads to the formation of hybrid vesicles with unprecedented membrane toughness.
Introduction
Hybrid vesicles have become the subject of a growing number of studies by different scientific communities (chemists, physico-chemists, biophysicists, biologists, and pharmacists) in the last few years. This is due to the fact that these structures could synergistically combine the advantages of each component (permeability and biofunctionality of lipid bilayers, and chemical versatility and mechanical stability of polymer membranes). This is of great interest in applications such as controlled and targeted drug delivery, development of artificial cells or bioinspired micro/nanoreactors. Although the field of research is still in its infancy, several reviews have been written on the subject1–4 and a special issue in the journal polymers is focused on these systems:
https://www.mdpi.com/journal/polymers/special_issues/hybrid_polymer_lipid.
So far, many studies have sought to highlight their potential for different pharmaceutical, biological or biochemical applications such as in bioreactors,5–12 drug transport13–19 or molecular recognition.20 A relatively small number of studies have focused on the self-assembly and the relationship between membrane structure and properties of these hybrid systems.21–23 The molecular and macroscopic parameters that govern the membrane structure in the homogenous mixing of lipid and polymers or in phase separation leading to the presence of micron size or nanoscale domains are partially known. It is however of great importance to understand and ultimately control these properties in order to obtain optimized systems for future biomedical applications.
At the micrometer scale, a homogeneous distribution of the components seems to be favoured if the lipid phase is in a fluid state and at a relatively low fraction.23–26 Above a given lipid fraction, not yet rationalized in the literature, micrometer domains start to appear and eventually evolve towards budding and fission phenomena depending on the line tension at the lipid domain boundaries. This parameter is partially modulated by the hydrophobic length mismatch at the polymer/lipid interface and consequently by the molar mass of the hydrophobic part of the copolymers. Although this parameter plays a role in the structure of hybrid membranes, it has not yet been quantified for these systems.
Regarding their membrane properties, most of the studies have focused on the membrane permeability. It has been evaluated so far essentially through drug release experiments.10,15,16,27,28 However, it is relatively hard to extract a general conclusion on the diffusivity of a molecule through a hybrid membrane as the systems and loaded molecules were variable and the membrane structure was not resolved. Information about thermomechanical properties is also relatively scarce. Studies on PBD-b-PEO/POPC25 and PEO-b-PDMS-b-PEO/POPC29 hybrid giant vesicles realized through micropipette techniques have shown a modulation of area expansion modulus by the membrane composition. However, a dramatic decrease of the membrane toughness in the case of the triblock PEO-b-PDMS-b-PEO copolymer was observed. Another study on a PDMS-g-PEO/DPPC giant hybrid unilamellar vesicle (GHUV) mentioned that the area expansion modulus of the hybrid membrane was almost unchanged upon the addition of DPPC, compared to the polymer membrane.30 In another study, the membrane modulus of a DPPC vesicle obtained by measuring shrinking of the GUV during osmotic shock experiments was observed to increase upon lateral co-assembly of PEO-b-PDMS-b-PEO copolymers or PEO-b-PCL-b-PEO.31 Again, there is no clear trend regarding the influence of the polymer/lipid composition and membrane structure on the mechanical properties of the resulting hybrid membrane.
In this work, we propose a complete investigation of the membrane mechanical properties of a GHUV obtained with the diblock copolymers PDMS-b-PEO and POPC and compare the results with those obtained in a previous study with the triblock copolymers PEO-b-PDMS-b-PEO.29 We aim to ascertain the effect of the architecture of the copolymer on the mechanical properties of the resulting hybrid vesicles. We focused on a range of lipid compositions where homogenous membranes were obtained, at least at the micrometer scale.
Materials and methods
Materials
1-Palmitoyl-2-oleoyl-sn-glycero-3-phosphocholine (POPC), 1,2-dioleoyl-sn-glycero-3-phosphoethanol-amine-N-(lissamine rhodamine B sulfonyl) (PE-rhodamine), sucrose, glucose, bovine serum albumin and all organic solvents were purchased from Sigma Aldrich and used without further purification. Different PDMS-b-PEO copolymers were synthesized according to a previously described protocol.32 A brief description of the synthesis procedure is provided in the ESI.† The molecular characteristics of the copolymers are illustrated in Table 1.
Table 1 Molecular characteristics of the copolymers and the phospholipid used in this work and membrane thickness of the vesicles obtained. The molecular characteristics of the triblock copolymer used in previous studies23,29 are included
Compound |
Abbrev. |
Molar massa (g mol−1)/Dispersity |
Hydrophobic molar massa (g mol−1) |
Membrane thicknessb (nm) |
Molar mass determined by 1H NMR.
Membrane thickness determined by SANS as in ref. 32 and 33.
|
1-Palmitoyl-2-oleoyl-sn-glycero-3-phosphocholine |
POPC |
760 |
— |
4.7 ± 0.9 |
— |
PDMS23-b-PEO1332 |
Si23EO13 |
2500 |
1700 |
6.9 ± 1.0 |
1.15 |
PDMS27-b-PEO1732 |
Si27EO17 |
2900 |
2000 |
8.4 ± 1.1 |
1.11 |
PDMS36-b-PEO2332 |
Si36EO23 |
4000 |
2700 |
9.9 ± 1.6 |
1.04 |
PEO8-b-PDMS22-b-PEO823,29 |
EO8Si22EO8 |
2700 |
1600 |
5.4 ± 0.4 |
1.18 |
PEO12-b-PDMS43-b-PEO1223,29 |
EO12Si43EO12 |
5000 |
3200 |
8.8 ± 0.5 |
1.28 |
GHUV preparation
All giant unilamellar vesicles in this study were prepared by the same process based on the electroformation method reported by Angelova and Dimitrov34 at room temperature. The probes were used at a concentration of 0.2 wt% for PE-rhodamine and 1.5 wt% for PDMS-NBD. Briefly, mixtures of the appropriate lipids, polymers, and probes were prepared in chloroform at a total concentration of 1 mg ml−1. About 50 μl of this solution was spread slowly on the conductive faces of two indium tin oxide coated glass slides (ITO, 30–60 Ω sq−1 from Sigma Aldrich). The slides were sealed on both sides of a rubber spacer using grease to form a chamber and dried under vacuum for 3 h. The slides were connected to an AC voltage generator using electric wires, a typical sinusoidal tension (2 V, 10 Hz) was applied and the chamber was filled with a 100 mM sucrose solution. The vesicles were collected after 75 minutes and kept in the dark at room temperature.
Confocal imaging
All images were acquired using a Leica TCS SP5 (Leica Microsystems CMS GmbH, Mannheim, Germany) inverted confocal microscope (DMI6000) equipped with a HCX PL APO x63 NA 1.4 oil immersion objective (Zeiss). A 50 μL aliquot of the GUV suspension was added into an eight-well μ-Slide (Ibidi, Martinsried, Germany) containing 150 μL of iso-osmolar (100 mM) glucose solution. GUVs were allowed to sediment for 10 min before imaging. PDMS-NBD and rhodamine-PE were used as markers of the polymer and lipid phases, respectively, and were separately imaged using an argon laser line with an excitation/range of emission respectively of 488 nm/500–530 nm and 514 nm/600–700 nm. The images were processed using Leica and ImageJ software.
Micropipette aspiration experiment
The micropipette aspiration technique was also carried out using a Leica TCS SP5 (Leica Microsystems CMS GmbH, Mannheim, Germany) inverted confocal microscope (DMI6000) equipped with a x63 apochromatic water immersion objective with a NA of 1.2 (Zeiss, Jena Germany). Micropipettes were obtained by stretching Borosilicate capillaries (1 mm OD, 0.58 mm ID) from WPI, using a pipette puller (Sutter Instrument P-97). The pulled pipettes were then forged to the desired diameter using a Narishige MF-900 micro-forge. The micropipettes were coated with BSA to prevent vesicle adhesion. The vesicle tension was controlled using a home-made hydraulic watertight setup, and the micropipette was controlled using a micromanipulator (Eppendorf, Patchman NP2).
The suction pressure exerted over the membrane can be calculated from:
where
ρ is the water density,
g is the gravitational acceleration,
h is the position of the water tank and
h0 is the initial position where the pressure is zero.
The membrane tension was calculated classically from the Laplace equation:
| 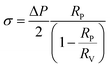 | (2) |
where
RP and
RV are respectively the micropipette and vesicle radii (outside the micropipette), and Δ
P is the suction pressure. The relative area change of the membrane (
α) is defined as:
|  | (3) |
where
A0 is the membrane area of the vesicle at the lower suction pressure.
α can be estimated from the increase in projection length Δ
L of the vesicle inside the capillary tip according to
eqn (4):
| 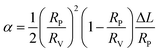 | (4) |
The surface area strain can be linked to the membrane tension through the following equation:
| 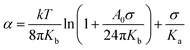 | (5) |
where
k is the Boltzmann constant,
T is the temperature and
Kb and
Ka are the bending and stretching modulus, respectively. According to previous work, at high tension, membrane undulations are completely suppressed and membrane area increases as a result of increased spacing between molecules, giving access to the area compressibility modulus:
| 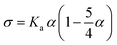 | (6) |
Each parameter measured in this work is the average of the values of at least 15 vesicles unless specified. The vesicles were analyzed one hour after electroformation.
Detailed protocols35 and a video demonstration on how to set up a micropipette aspiration experiment are also available at the following link:
https://www.jove.com/video/60199/obtention-giant-unilamellar-hybrid-vesicles-electroformation.
It should be noted that the micropipette experiments have been performed without fluorescent probes, as an effect of the probes has been detected on mechanical properties of the membrane, as described in ESI† (Fig. S1).
Results
Membrane structure
Three diblock copolymers (Si23EO13, Si27EO17 and Si36EO23) were used in the formation of the GHUVs, using different proportions of POPC. The structure of the GHUV membrane was studied using confocal microscopy on a population large enough (between 200 and 800 objects counted per sample) to be representative. The proportion of each structure was counted manually from images taken in different randomly selected areas of the sample. Depending on the copolymer used and the POPC fraction, we could observe different structures (Fig. 1). The observed structures are consistent with the multicomponent membrane, and in particular with the hybrid membrane morphologies described in the literature.23–25 The simplest morphology corresponds to a homogeneous mixture of the copolymer and the lipid within the membrane. The signals of PE-Rhodamine and PDMS-NBD are, in this case, visible throughout the vesicle. This morphology is called “homogeneous membrane” (Fig. 1A and a). An inhomogeneous distribution of the probes within the membrane indicates the formation of copolymer or lipid-enriched domains as a result of phase separation (Fig. 1B and b). The spherical shape of the vesicle is maintained and, in most cases, only one domain per vesicle is visible. The size of the domains can vary, showing a slight difference in the lipid fraction from one vesicle to another. Another type of morphology observed corresponds to the separation of the vesicles into two hemispheres, each incorporating a different probe. This shape is due to the phenomenon of budding of a domain outside the plane of the membrane (Fig. 1C and c). Vesicles marked only by one of the two probes have also been observed. These vesicles are enriched in either polymer or lipid, and result from the fission of hybrid membranes through the budding process (Fig. 1C and d).
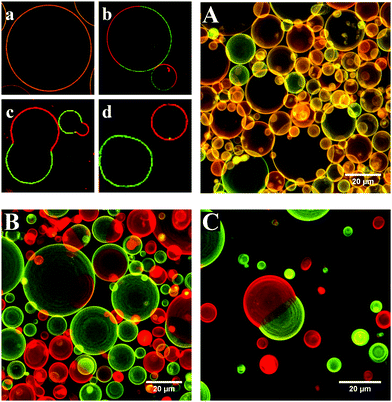 |
| Fig. 1 (A–C) 3D reconstruction based on confocal images taken along the z-axis illustrating the different structures. (a) Equatorial slice of a GHUV presenting a homogeneous membrane (PDMS-NBD and rhodamine-PE perfectly overlapped). (b) Equatorial slice of a GHUV presenting domains while maintaining the spherical shape of the vesicle. (c) Equatorial slice of a GHUV presenting a budded part (growing of a second spherical structure from a preformed domain). (d) Equatorial slice of a GUV composed exclusively of lipid resulting from the fission of a budded GHUV. | |
By measuring the proportion of each structure at different lipid contents, we were able to draw an apparent phase diagram for the three hybrid systems (Fig. 2).
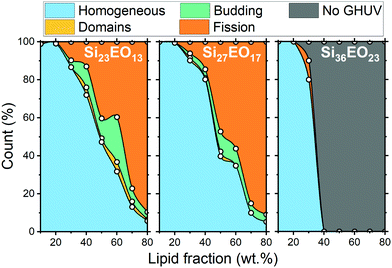 |
| Fig. 2 Apparent phase diagrams of the GHUVs formed from POPC and the diblock copolymers. | |
In the case of the two shortest diblock copolymers (Si23EO13 and Si27EO17), GHUVs are formed over the entire range of POPC fractions, as observed for their triblock analogs.23 Whatever the copolymer used, homogeneous membranes were formed at low POPC contents. POPC is dispersed in a continuous polymer phase until a limit fraction is reached. Above this limit (50 wt% for Si23EO13, 50 wt% for Si27EO17 and 30 wt% for Si36EO23), demixing of the system is favored. Evidence of phase separation can be found in systems composed of Si23EO13 and Si27EO17. The domains enriched in lipid or copolymer are both circular, illustrating the fluidity of the membrane. About 25% of the vesicles form buds, especially for Si23EO13 at about 60 wt% POPC. The buds can be formed either from lipid or polymer domains and are stable over two to four days. Upon further increasing the lipid fraction, the GHUVs tend to undergo fission. The fission fraction becomes predominant for Si23EO13 from 65 wt% POPC, and for Si27EO17 from 55 wt% POPC.
The behavior of Si36EO23 differs from the other systems: homogeneous membranes formed at low lipid fractions, however no GHUV could be observed above 40 wt% POPC. Liposomes were visible, but they did not appear to be the result of GHUV fission as no intermediate step (formation of spherical domains and/or budding) could be observed. The polymer was sometimes present as aggregates, attached to the liposome membrane (see Fig. S2 in the ESI†).
Mechanical properties
We studied the mechanical properties of the GHUV membrane. The objective was to evaluate the effect of the membrane composition and to verify that hybrid vesicles do indeed have potential in terms of mechanical strength compared to liposomes. Hybrid systems developed from triblock copolymers have shown lower toughness than those of liposomes.29 In the literature, it has been observed that vesicles obtained from PBD-b-PEO diblock copolymers show tenacities much higher than those of POPC but also higher than those obtained from PEO-b-PDMS-b-PEO triblock copolymers.25 As vesicles obtained from PDMS-b-PEO copolymers present very high tenacities,32 even higher than those of vesicles obtained from PBD-b-PEO reported in the literature, advantageous mechanical properties of GHUVs obtained from these diblock PDMS-b-PEO copolymers are expected. We evaluated the membrane properties of vesicles with a homogeneous membrane structure using the micropipette suction method.
The evolution of the membrane structure of each system was first checked under tension. Indeed, in one of our previous studies, it was found that for hybrid vesicles composed of POPC and triblock copolymers, micro-domains can appear under stress when the membrane composition is close to the limit where micro-domains spontaneously appear. These micro-domains result from the coalescence of nano-domains that were revealed by FLIM FRET.23 For all the systems studied, the membranes remain homogeneous under tension (Fig. 3).
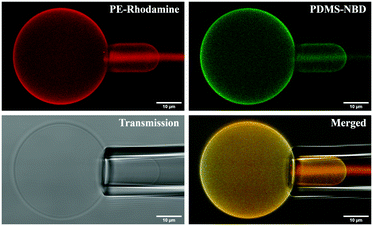 |
| Fig. 3 Confocal images of micropipette experiment in the different observation modes: PE Rhodamine channel, PDMS NBD channel, PE-Rhodamine and PDMS NBD channel merged, and transmission. | |
The mechanical properties were then determined in the absence of any probe, in order to measure the intrinsic properties of the membrane. The study carried out here is limited to POPC fractions up to 20 wt%. The mechanical properties of the homogeneous membranes were measured by micropipette aspiration until rupture was reached. Pure liposomes were also studied. The curves obtained (Fig. S3, ESI†) were fitted using eqn (6) to determine the area expansion modulus. The lysis strain (γc) and stress (σc) were determined from the abscissa and ordinate of the last point of the curves, respectively. Membrane toughness was obtained by calculating the area under the curves. The mean curves (Fig. 4) were plotted using the mean area expansion modulus and the mean lysis strain determined using eqn (6) (Table S1, ESI†).
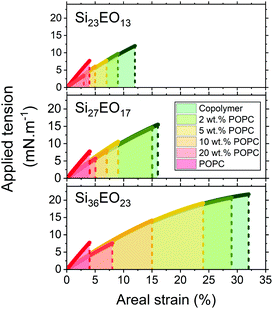 |
| Fig. 4 Average stress–strain curves of the GHUVs prepared from Si23EO13/POPC (top panel), Si27EO17/POPC (middle panel) and Si36EO23/POPC (bottom panel). Colored areas under the curves represent the average toughness. | |
The area expansion moduli of the GHUVs remain almost constant whatever the lipid composition, between 100 and 130 mN m−1. However, lysis strain and stress decrease progressively with the lipid fraction and increase with the molecular weight of the hydrophobic block (Fig. 5). The lysis strain remains higher than that of POPC, except for GHUVs composed of the lower molar mass copolymer (Si23EO13) with 20 wt% POPC. Concerning the lysis stress, the systems show lower values than POPC above 5 wt% for GHUVs composed of Si23EO13, 10 wt% for GHUVs composed of Si27EO17 and 20 wt% for GHUVs composed of Si36EO23.
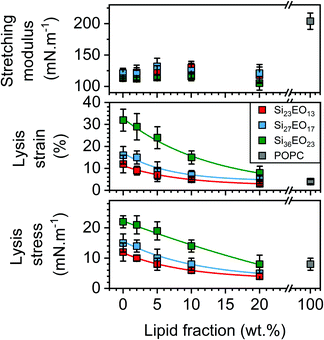 |
| Fig. 5 Mechanical properties determined from the stress–strain curves: stretching modulus (top panel), lysis strain (middle panel) and lysis stress (bottom panel). | |
To compare the mechanical resistance of the GHUVs, as previously stated, we measured the toughness of the hybrid membranes by calculating the area under the curve. A decrease in toughness with the POPC fraction was observed (Fig. 6). GHUVs composed of Si23EO13 have the lowest tenacities and therefore the most fragile membranes. The tenacity of this system remained higher than that of POPC up to 10 wt% POPC. GHUVs composed of Si27EO17 have a higher tenacity than the lipid membranes up to a fraction of 20 wt% POPC. Finally, GHUVs made of Si36EO23 have the most resistant membranes, with a tenacity always higher than POPC whatever the fraction of POPC in the studied range. For comparison, the triblock PEO12-b-PDMS43-b-PEO12 forms GHUVs with a membrane toughness lower than that of POPC even at a low lipid fraction (5 wt% POPC).29
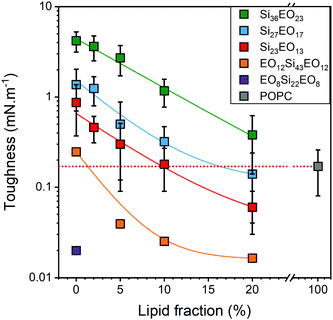 |
| Fig. 6 Toughness of the GHUVs composed of diblock and triblock copolymers. The dashed red line is a guide for the eye to show the typical toughness of a liposome. | |
Discussion
The membrane structure of the GHUVs has been studied over the entire range of POPC composition using PE-Rhodamine as the lipid marker and PDMS-NBD as the polymer marker. The observed structures are in agreement with those reported for different hybrid systems: a homogeneous mixture for low lipid fractions is obtained, followed by phase separation beyond a certain limit fraction, and then budding and fission phenomena as the lipid fraction increases (Fig. 2).23,30 The structures obtained do not differ from those obtained for GHUVs composed of PEO-b-PDMS-b-PEO triblock copolymers. The diblock copolymers used in this study lead to the formation of membranes with similar thicknesses to those investigated with these triblock copolymers. Therefore, it appears that for identical chemical nature is identical, the architecture of the copolymer used has no significant effect on the membrane structure of the GHUVs. Indeed, the phase separation phenomenon for GHUVs formed from diblock copolymers is comparable to that observed with triblock copolymers. The boundary fractions follow more or less the same evolution with the hydrophobic mismatch whatever the architecture of the copolymer. The difference in line tension at the lipid polymer boundaries associated with the different conformations of diblock and triblock copolymers in the membrane is probably not high enough to create a difference in the phase separation phenomenon.
In the same manner as for the triblock copolymers, the hydrophobic length mismatch between the polymer and the lipid strongly influences the structure of the membrane (homogeneous mixing or phase separation) as well as the stability of the domains and buds once the phase separation is achieved.
For a small hydrophobic length difference (e.g., hybrids composed of Si23EO13), the polymer/lipid interface is stable enough to produce heterogeneous membranes. Thus, a significant number of GHUVs will present domains as well as buds (up to 25% buds for 60 wt% POPC). An intermediate hydrophobic length difference (e.g., hybrids composed of Si27EO17) will produce less GHUVs showing domains and buds. This originates probably from a higher line tension at the polymer/lipid interfaces, leading more rapidly to the formation of polymersomes and liposomes by budding and fission phenomena. This budding phenomenon allows a decrease of the interfacial energy by reducing the interfacial length when line tension is too high36,37 and can ultimately evolve towards fission. For example, 50% fission is obtained for 55 wt% POPC and Si27EO17, whereas the lipid fraction has to be increased to 65 wt% to obtain the same amount of fission with Si23EO13.
Finally, a high hydrophobic length difference (e.g., hybrids composed of Si36EO23) will prevent the formation of GHUVs with domains or buds. The incompatibility between the polymer and lipid phase may be so high that the polymer tolerates a very low amount of lipid, and almost complete phase separation occurs as soon as the film is formed.
Micropipette suction experiments have been used to determine the mechanical properties of the GHUVs with a homogeneous membrane at the micrometer scale and assess the influence of the lipid fraction and length of the copolymer hydrophobic block. The high hydrophobic length difference for Si36EO23 does not appear to cause membrane embrittlement. GHUVs composed of diblock copolymers have mechanical properties far superior to what could be obtained for triblock systems. The origin of the brittleness of hybrid systems formulated from triblock copolymers and the outstanding toughness of those obtained from diblock copolymers of the same chemical nature remains to be determined. It is possible that differences in chain conformation in the membrane (between triblock and diblock copolymers) may play a role. A pure bilayer conformation is expected for a diblock copolymer whereas a mixture of extended and loop conformations is expected for a triblock copolymer.38,39 The GHUV membrane obtained from diblock copolymers did not show any sign of nanodomain segregation and merging into micrometer domains, as described for triblock copolymer based GHUVs.23 The existence of lipid nanodomains is therefore unlikely.
The values of stretching modulus are very close to the stretching moduli obtained for polymersomes and do not vary significantly with the lipid fraction (Fig. 6) in contrast to what has been observed for hybrid systems based on PEO-b-PDMS-b-PEO/POPC29 and PBD-b-PEO/POPC.25 This result is surprising in that a modulation of the membrane composition should lead to a change in the interfacial tension between hydrophilic and hydrophobic parts and thus a change in the stretching modulus.40,41 The latter therefore seems not to be influenced by the presence of the lipid in the membrane.
Conclusions
In this work, we reported a systematic study of the formation of GHUVs from the self-assembly of PDMS-b-PEO diblock copolymers and POPC, and their mechanical properties. A comparison with a previous study realized with triblock PEO-b-PDMS-b-PEO copolymers leading to the formation of vesicles with membrane thickness similar to that of the diblock copolymers studied here has been performed. Globally, the evolution of membrane structure follows the same tendency with lipid composition whatever the architecture of the copolymer. Therefore, it seems that the different conformations of the polymer chains (extended and loop for triblock, bilayer for diblock) do not play a major role in the membrane structure. However, a huge difference has been evidenced in terms of membrane toughness. GHUVs obtained with PDMS-b-PEO diblock copolymers show outstanding toughness, especially for high molar masses, even at relatively high fractions of POPC (15-20 wt%), whereas it has been shown previously that the association of PEO-b-PDMS-b-PEO triblock copolymers with POPC led to the formation of GHUVs that could be even more fragile than pure liposomes. Quantitative measurements of line tension at the lipid–polymer boundaries could help to improve the qualitative interpretation of such differences and are planned in a further study. We think that this architecture effect could be extrapolated to copolymers of different chemical nature. Indeed, for instance, diblock PBD-b-PEO copolymers, which are some of the most investigated block copolymers for developing hybrid vesicles, present, at low lipid fractions, similar tendencies in terms of membrane structure and mechanical properties.25 Such a hypothesis should be confirmed in further studies.
Conflicts of interest
There are no conflicts to declare.
Acknowledgements
M. Fauquignon gratefully acknowledges the Scientific Department of University of Bordeaux for a PhD fellowship.
Notes and references
- A. Krywko-Cendrowska, S. di Leone, M. Bina, S. Yorulmaz-Avsar, C. G. Palivan and W. Meier, Polymers, 2020, 12, 1003 CrossRef CAS.
- M. Schulz and W. H. Binder, Macromol. Rapid Commun., 2015, 36, 2031–2041 CrossRef CAS.
- J. F. Le Meins, C. Schatz, S. Lecommandoux and O. Sandre, Mater. Today, 2013, 16, 397–402 CrossRef CAS.
-
T. P. T. Dao, K. Ferji, F. Fernandes, M. Prieto, S. Lecommandoux, E. Ibarboure, O. Sandre and J.-F. Le Meins, in The Giant Vesicle Book, ed. C. M. Marques and D. Dimova, CRC Press, 2020, ch. 27 Search PubMed.
- C. Kleineberg, C. Wolfer, A. Abbasnia, D. Pischel, C. Bednarz, I. Ivanov, T. Heitkamp, M. Borsch, K. Sundmacher and T. Vidakovic-Koch, ChemBioChem, 2020, 21(15), 2149–2160 CrossRef CAS.
- T. Nishimura, S. Hirose, Y. Sasaki and K. Akiyoshi, J. Am. Chem. Soc., 2020, 142, 154–161 CrossRef CAS.
- S. Di Leone, S. Y. Avsar, A. Belluati, R. Wehr, C. G. Palivan and W. Meier, J. Phys. Chem. B, 2020, 124, 4454–4465 CrossRef CAS.
- Y. Zhang, N. Gal, F. Itel, I. N. Westensee, E. Brodszkij, D. Mayer, S. Stenger, M. Castellote-Borrell, T. Boesen, S. R. Tabaei, F. Höök and B. Städler, Nanoscale, 2019, 11, 11530–11541 RSC.
- P. A. Beales, S. Khan, S. P. Muench and L. J. C. Jeuken, Biochem. Soc. Trans., 2017, 45, 15–26 CrossRef CAS.
- W. F. Paxton, P. T. McAninch, K. E. Achyuthan, S. H. R. Shin and H. L. Monteith, Colloids Surf., B, 2017, 159, 268–276 CrossRef CAS.
- R. Seneviratne, S. Khan, E. Moscrop, M. Rappolt, S. P. Muench, L. J. C. Jeuken and P. A. Beales, Methods, 2018, 147, 142–149 CrossRef CAS.
- S. Khan, M. Li, S. P. Muench, L. J. C. Jeuken and P. A. Beales, Chem. Commun., 2016, 52, 11020–11023 RSC.
- S. Khan, J. McCabe, K. Hill and P. A. Beales, J. Colloid Interface Sci., 2020, 562, 418–428 CrossRef CAS.
- M. Mumtaz Virk and E. Reimhult, Langmuir, 2018, 34, 395–405 CrossRef CAS.
- N. Pippa, M. Merkouraki, S. Pispas and C. Demetzos, Int. J. Pharm., 2013, 450, 1–10 CrossRef CAS.
- S. Lim, H.-P. de Hoog, A. Parikh, M. Nallani and B. Liedberg, Polymers, 2013, 5, 1102–1114 CrossRef.
- N. Pippa, D. Stellas, A. Skandalis, S. Pispas, C. Demetzos, M. Libera, A. Marcinkowski and B. Trzebicka, Eur. J. Pharm. Biopharm., 2016, 107, 295–309 CrossRef CAS.
- K. Panneerselvam, M. E. Lynge, C. F. Riber, S. Mena-Hernando, A. A. Smith, K. N. Goldie, A. N. Zelikin and B. Stadler, Biomicrofluidics, 2015, 9, 052610 CrossRef.
- Z. Cheng, D. R. Elias, N. P. Kamat, E. Johnston, A. A. Poloukhtine, V. V. Popik, D. A. Hammer and A. Tsourkas, Bioconjugate Chem., 2011, 22, 2021–2029 CrossRef CAS.
- M. Schulz, S. Werner, K. Bacia and W. H. Binder, Angew. Chem., Int. Ed., 2013, 52, 1829–1833 CrossRef CAS.
- S. K. Lim, A. S. W. Wong, H.-P. M. de Hoog, P. Rangamani, A. N. Parikh, M. Nallani, S. Sandin and B. Liedberg, Soft Matter, 2017, 13, 1107–1115 RSC.
- S.-W. Hu, C.-Y. Huang, H.-K. Tsao and Y.-J. Sheng, Phys. Rev. E, 2019, 99, 012403 CrossRef CAS.
- T. P. T. Dao, F. Fernandes, E. Ibarboure, K. Ferji, M. Prieto, O. Sandre and J.-F. Le Meins, Soft Matter, 2017, 13, 627–637 RSC.
- J. Nam, T. K. Vanderlick and P. A. Beales, Soft Matter, 2012, 8, 7982–7988 RSC.
- J. Nam, P. A. Beales and T. K. Vanderlick, Langmuir, 2011, 27, 1–6 CrossRef CAS.
- M. Chemin, P. M. Brun, S. Lecommandoux, O. Sandre and J. F. Le Meins, Soft Matter, 2012, 8, 2867–2874 RSC.
- W. Shen, J. Hu and X. Hu, Chem. Phys. Lett., 2014, 600, 56–61 CrossRef CAS.
- M. Kang, B. Lee and C. Leal, Chem. Mater., 2017, 29, 9120–9132 CrossRef CAS.
- T. P. T. Dao, F. Fernandes, M. Fauquignon, E. Ibarboure, M. Prieto and J. F. Le Meins, Soft Matter, 2018, 14, 6476–6484 RSC.
- D. Chen and M. M. Santore, Soft Matter, 2015, 11, 2617–2626 RSC.
- J. Y. Kang, I. Choi, M. Seo, J. Y. Lee, S. Hong, G. Gong, S. S. Shin, Y. Lee and J. W. Kim, J. Colloid Interface Sci., 2020, 561, 318–326 CrossRef CAS.
- M. Fauquignon, E. Ibarboure, S. Carlotti, A. Brûlet, M. Schmutz and J.-F. Le Meins, Polymers, 2019, 11, 2013 CrossRef CAS.
- T. P. T. Dao, A. Brûlet, F. Fernandes, M. Er-Rafik, K. Ferji, R. Schweins, J. P. Chapel, A. Fedorov, M. Schmutz, M. Prieto, O. Sandre and J. F. Le Meins, Langmuir, 2017, 33, 1705–1715 CrossRef CAS.
- M. I. Angelova and D. S. Dimitrov, Faraday Discuss. Chem. Soc., 1986, 81, 303–311 RSC.
- E. Ibarboure, M. Fauquignon and J.-F. Le Meins, JoVE, 2020, e60199 Search PubMed.
- J. Wolff, S. Komura and D. Andelman, Phys. Rev. E: Stat., Nonlinear, Soft Matter Phys., 2015, 91, 012708 CrossRef.
- M. Schulz, A. Olubummo, K. Bacia and W. H. Binder, Soft Matter, 2014, 10, 831–839 RSC.
- F. Itel, M. Chami, A. Najer, S. Loercher, D. Wu, I. A. Dinu and W. Meier, Macromolecules, 2014, 47, 7588–7596 CrossRef CAS.
- H.-C. Tsai, Y.-L. Yang, Y.-J. Sheng and H.-K. Tsao, Polymers, 2020, 12, 639 CrossRef CAS.
- B. M. Discher, Y. Y. Won, D. S. Ege, J. C. M. Lee, F. S. Bates, D. E. Discher and D. A. Hammer, Science, 1999, 284, 1143–1146 CrossRef CAS.
- H. Bermudez, A. K. Brannan, D. A. Hammer, F. S. Bates and D. E. Discher, Macromolecules, 2002, 35, 8203–8208 CrossRef CAS.
Footnote |
† Electronic supplementary information (ESI) available: Stress–strain curves; analysis of the influence of molecular probes on mechanical properties. See DOI: 10.1039/d0sm01581d |
|
This journal is © The Royal Society of Chemistry 2021 |