DOI:
10.1039/D0SC05409G
(Edge Article)
Chem. Sci., 2021,
12, 3216-3225
C4-arylation and domino C4-arylation/3,2-carbonyl migration of indoles by tuning Pd catalytic modes: Pd(I)–Pd(II) catalysis vs. Pd(II) catalysis†
Received
30th September 2020
, Accepted 7th January 2021
First published on 7th January 2021
Abstract
Efficient C4-arylation and domino C4-arylation/3,2-carbonyl migration of indoles have been developed. The former route enables C4-arylation in a highly efficient and mild manner and the latter route provides an alternative straightforward protocol for synthesis of C2/C4 disubstituted indoles. The mechanism studies imply that the different reaction pathways were tuned by the distinct acid additives, which led to either the Pd(I)–Pd(II) pathway or Pd(II) catalysis.
Introduction
Multi-substituted-indoles are key building blocks in a large number of natural products, pharmaceuticals and agrochemicals.1 Transition-metal-catalyzed directed C–H activation at the benzene moiety has emerged as a powerful synthetic approach to streamline the synthesis of highly substituted indoles.2 It normally requires an adjacent directing group to the C–H functionalization sites, which leads to the generation of vicinal disubstituted indoles. However, direct formation of non-vicinal disubstituted indoles via the directing group's assistance remains challenging.
To achieve this goal, several directed remote C–H functionalization strategies have been developed recently (Scheme 1A). C6-selective olefination of indoles has successfully been achieved by groups of Yu using a combination of a monoprotected amino acid ligand and the nitrile template attached at the indole nitrogen via a sulfonamide linkage (Scheme 1B).3 Frost developed an N-pyrimidinyl group assisted cycloruthenation pathway to achieve remote C6-selective alkylation.4 Shi reported a Cu(II)-diaryliodonium triflate salt catalytic system for N–P(O)tBu2 directed C6-selective arylation and C3-pivaloyl directed C5-selective arylation.5 Despite this impressive progress, the scope is limited to the synthesis of 3,5- and N,6-disubstituted indoles and strategies other than directed remote C–H activation have been elusive. Herein, we reported the first catalysis mode tuned C4-arylation/directing group migration. With different acidic additives, the different pathways were tuned to either the Pd(I)–Pd(II) pathway or Pd(II) catalysis (Scheme 1C). The Pd(I)–Pd(II) pathway enables the rapid and mild C4-arylation and the latter Pd(II) catalysis undergoes an unprecedented domino C4-arylation/3,2-carbonyl migration of indoles, which provides a straightforward protocol for synthesis of C2/C4 disubstituted indoles.
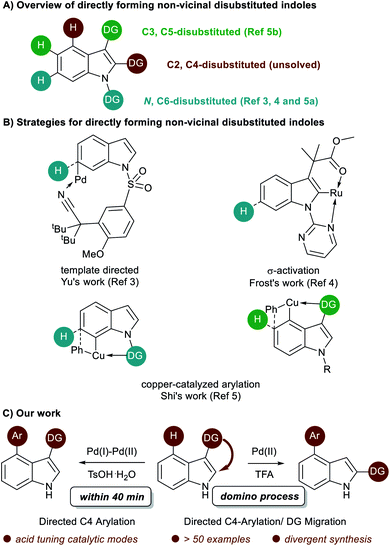 |
| Scheme 1 Transition-metal-catalyzed synthesis of non-vicinal disubstituted indoles via C–H functionalization. | |
Results and discussion
Optimization of reaction conditions
Shi5a and Zou2u reported C4/C5-arylation of a N–Bn protected indole, and C2/C4-regioselective heteroarylation of N–Me protected indoles has successfully been achieved by You's groups.2v Until now, direct C4-arylation of unprotected indoles has not been reported. Thus, we employed unprotected indoles as the starting material. As transient directing group strategies would enhance coordination between Pd catalysts and weak-coordinating directing groups,6 we commenced our investigation by evaluation of several transient directing groups (TDGs) in C4-arylation of 1-(1H-indol-3-yl)ethan-1-one (1a) with methyl 4-iodobenzoate (2a) using Pd(OAc)2 as the catalyst and AgTFA as the additive. Interestingly, both C4-arylation product 3a and unexpected 4a were obtained when using glycine as a transient directing group in the cosolvent of HFIP/HOAc (3/1, v/v, 1.0 mL) (Tables S1 in the ESI†). The structures of 3a and 4a were confirmed unambiguously by X-ray crystallography (Schemes 5 and 6, and crystallographic data in the ESI†). Extensive screening of TDGs and solvent revealed that the acid is crucial for the promotion of the reaction (Tables S1 and S2 in the ESI†). Therefore, further investigation was carried out without TDGs. Surprisingly, replacing acetic acid with TsOH·H2O significantly enabled C4-arylation in a highly efficient and mild manner, providing 3a as a sole product in 90% yield within 40 minutes (Table 1, entry 2). Notably, with trifluoroacetic acid as a co-solvent, the product 4a was selectively obtained in 75% yield (Table 1, entry 4). Further screening of other factors didn't improve the reaction efficiency. Thus, TsOH·H2O is the best acidic additive for 3a and TFA/HFIP is an optimal cosolvent for 4a.
Table 1 Optimization of the reaction conditionsa
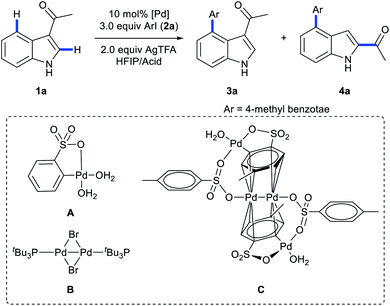
|
Entry |
HFIP/acid |
Pd catalyst |
Yield% (3a)b |
Yield% (4a)b |
Reaction conditions: 1a (0.2 mmol), 2a (3.0 equiv.), AgTFA (2.0 equiv.), Pd catalyst (10 mol%), HFIP/acid = 3 : 1 (v/v, 1.0 mL), 100 °C, 13 h.
Isolated yields.
C2-arylation products obtained in 20% yields.
The reaction was carried out with 1.0 mL HFIP at 60 °C in 40 minutes.
HFIP : TFA = 3 : 1 (v/v, 1.1 mL).
C2-arylation products obtained in 6% yields. HFIP = 1,1,1,3,3,3-hexafluoro-2-propanol, TsOH·H2O = p-toluenesulfonic acid monohydrate, TFA = trifluoroacetate.
|
1c |
HFIP/AcOH (3 : 1, v/v) |
Pd(OAc)2 |
50 |
6 |
2d |
TsOH·H2O (3.75 equiv.) |
Pd(OAc)2 |
90 |
— |
3 |
ClCH2COOH (3.75 equiv.) |
Pd(OAc)2 |
20 |
3 |
4e,f |
HFIP/TFA (3 : 1, v/v) |
Pd(OAc)2 |
3 |
75 |
5 |
— |
Pd(OAc)2 |
8 |
— |
6d |
TsOH·H2O (3.75 equiv.) |
Pd(OTs)2(MeCN)2 |
15 |
— |
7d |
TsOH·H2O (3.75 equiv.) |
A
|
88 |
— |
8d |
TsOH·H2O (3.75 equiv.) |
B
|
91 |
— |
9e |
HFIP/TFA (3 : 1, v/v) |
Pd(TFA)2 |
3 |
75 |
Mechanism studies: tuning the catalytic mode via acids
To probe the role of TsOH·H2O in C4-arylation, several control experiments were carried out. According to previous reports, the role of TsOH·H2O in the Pd-catalyzed reactions can be categorized into two aspects: combination of Pd(OAc)2 with TsOH·H2O would afford either electrophilic Pd(OTs)2(MeCN)2 (ref. 7) or complex A.8 Pd(OTs)2(MeCN)2 instead of Pd(OAc)2 delivered lower yields with an induction period (Table 1, entry 6 and Fig. 1a). In contrast, complex A8 provided 3a in 88% yield without the induction period (Table 1, entry 7 and Fig. 1a), suggesting that complex A would be a competent catalyst. As Bedford and coworkers revealed that complex A would be readily converted to unstable Pd(I) species C (see Table 1),8 an investigation of the possible involvement of Pd(I) species in this catalysis process was carried out. A stable dinuclear Pd(I) complex B9 was employed, providing 91% yield without the induction period (Table 1, entry 8 and Fig. 1a).10 This result is in contrast to that for a previously reported DAF-Pd(I) species, which reduces the catalytic activity in allylic C–H acetoxylation of terminal alkenes and intramolecular aza-Wacker cyclization.11 These results indicated that TsOH·H2O together with Pd(OAc)2 would form a reported Pd(I) catalyst Cin situ via complex A, which is involved in the catalytic cycle. To explore Pd species in the catalytic cycle, the X-ray photoelectron spectroscopy (XPS) measurement of the reaction mixture using the Pd(OAc)2/TsOH·H2O system was carried out. The observed peak structures indicate the presence of two distinct oxidation states of Pd species (Fig. 2). These peaks can be attributed to Pd(I) (49.77 at%) and Pd(II) (50.23 at%) without apparent Pd(0) signals,12 which shows that the C4-arylation reaction may proceed through a Pd(I)–Pd(II) mechanism. In the Pd(I)–Pd(II) catalytic pathway, involvement of silver salts is uncommon. Owing to the halogenophilicity of silver,13 Ag(I) was reported to abstract halogen during a reported Pd(I)-involved cross-coupling of enamides with α-bromocarbonyls by Loh.14 In our case, we indeed detected Ag(I) as the only silver species in XPS (Fig. 2),15 further confirming that Ag(I) acts as a halogen abstractor for aryl iodides instead of an oxidant.16 To our knowledge, this Pd(I)–Pd(II) catalytic pathway would be the first report of the Pd(I) involved C–H arylation process.8,9,11,12,14,17,18
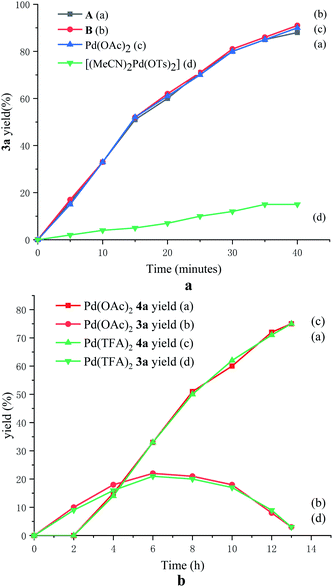 |
| Fig. 1 (a) Time-dependent formation of 3a using various Pd(I) and Pd(II) catalysts. (b) Time-dependent formation of 3a and 4a using Pd(OAc)2 and Pd(TFA)2 catalysts. | |
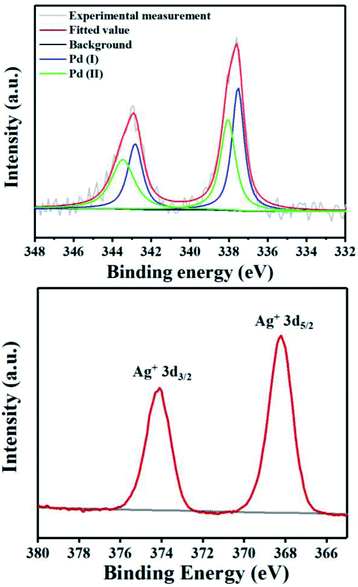 |
| Fig. 2 The X-ray photoelectron spectroscopy (XPS) data of the reaction mixture. | |
To elucidate the pathway of domino C4-arylation/3,2-carbonyl migration of indoles, several tests were carried out. Pd(TFA)2 instead of Pd(OAc)2 provided 4a in 75% yield, suggesting that Pd(OAc)2 would be readily converted to Pd(TFA)2 to catalyze reactions (Table 1, entry 9 and Fig. 1b). As we monitored the reaction for 4a, 3a was formed before the generation of 4a and the rate for 4a formation decreased after maximum production of 3a (Fig. 1b), indicating a plausible generation of 4a from 3a as path 2 (Scheme 2a). Furthermore, 1-(1H-indol-2-yl)ethan-1-one (6a) was subjected to the standard reaction conditions and failed to give the desired 4a (Scheme 2b), which ruled out path 1 and suggested a domino C4-arylation/3,2-carbonyl immigration.19
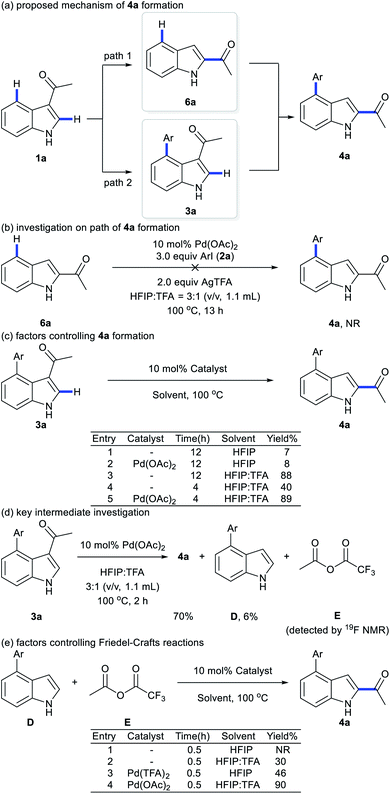 |
| Scheme 2 Pathway of domino C4-arylation/3,2-carbonyl migration of indoles. | |
To probe the role of acid in the 3,2-carbonyl migration process, several parallel experiments were conducted (Scheme 2c). HFIP as solvent with or without Pd(OAc)2 only afforded 3a in 8% or 7% yield, respectively. Addition of TFA delivered 4a with 40% yield in 4 h and 88% yield of 4a was obtained by extending the reaction time to 12 h. These results indicate that TFA might be crucial to trigger this reverse Friedel–Crafts reactions via protonation of 3a. Notably, a significant improvement of efficiency was achieved by using TFA and Pd(OAc)2 (89% yield in 4 h), indicating that cooperation of TFA with Pd(OAc)2 prompted the efficient 3,2-carbonyl migration process. Further efforts towards key intermediate trapping were carried out as well. After reacting 3a with Pd(OAc)2 in HFIP
:
TFA = 3
:
1 (v/v, 1.1 mL) at 100 °C for 2 h, the 19F NMR spectrum indicated generation of anhydride E (Fig. S4 in the ESI†) and D was isolated with 6% yield (Scheme 2d). We hypothesized that E would react with D to afford the product 4a. Indeed, when D was subjected to the reaction with E, migration product 4a was obtained in 90% yield without 3a (Scheme 2e). These outcomes suggest that reverse Friedel–Crafts reactions of species 3a might generate intermediates D and E. Next, Friedel–Crafts reactions of D selectively occurred at the C2 position with E as an intermolecular reaction, which provided product 4a. TFA would promote the Friedel–Crafts reaction of D with Evia protonation of E, which is consistent with results from Scheme 2e: the reaction between D and E failed in the absence of TFA; addition of TFA delivered 4a with 30% yield in 0.5 h. Furthermore, comparing the different results in Scheme 2e with or without Pd species, addition of Pd species would increase the reaction rate: a significant improvement of efficiency was achieved by using TFA and Pd(OAc)2 (90% yield in 0.5 h). Thus, we proposed that either Pd(TFA)2 as a Lewis acid or TFA as a Bronsted acid would activate E for Friedel–Crafts reaction of D.
When 1a was subjected to C4-arylation conditions using 1,1,1,3,3,3-hexafluoro-2-propanol-d2 as solvent and TsOD·D2O as acid additive in the presence of D2O, no D/H exchange was detected by NMR (Scheme 3). It implies that in the reaction (1) the C–H bond cleavage is an irreversible process and (2) Pd catalysts may undergo oxidative addition with iodobenzenes before C–H activation. In the C4-arylation/3,2-carbonyl migration reaction, D/H exchange was detected by NMR at C4 as well as Me, C5 and C7. It implies that in the domino reaction Pd catalysts may undergo oxidative addition with iodobenzenes after C–H activation.
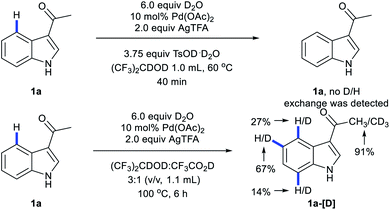 |
| Scheme 3 H/D exchange experiments. | |
Based on previous literature9 and our results, we proposed two catalytic cycles for the aforementioned reactions (Scheme 4). In the C4-arylation catalysis cycles, Pd(OAc)2 reacts with TsOH·H2O to afford Pd(I) catalytic species C,8 which then readily undergoes oxidative addition with aryl iodides to form Pd(II) species F. Subsequent C–H activation of 1a with F affords G, which undergoes reductive elimination to give C4-arylation products 3a and regenerate Pd(I) species C. In the domino C4-arylation and 3,2-carbonyl migration of indole catalysis cycles, Pd(OAc)2 reacts with TFA to afford Pd(TFA)2, which undergoes C–H activation with substrate 1a to afford species H. Oxidative addition of H with aryl iodides forms I, which undergoes reductive elimination to give species J. Reverse Friedel–Crafts reactions of J begin with the protonation at the C3 positions of indoles, providing K. K reacts with CF3COO− to generate species D and E. Friedel–Crafts reaction of species D and E releases L and CF3COO−, and regenerates Pd(TFA)2. Finally, the process of deprotonation–rearomatization of L affords product 4a and TFA.
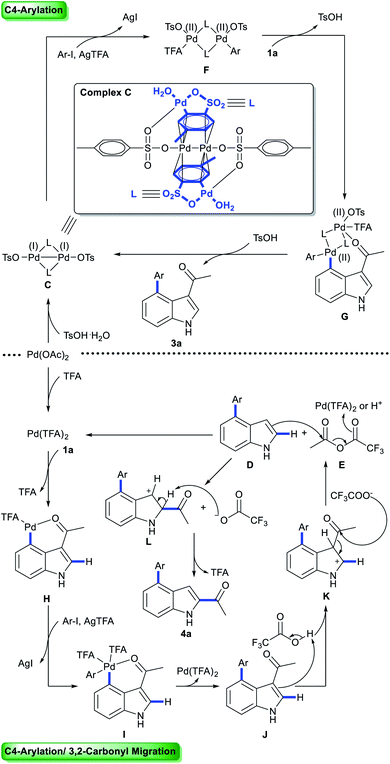 |
| Scheme 4 Proposed mechanism. | |
Substrate scope
We next explored the scope of C4-arylation under the optimized conditions (Scheme 5). Arylation of indole 1a with diverse aryl iodides was first examined. A series of aryl iodides with electron-withdrawing or electron-donating groups at the ortho, meta or para position successfully provided arylation products with moderate to good yields in 40 minutes (Scheme 5A). 4-Iodobenzonitrile with a labile cyano group also provided arylation products (3g and 3l) successfully. Although 4-iodobenzaldehyde and 4′-iodoacetophenone were not compatible with basic coupling conditions,5a they afforded the products 3h and 3i under these optimal conditions. These C4-arylations were previously inaccessible (3g and 3i). Lower aryl iodide loading (1.2 equiv.) also afforded good to excellent yields. Methyl 4-bromobenzoate provided arylation product 3a in 12% yield as well (Table S11 in the ESI†). With iodobenzene (2a) as the coupling partner, diverse indole derivatives were explored (Scheme 5B). In contrast to previous reports, various carbonyl directing groups at the C3 position proved to be viable for directed arylation (3o–3s), which provides an alternative route for direct synthesis of 3,4-disubstituted indoles. Furthermore, reactions of indoles with methyl (3t and 3u), esters (3v and 3z), fluoro (3w), chloro (3x), and bromo substituents (3y and 3aa) afforded the corresponding 4-aryl indoles in moderate to excellent yields. Although aza-indole derived 1-(1H-pyrrolo[2,3-b]pyridin-3-yl)ethan-1-one failed to give C4-arylation products (Table S13 in the ESI†), other heterocyclic substrates, such as 1-(benzo[b]thiophen-3-yl)ethan-1-one, were compatible with this reaction (3ab). Pleasingly, the robustness of this protocol can also be proven by application to highly functionalized indoles in 40 minutes (Scheme 5C). Tri-substituted indoles, such as a lilolidine derivative and bioactive 4-oxocarbazoles, afforded the desired product in excellent yields (5a–5c). Notably, this approach didn't afford arylation at the N of pyrrole, which clearly enables the rapid and modular construction of highly substituted indoles (5e) from simple and available indole substrates with minimal prefunctionalization.2c,20 Further screening of the reaction scope revealed that methyl 1H-indole-3-carboxylate and (1H-indol-3-yl)(morpholino)methanone failed to give C4-arylation products (Table S13 in the ESI†).
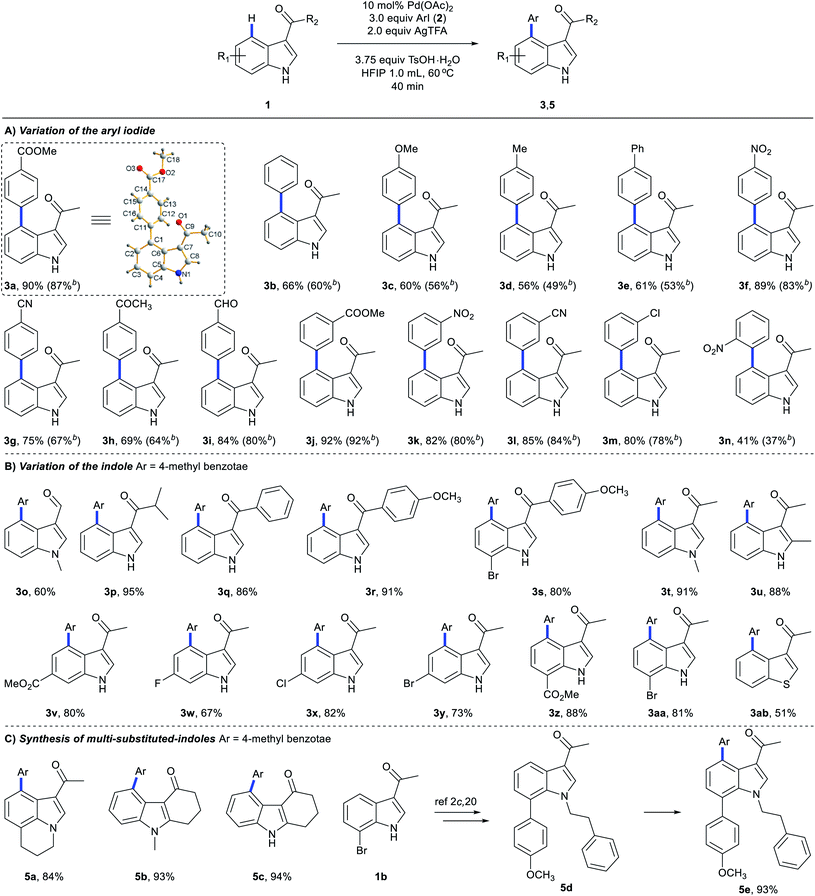 |
| Scheme 5 C4-arylation of indoles. aReaction conditions: 1 (0.2 mmol), 2 (3.0 equiv.), AgTFA (2.0 equiv.), Pd(OAc)2 (10 mol%), TsOH·H2O (3.75 equiv.), HFIP (1.0 mL), 60 °C, 40 minutes. b2 (1.2 equiv.), 90 minutes. cIsolated yields. | |
We next investigated the scope of C4-arylation and 3,2-carbonyl migration of indole under the optimal conditions (Scheme 6). Iodoarenes containing esters (4l), nitriles (4b and 4j), trifluoromethyl (4c) and nitro group (4k and 4p) afforded the desired products in moderate to good yields. Notably, reactive ketone and aldehyde functionalities on the aryl iodide remained intact during the reaction (4h and 4i). Aryl iodides containing fluoro, chloro, bromo and iodo substituents are also compatible in the reaction (4d–4g and 4m–4o), thus highlighting the potential of this process in combination with further conventional cross-coupling transformations. Besides, various carbonyl directing groups were tolerated well and gave 2,4-disubstituted indole products (4q–4t). Indoles containing halide substituents were compatible providing the corresponding products (4u–4w) in moderate to good yields. Notably, this approach enables one-pot C4-arylation and directing group removal when a thiophene derivative was employed as a substrate (4x). When trisubstituted indole 5d was subjected to these domino conditions, a similar directing-group-removal product 4y was obtained, which might be attributed to the bulkiness of the N-protecting group. C2-substituted indoles also provided 4z and 4aa with directing group removal from their 3-carbonyl indole derivatives with generation of intermediate E. Given that 2,4-disubstituted indoles are important structural units in biologically active molecules and drugs,21 this approach would provide an alternative pathway for facile construction of diverse bioactive indole building blocks. Further exploring the reaction scope revealed that 1H-indole-3-carbaldehyde, methyl 1H-indole-3-carboxylate and (1H-indol-3-yl)(morpholino)methanone failed to give C4-arylation/3,2-carbonyl migration products (Table S14 in the ESI†).
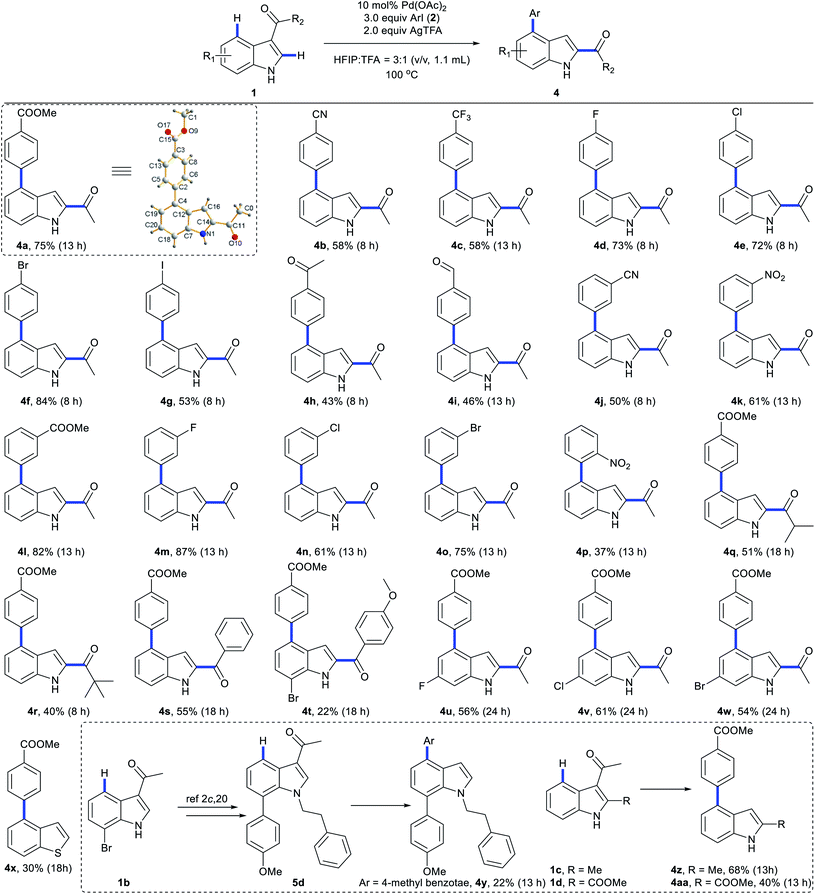 |
| Scheme 6 C4-arylation and 3,2-carbonyl migration of indoles. aReaction conditions: 1 (0.2 mmol), 2 (3.0 equiv.), AgTFA (2.0 equiv.), Pd(OAc)2 (10 mol%), HFIP : TFA = 3 : 1 (v/v, 1.1 mL), 100 °C. bIsolated yields. | |
We next examined the scope of 3,2-carbonyl migration of C3/C4-disubstituted indoles (Scheme 7). 1a without C4-substituents failed to react under migration conditions. A 4-methyl indole derivative incorporating C4 electron-donating substituents was compatible in these conditions, providing migration product 6b in 75% yield. In contrast, indoles bearing electron-withdrawing substituents (CN and NO2) at the C4 positions afforded 6c and 6d with directing group removal.
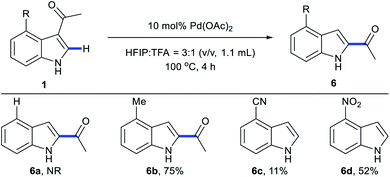 |
| Scheme 7 Substrate scope with C3/C4-disubstituted indoles. aStandard conditions: 1 (0.2 mmol), Pd(OAc)2 (10 mol%), HFIP : TFA = 3 : 1 (v/v, 1.1 mL), 100 °C, 4 h. bIsolated yields. | |
Conclusions
In summary, we have developed the C4-arylation and domino C4-arylation/3,2-carbonyl migration of indoles. The former route enables C4-arylation in a highly efficient and mild manner employing TsOH·H2O as acid additive and the latter route provides an alternative straightforward protocol for synthesis of C2/C4 disubstituted indoles. The different reaction pathways were tuned by the distinct acid additives, which led to either the Pd(I)–Pd(II) pathway or Pd(II) catalysis. Given the importance of 3,4- and 2,4-disubstituted indoles in materials science and active pharmaceutical ingredients, it is expected that the reactions will have wide application in organic chemistry, chemical materials and pharmaceutical research.
Author contributions
Y. H. C., S. J. Y. and Y. H. H. conducted all the experimental work. Y. H. C. and G. H. A. collected and analyzed the data. Y. H. C., G. H. A., G. M. L. and Z. Y. Y. wrote the paper. G. H. A., G. M. L. and Z. Y. Y. proposed and supervised the project. All the authors discussed the results and commented on the manuscript. All authors have given approval to the final version of the manuscript.
Conflicts of interest
There are no conflicts to declare.
Acknowledgements
The authors gratefully acknowledge support from the University Nursing Program for Young Scholars with Creative Talents in Heilongjiang Province (UNPYSCT-2017124). Associate Professor Hao Wu is acknowledged for his kind discussion on the XPS spectrum.
Notes and references
-
(a) J. Diesel, D. Grosheva, S. Kodama and N. Cramer, Angew. Chem., Int. Ed., 2019, 58, 11044 CrossRef CAS;
(b) G. N. Hermann, M. T. Unruh, S. H. Jung, M. Krings and C. Bolm, Angew. Chem., Int. Ed., 2018, 57, 10723 CrossRef CAS;
(c) Z. Huang, O. Kwon, H. Huang, A. Fadli, X. Marat, M. Moreau and J.-P. Lumb, Angew. Chem., Int. Ed., 2018, 57, 11963 CrossRef CAS;
(d) T. Liu, W. Zhou and J. Wu, Org. Lett., 2017, 19, 6638 CrossRef CAS;
(e) S. Maity, U. Karmakar and R. Samanta, Chem. Commun., 2017, 53, 12197 RSC;
(f) H. Nakamura, K. Yasui, Y. Kanda and P. S. Baran, J. Am. Chem. Soc., 2019, 141, 1494 CrossRef CAS;
(g) D. K. Pandey, S. B. Ankade, A. Ali, C. P. Vinod and B. Punji, Chem. Sci., 2019, 10, 9493 RSC;
(h) J. Zhang, H. Xie, H. Zhu, S. Zhang, M. Reddy Lonka and H. Zou, ACS Catal., 2019, 9, 10233 CrossRef CAS;
(i) A. J. Kochanowska-Karamyan and M. T. Hamann, Chem. Rev., 2010, 110, 4489 CrossRef CAS;
(j) G. Bartoli, R. Dalpozzo and M. Nardi, Chem. Soc. Rev., 2014, 43, 4728 RSC;
(k) J. Kalepu, P. Gandeepan, L. Ackermann and L. T. Pilarski, Chem. Sci., 2018, 9, 4203 RSC;
(l) K. Nagaraju and D. Ma, Chem. Soc. Rev., 2018, 47, 8018 RSC.
-
(a) X. Qiu, H. Deng, Y. Zhao and Z. Shi, Sci. Adv., 2018, 4, eaau6468 CrossRef CAS;
(b) A. Biswas, S. Bera, P. Poddar, D. Dhara and R. Samanta, Chem. Commun., 2020, 56, 1440 RSC;
(c) A. J. Borah and Z. Shi, Chem. Commun., 2017, 53, 3945 RSC;
(d) A. J. Borah and Z. Shi, J. Am. Chem. Soc., 2018, 140, 6062 CrossRef CAS;
(e) X. Han, Y. Yuan and Z. Shi, J. Org. Chem., 2019, 84, 12764 CrossRef CAS;
(f) C. N. Kona, Y. Nishii and M. Miura, Angew. Chem., Int. Ed., 2019, 58, 9856 CrossRef CAS;
(g) X.-H. Liu, H. Park, J.-H. Hu, Y. Hu, Q.-L. Zhang, B.-L. Wang, B. Sun, K.-S. Yeung, F.-L. Zhang and J.-Q. Yu, J. Am. Chem. Soc., 2017, 139, 888 CrossRef CAS;
(h) J. Lv, X. Chen, X. S. Xue, B. Zhao, Y. Liang, M. Wang, L. Jin, Y. Yuan, Y. Han, Y. Zhao, Y. Lu, J. Zhao, W.-Y. Sun, K. N. Houk and Z. Shi, Nature, 2019, 575, 336 CrossRef CAS;
(i) X. Qiu, P. Wang, D. Wang, M. Wang, Y. Yuan and Z. Shi, Angew. Chem., Int. Ed., 2019, 58, 1504 CrossRef CAS;
(j) M. S. Sherikar, R. Kapanaiah, V. Lanke and K. R. Prabhu, Chem. Commun., 2018, 54, 11200 RSC;
(k) N. Thrimurtulu, A. Dey, A. Singh, K. Pal, D. Maiti and C. M. R. Volla, Adv. Synth. Catal., 2019, 361, 1441 CrossRef CAS;
(l) T. Wang, L. Zhou, Y. Yang, X. Zhang, Z. Shi and Y. D. Wu, Org. Lett., 2018, 20, 6502 CrossRef CAS;
(m) Y. Yang, X. Qiu, Y. Zhao, Y. Mu and Z. Shi, J. Am. Chem. Soc., 2016, 138, 495 CrossRef CAS;
(n) J. Zhang, M. Wu, J. Fan, Q. Xu and M. Xie, Chem. Commun., 2019, 55, 8102 RSC;
(o) J. A. Leitch, C. J. Heron, J. McKnight, G. Kociok-Kohn, Y. Bhonoah and C. G. Frost, Chem. Commun., 2017, 53, 13039 RSC;
(p) J. A. Leitch, Y. Bhonoah and C. G. Frost, ACS Catal., 2017, 7, 5618 CrossRef CAS;
(q) T. A. Shah, P. B. De, S. Pradhan and T. Punniyamurthy, Chem. Commun., 2019, 55, 572 RSC;
(r) Y. Yang and Z. Shi, Chem. Commun., 2018, 54, 1676 RSC;
(s) S. K. Banjare, T. Nanda and P. C. Ravikumar, Org. Lett., 2019, 21, 8138 CrossRef CAS;
(t) S. Pradhan, M. Mishra, P. B. De, S. Banerjee and T. Punniyamurthy, Org. Lett., 2020, 22, 1720 CrossRef CAS;
(u) P.-G. Li, Y. Yang, S. Zhu, H.-X. Li and L.-H. Zou, Eur. J. Org. Chem., 2019, 73 CrossRef;
(v) S. Chen, M. Zhang, R. Su, X. Chen, B. Feng, Y. Yang and J. You, ACS Catal., 2019, 9, 6372 CrossRef CAS.
-
(a) G. Yang, P. Lindovska, D. Zhu, J. Kim, P. Wang, R.-Y. Tang, M. Movassaghi and J.-Q. Yu, J. Am. Chem. Soc., 2014, 136, 10807 CrossRef CAS;
(b) G. Yang, D. Zhu, P. Wang, R.-Y. Tang and J.-Q. Yu, Chem.–Eur. J., 2018, 24, 3434 CrossRef CAS.
- J. A. Leitch, C. L. McMullin, M. F. Mahon, Y. Bhonoah and C. G. Frost, ACS Catal., 2017, 7, 2616 CrossRef CAS.
-
(a) Y. Yang, P. Gao, Y. Zhao and Z. Shi, Angew. Chem., Int. Ed., 2017, 56, 3966 CrossRef CAS;
(b) Y. Yang, R. Li, Y. Zhao, D. Zhao and Z. Shi, J. Am. Chem. Soc., 2016, 138, 8734 CrossRef CAS.
-
(a) F. L. Zhang, K. Hong, T. J. Li, H. Park and J. Q. Yu, Science, 2016, 351, 252 CrossRef CAS;
(b) Y. Cheng, J. Zheng, C. Tian, Y. He, C. Zhang, Q. Tan, G. An and G. Li, Asian J. Org. Chem., 2019, 8, 526 CrossRef CAS;
(c) Y. Cheng, Y. He, J. Zheng, H. Yang, J. Liu, G. An and G. Li, Chin. Chem. Lett., 2021 DOI:10.1016/j.cclet.2020.09.044;
(d) B. Li, B. Lawrence, G. Li and H. Ge, Angew. Chem., Int. Ed., 2020, 59, 3078 CrossRef CAS;
(e) L. Pan, K. Yang, G. Li and H. Ge, Chem. Commun., 2018, 54, 2759 RSC;
(f) K. Yang, Q. Li, Y. Liu, G. Li and H. Ge, J. Am. Chem. Soc., 2016, 138, 12775 CrossRef CAS;
(g) P. Gandeepan and L. Ackermann, Chem, 2018, 4, 199 CrossRef CAS;
(h) Q. Shao, K. Wu, Z. Zhuang, S. Qian and J. Q. Yu, Acc. Chem. Res., 2020, 53, 833 CrossRef CAS.
-
(a) A. J. Reay, L. A. Hammarback, J. T. W. Bray, T. Sheridan, D. Turnbull, A. C. Whitwood and I. J. S. Fairlamb, ACS Catal., 2017, 7, 5174 CrossRef CAS;
(b) S. P. Cooper and K. I. Booker-Milburn, Angew. Chem., Int. Ed., 2015, 54, 6496 CrossRef CAS;
(c) C. E. Houlden, C. D. Bailey, J. G. Ford, M. R. Gagne, G. C. LloydJones and K. I. Booker-Milburn, J. Am. Chem. Soc., 2008, 130, 10066 CrossRef CAS;
(d) R. Giri, J. K. Lam and J.-Q. Yu, J. Am. Chem. Soc., 2010, 132, 686 CrossRef CAS;
(e) C. E. Houlden, M. Hutchby, C. D. Bailey, J. G. Ford, S. N. G. Tyler, M. R. Gagné, G. C. Lloyd-Jones and K. I. Booker-Milburn, Angew. Chem., Int. Ed., 2009, 48, 1830 CrossRef CAS.
- R. B. Bedford, M. F. Haddow, C. J. Mitchell and R. L. Webster, Angew. Chem., Int. Ed., 2011, 50, 5524 CrossRef CAS.
-
(a) M. Mendel, I. Kalvet, D. Hupperich, G. Magnin and F. Schoenebeck, Angew. Chem., Int. Ed., 2020, 59, 2115 CrossRef CAS;
(b) I. Kalvet, K. Deckers, I. Funes-Ardoiz, G. Magnin, T. Sperger, M. Kremer and F. Schoenebeck, Angew. Chem., Int. Ed., 2020, 59, 7721 CrossRef CAS;
(c) K. J. Bonney, F. Proutiere and F. Schoenebeck, Chem. Sci., 2013, 4, 4434 RSC;
(d) K. J. Bonney and F. Schoenebeck, Chem. Soc. Rev., 2014, 43, 6609 RSC;
(e) C. C. C. Johansson Seechurn, T. Sperger, T. G. Scrase, F. Schoenebeck and T. J. Colacot, J. Am. Chem. Soc., 2017, 139, 5194 CrossRef CAS;
(f) G. Yin, I. Kalvet and F. Schoenebeck, Angew. Chem., Int. Ed., 2015, 54, 6809 CrossRef CAS.
- Preparation of reported Pd(I) species C in Bedford reference failed owing to its high instability and ready decomposition to palladium black.
-
(a) J. N. Jaworski, S. D. McCann, I. A. Guzei and S. S. Stahl, Angew. Chem., Int. Ed., 2017, 56, 3605 CrossRef CAS;
(b) S. J. Tereniak and S. S. Stahl, J. Am. Chem. Soc., 2017, 139, 14533 CrossRef CAS;
(c) J. N. Jaworski, C. V. Kozack, S. J. Tereniak, S. M. M. Knapp, C. R. Landis, J. T. Miller and S. S. Stahl, J. Am. Chem. Soc., 2019, 141, 10462 CrossRef CAS.
-
(a) G.-Z. Wang, R. Shang, W.-M. Cheng and Y. Fu, J. Am. Chem. Soc., 2017, 139, 18307 CrossRef CAS;
(b) H.-M. Huang, P. Bellotti, P. M. Pflüger, J. L. Schwarz, B. Heidrich and F. Glorius, J. Am. Chem. Soc., 2020, 142, 10173 CrossRef CAS.
-
(a) M. Naodovic and H. Yamamoto, Chem. Rev., 2008, 108, 3132 CrossRef CAS;
(b) J.-M. Weibel, A. Blanc and P. Pale, Chem. Rev., 2008, 108, 3149 CrossRef CAS.
- R. Ding, Z.-D. Huang, Z.-L. Liu, T.-X. Wang, Y.-H. Xu and T.-P. Loh, Chem. Commun., 2016, 52, 5617 RSC.
- W. Huang, X. Kang, C. Xu, J. Zhou, J. Deng, Y. Li and S. Cheng, Adv. Mater., 2018, 30, 1706962 CrossRef.
-
(a) Y. Yu and U. K. Tambar, Chem. Sci., 2015, 6, 2777 RSC;
(b) J. Das, P. Dolui, W. Ali, J. P. Biswas, H. B. Chandrashekar, G. Prakash and D. Maiti, Chem. Sci., 2020, 11, 9697 RSC.
- For other examples of recent representative Pd(I) catalysis, see:
(a) Z.-Z. Zhou, J.-H. Zhao, X.-Y. Gou, X.-M. Chen and Y.-M. Liang, Org. Chem. Front., 2019, 6, 1649 RSC;
(b) Z. Feng, Q. Q. Min, H. Y. Zhao, J. W. Gu and X. Zhang, Angew. Chem., Int. Ed., 2015, 54, 1270 CrossRef CAS;
(c) N. J. Race, A. Faulkner, M. H. Shaw and J. F. Bower, Chem. Sci., 2016, 7, 1508 RSC;
(d) X. Bao, Q. Wang and J. Zhu, Angew. Chem., Int. Ed., 2017, 56, 9577 CrossRef CAS;
(e) W. J. Zhou, G. M. Cao, G. Shen, X. Y. Zhu, Y. Y. Gui, J. H. Ye, L. Sun, L. L. Liao, J. Li and D. G. Yu, Angew. Chem., Int. Ed., 2017, 56, 15683 CrossRef CAS;
(f) W.-M. Cheng, R. Shang and Y. Fu, Nat. Commun., 2018, 9, 5215 CrossRef;
(g) Z. Jiao, L. H. Lim, H. Hirao and J. S. Zhou, Angew. Chem., Int. Ed., 2018, 57, 6294 CrossRef CAS;
(h) S. Sumino, M. Uno, H. J. Huang, Y. K. Wu and I. Ryu, Org. Lett., 2018, 20, 1078 CrossRef CAS;
(i) L. Sun, J.-H. Ye, W.-J. Zhou, X. Zeng and D.-G. Yu, Org. Lett., 2018, 20, 3049 CrossRef CAS;
(j) S. Teng, M. E. Tessensohn, R. D. Webster and J. S. Zhou, ACS Catal., 2018, 8, 7439 CrossRef CAS;
(k) C. Wang and G. Dong, J. Am. Chem. Soc., 2018, 140, 6057 CrossRef CAS;
(l) R. Kancherla, K. Muralirajan, B. Maity, C. Zhu, P. E. Krach, L. Cavallo and M. Rueping, Angew. Chem., Int. Ed., 2019, 58, 3412 CrossRef CAS;
(m) Y. J. Mao, B. X. Wang, Q. Z. Wu, K. Zhou, S. J. Lou and D. Q. Xu, Chem. Commun., 2019, 55, 2019 RSC;
(n) N. Pirkl, A. Del Grosso, B. Mallick, A. Doppiu and L. J. Goossen, Chem. Commun., 2019, 55, 5275 RSC;
(o) N. W. J. Scott, M. J. Ford, C. Schotes, R. R. Parker, A. C. Whitwood and I. J. S. Fairlamb, Chem. Sci., 2019, 10, 7898 RSC;
(p) S. Sun, C. Zhou, J. T. Yu and J. Cheng, Org. Lett., 2019, 21, 6579 CrossRef CAS;
(q) W. L. Xing, R. Shang, G. Z. Wang and Y. Fu, Chem. Commun., 2019, 55, 14291 RSC;
(r) S. Ye, T. Xiang, X. Li and J. Wu, Org. Chem. Front., 2019, 6, 2183 RSC;
(s) B. Zhao, R. Shang, G.-Z. Wang, S. Wang, H. Chen and Y. Fu, ACS Catal., 2019, 10, 1334 CrossRef;
(t) C. Zhu, Y. F. Zhang, Z. Y. Liu, L. Zhou, H. Liu and C. Feng, Chem. Sci., 2019, 10, 6721 RSC;
(u) L. Feng, L. Guo, C. Yang, J. Zhou and W. Xia, Org. Lett., 2020, 22, 3964 CrossRef CAS;
(v) M. Koy, P. Bellotti, F. Katzenburg, C. G. Daniliuc and F. Glorius, Angew. Chem., Int. Ed., 2020, 59, 2375 CrossRef CAS;
(w) L. Li, Z. Zhao, J. Xu, H. Luo, Y. Li, X. Ma, L. Tang, B. Ren, X. Cao and Y. N. Ma, Chem. Commun., 2020, 56, 9384 RSC;
(x) M. Ratushnyy, N. Kvasovs, S. Sarkar and V. Gevorgyan, Angew. Chem., Int. Ed., 2020, 59, 10316 CrossRef CAS;
(y) K. P. Shing Cheung, D. Kurandina, T. Yata and V. Gevorgyan, J. Am. Chem. Soc., 2020, 142, 9932 CrossRef CAS;
(z) Z. Zhang, C. R. Rogers and E. A. Weiss, J. Am. Chem. Soc., 2020, 142, 495 CrossRef CAS.
- For reviews of recent representative Pd(I) catalysis, see:
(a) C. Wang and G. Dong, ACS Catal., 2020, 10, 6058 CrossRef CAS;
(b) N. Kambe, T. Iwasaki and J. Terao, Chem. Soc. Rev., 2011, 40, 4937 RSC;
(c) S. Sumino, A. Fusano, T. Fukuyama and I. Ryu, Acc. Chem. Res., 2014, 47, 1563 CrossRef CAS;
(d) Q. Liu, X. Dong, J. Li, J. Xiao, Y. Dong and H. Liu, ACS Catal., 2015, 5, 6111 CrossRef CAS;
(e) N. Hazari and D. P. Hruszkewycz, Chem. Soc. Rev., 2016, 45, 2871 RSC;
(f) M. Parasram and V. Gevorgyan, Chem. Soc. Rev., 2017, 46, 6227 RSC;
(g) D. Balcells and A. Nova, ACS Catal., 2018, 8, 3499 CrossRef CAS;
(h) P. Chuentragool, D. Kurandina and V. Gevorgyan, Angew. Chem., Int. Ed., 2019, 58, 11586 CrossRef CAS;
(i) M. R. Kwiatkowski and E. J. Alexanian, Acc. Chem. Res., 2019, 52, 1134 CrossRef CAS;
(j) S. Crespi and M. Fagnoni, Chem. Rev., 2020, 120, 9790 CrossRef CAS;
(k) W.-M. Cheng and R. Shang, ACS Catal., 2020, 10, 9170 CrossRef CAS;
(l) W.-J. Zhou, G.-M. Cao, Z.-P. Zhang and D.-G. Yu, Chem. Lett., 2019, 48, 181 CrossRef CAS;
(m) C. Fricke, T. Sperger, M. Mendel and F. Schoenebeck, Angew. Chem., Int. Ed., 2021 DOI:10.1002/anie.202011825.
- For domino reactions, see:
(a) L. F. Tietze, Chem. Rev., 1996, 96, 115 CrossRef CAS;
(b) L. F. Tietze, T. Kinzel and C. C. Brazel, Acc. Chem. Res., 2009, 42, 367 CrossRef CAS;
(c) M. Brandstatter, N. Huwyler and E. M. Carreira, Chem. Sci., 2019, 10, 8219 RSC.
- A. K. Pitts, F. O'Hara, R. H. Snell and M. J. Gaunt, Angew. Chem., Int. Ed., 2015, 54, 5451 CrossRef CAS.
-
(a) A. Kopinathan, C. Draper-Joyce, M. Szabo, A. Christopoulos, P. J. Scammells, J. R. Lane and B. Capuano, J. Med. Chem., 2019, 62, 371 CrossRef CAS;
(b) X. Wang, G. Xue and Z. Pan, Eur. J. Med. Chem., 2020, 187, 111918 CrossRef CAS;
(c) K. Zhao, R. Du, B. Wang, J. Liu, C. Xia and L. Yang, ACS Catal., 2019, 9, 5545 CrossRef CAS.
Footnote |
† Electronic supplementary information (ESI) available. CCDC 2031280 (3a) and 2031282 (4a). For ESI and crystallographic data in CIF or other electronic format see DOI: 10.1039/d0sc05409g |
|
This journal is © The Royal Society of Chemistry 2021 |
Click here to see how this site uses Cookies. View our privacy policy here.