DOI:
10.1039/D1RA08277A
(Paper)
RSC Adv., 2021,
11, 39680-39695
Metabolome-based profiling of African baobab fruit (Adansonia digitata L.) using a multiplex approach of MS and NMR techniques in relation to its biological activity†
Received
11th November 2021
, Accepted 6th December 2021
First published on 13th December 2021
Abstract
Adansonia digitata L. also known as African baobab is one of the most important fruit-producing trees, widely distributed in the African continent. Baobab fruits are known to possess potential health benefits and nutritional value. This study aimed to holistically dissect the metabolome of A. digitata fruits using a novel comparative protocol using three different analytical platforms. Ultra high performance liquid chromatography coupled to high-resolution tandem mass spectrometry (UHPLC-HRMS/MS), and headspace solid-phase microextraction/gas chromatography coupled to mass spectrometry (HS-SPME/GC-MS) were respectively employed for phytonutrients and aroma profiling, whereas GC-MS post silylation provided an overview of nutrients i.e., sugars. UHPLC-HRMS/MS analysis allowed for the assignment of 77 metabolites, among which 50% are reported for the first time in the fruit. While GC-MS of silylated and aroma compounds led to the identification of 74 and 16 compounds, respectively. Finally, NMR-based metabolite fingerprinting permitted the quantification of the major metabolites for future standardization. In parallel, in vivo antidiabetic potential of the baobab fruit using a streptozotocin (STZ) induced diabetic rat model was assessed. Histopathological and immune-histochemical investigations revealed hepatoprotective and renoprotective effects of A. digitata fruit along with mitigation against diabetes complications. Moreover, the administration of A. digitata fruits (150 mg kg−1) twice a week lowered fasting blood glucose levels.
1. Introduction
African baobab (Adansonia digitata L.) belonging to the family Malvaceae is a large sub-tropical tree widely distributed in Southern, West, and East Africa.1 Baobab is considered an extremely important edible fruit with myriad traditional uses and is widely consumed in African countries owing to its medicinal importance in the treatment of fever, diarrhea, and dysentery. Additionally, it is used as an immuno-stimulant, analgesic, antimalarial, and insect repellent.1 Further, baobab fruit is recognized for its nutritional value and has been approved as a food ingredient in the European continent and the United States of America.1,2
In West Africa, the fruit pulp and seeds are consumed as food and as an ingredient in other food-based products such as sauces, porridges, and beverages.3 Baobab fruit pulp is an essential source of vitamins, minerals, carbohydrates, amino acids, fatty acids, and sterols.3 In addition, baobab is highly rich in vitamin C as it supplies 150–500 mg/100 g fruit so it is useful as a potent antioxidant and about 40 g of baobab pulp consumption daily provides 100% of the daily required vitamin C.4 Baobab fruit is also rich in fibers, calcium, and vitamin B, and is low in proteins and fats.5 The fresh fruit pulp is acidic in taste and consumed in African countries as a snack or as a refreshing juice by adding milk or water. Baobab edible fruits were reported to be enriched in various phytochemical compounds belonging to different chemical classes such as flavonoids, triterpenoids, steroids, vitamins, amino acids, lipids, and carbohydrates.6 Reported biological properties of baobab include anti-hyperglycemic,7 analgesic and antipyretic, antibacterial, anti-inflammatory, antioxidant, and antiviral.5
Recently, modern analytical approaches especially metabolomics platforms have been used in the chemical analysis of food and spices.8 Among analytical tools, UHPLC-HRMS/MS, GC/MS and NMR spectroscopy have been extensively adopted to characterize the metabolite diversity, e.g., volatiles and non-volatiles in fruits and seeds.9,10 Lastly, SPME is considered a convenient solvent-free method that requires minimal sample preparation for the enhancement of volatiles recovery from food samples.11,12
To the best of our knowledge, this is the first report on holistic metabolites profiling of A. digitata L., metabolites using 3 different analytical platforms i.e., UHPLC-HRMS/MS, headspace SPME-GC/MS, GC/MS post silylation, and NMR simultaneously as a novel protocol for the determination of its quality characteristics. Additionally, molecular networks through the Global Natural Products Social Molecular Networking (GNPS) platform was exploited for the propagation of metabolites annotation from the UPLC-HRMS/MS dataset through the imaged structural similarities among the metabolites, with several novel metabolites reported for the first time. Moreover, the in vivo antidiabetic potential of A. digitata fruits was assessed in relation to the metabolites profile in streptozotocin (STZ)-induced diabetic animal model at multiple levels i.e., histopathological examination and biochemical markers.
2. Materials and methods
2.1. Plant material and extract preparation
Adansonia digitata L. fruits (700 g) were purchased from a local market in Khartoum state, Sudan in January 2019. The air-dried powdered fruits (100 g) were extracted by soaking in 500 mL methanol for 48 h. The extract was then filtered and evaporated under reduced pressure at 40 °C and kept in a freezer at −10 °C for further investigation (6 g). A voucher specimen of the fruit material was deposited at the College of Pharmacy Herbarium, Cairo University, Cairo, Egypt.
2.2. Phytochemical investigation
2.2.1. Preparation of fruit extracts for UHPLC-MS analysis. The sample preparation method was performed as mentioned in ref. 13 with slight modifications. Briefly, 2 g of freeze-dried grounded fruits was homogenized with 5 mL 100% MeOH containing 10 μg mL−1 umbelliferone standard (Sigma-Aldrich, St. Louis, MO, US). The extract was then vortexed vigorously and centrifuged at 3000 g for 30 min to remove plant debris. For solid-phase extraction, 500 μL were aliquoted and loaded on a (500 mg) C18 cartridge, which was preconditioned with methanol and water. Samples were then eluted with 3 mL 70% MeOH and 3 mL 100% MeOH. The eluents were evaporated to dryness under a gentle nitrogen stream. The obtained dry residue was re-suspended in 500 μL methanol for further UHPLC-MS analysis.
2.2.2. UHPLC-HRMS/MS analysis. The UHPLC analysis was performed using an Acquity UHPLC System (Waters) equipped with a HSS T3 column (100 × 1.0 mm, particle size 1.8 μm; Waters). The analysis was carried out by applying the following binary gradient at a flow rate of 150 μL min−1: 0–1 min, isocratic 95% A (water/formic acid, 99.9/0.1 [v/v]), 5% B (acetonitrile/formic acid, 99.9/0.1 [v/v]); 1–16 min, linear from 5 to 95% B; 16–18 min, isocratic 95% B; and 18–20 min, isocratic 5% B.14 The injection volume was 3.1 μL (full loop injection). Eluted compounds were detected from m/z 90 to 1000 using a MicroTOF-Q hybrid quadrupole time-of-flight mass spectrometer (Bruker Daltonics) equipped with an Apollo-II electrospray ion source in negative and positive (deviating values in brackets) ion modes using the following instrument settings previously reported.15
2.2.3. UPLC/MS metabolites data extraction and molecular networking. Bruker Daltonics Data Analysis 4.4 was used for UPLC/MS chromatograms inspection. While Metaboscape (Bruker Daltonics) 3.0 was used for data pre-processing using T-ReX 3D (Time aligned Region Complete eXtraction) algorithm for retention time alignment. Where isotopes, adducts, and fragments belonging to the same compound are automatically detected and combined into one feature. Detected features are displayed as a bucket table with their corresponding Rt, measured m/z, molecular weight, and detected fragments. The bucket table was created with an intensity threshold of 10e4 for both negative and positive ionization modes with a retention time range from 0 to 18 min and mass range from 120 to 1600 m/z.16 For the molecular networking and metabolites annotation, the features list created by the Metaboscape was exported as an MGF file for both the positive and negative measurements. The negative and positive mode MGF files were uploaded independently to the GNPS online platform (https://gnps.ucsd.edu), where two molecular networks were created following the online workflow (GNPS 2.0). A molecular network was created with a cosine score above 0.65 and 0.7 for positive and negative modes, respectively, and the number of shared fragments was adjusted to 4. The networks were visualized using Cytoscape 3.5.1.17
2.2.4. SPME and chemicals. SPME fibers of stable flex coated with divinylbenzene/carboxen/polydimethylsiloxane (DVB/CAR/PDMS, 50/30_m) or PDMS (polydimethylsiloxane) were purchased from Supelco (Oakville, ON, Canada). All other chemicals, volatile standards, and sugars were purchased from Sigma Aldrich (St. Louis, MO, USA).
2.2.5. GC-MS analysis of silylated metabolites. Primary metabolite analysis was carried out as follows.11,18 Briefly, 100 mg of finely powdered fruits were extracted with 5 mL 100% methanol with sonication for 30 min and frequent shaking, succeeded by centrifugation at 12
000 × g for 10 min to remove debris. 3 different samples for each A. digitata fruits accession were analyzed under the same conditions. Then, 100 μL of the methanol extract was aliquoted in screw-cap vials and left to evaporate under a nitrogen gas stream until complete dryness. For derivatization, 150 μL of N-methyl-N-(trimethylsilyl)-trifluoroacetamide (MSTFA) previously diluted 1
:
1% with anhydrous pyridine was mixed with the dried methanol extract and incubated for 45 min at 60 °C prior to analysis using GC-MS. Separation of silylated derivatives was achieved on a Rtx-5MS (30 m length, 0.25 mm inner diameter, and 0.25 mm film). The protocol to validate silylation was adopted as previously reported.18
2.2.6. SPME-GC-MS volatile analysis. Dried, finely powdered fruits (20 mg) were placed in SPME screw-cap vials (1.5 mL) spiked with 10 μg (Z)-3-hexenyl acetate with fibers inserted manually above and placed in an oven kept at 50 °C for 30 min. HS-SPME analysis of the volatile compounds was performed as reported in ref. 18 with slight modifications. The fiber was subsequently withdrawn into the needle and then injected manually into the injection port of a gas chromatography-mass spectrometer (GC-MS). GC-MS analysis was adopted on an Agilent 5977B GC/MSD equipped with a DB-5 column (30 m × 0.25 mm i.d. × 0.25 m film thickness; Supelco) and coupled to a quadrupole mass spectrometer. The interface and the injector temperatures were both set at 220 °C. Volatile elution was carried out using the following gradient temperature program: oven was set at 40 °C for 3 min, then increased to 180 °C at a rate of 12 °C min−1, kept at 180 °C for 5 min, finally increased at a rate of 40 °C min−1 to 240 °C and kept at this temperature for 5 min. Helium was utilized as a carrier gas with a total flow rate of 0.9 mL min−1. For ensuring the complete elution of volatiles, SPME fiber was prepared for the next analysis by placing it in the injection port at 220 °C for 2 min. For assessment of biological replicates, three different samples for A. digitata fruits accession were analyzed under the same conditions.10 Blank runs were made during sample analyses. The mass spectrometer was adjusted to EI mode at 70 eV with a scan range set at m/z 40–500.
2.2.7. GC/MS metabolites identification. Identification of volatile and non-volatile silylated components was performed by comparing their retention indices (RI) in relation to n-alkanes (C6–C20), mass matching to NIST, WILEY library database, and with standards if available. Peaks were first deconvoluted using AMDIS software (www.amdis.net (accessed on 28 November 2019))18 before mass spectral matching.
2.3. Antidiabetic activity
2.3.1. Animals and induction of diabetes. All experiments were conducted following the NIH guidelines for animal care and use. Animal use protocol was approved by MSA University, Faculty of Biotechnology Animal Care and Use Committee (Courtesy of Dr Mona Saadeldin, MSA University). Study design and model intiation with the detailed methods were attached in the ESI (S-1).† Pancreatic tissue histological examination after animal termination confirmed diabetes mellitus.19
2.3.2. Histopathology and immunohistochemistry analysis. After 4 weeks of treatment, rats were sacrificed, and samples were collected (ESI, S-2 and S-3†). The liver, kidney, and pancreas tissues were treated as described by ref. 20. The scoring of liver and kidney damage was performed according to previous studies,21 the grade of damage was obtained by adding the entire score of the types of histopathological lesions (4–5 field/rat/200×), as described in ESI Table S1.† Immunohistochemistry analysis of insulin was detected in the pancreatic sections according to the method described.22
2.3.3. Biochemical analysis. Glucose, creatinine, urea, uric acid, cholesterol, HDL, and TG were measured in mg dL−1, whereas ALK, ALT, and AST were measured in IU L−1 (Spectrum Diagnostic, Germany) and detailed biochemical analysis was attached in the ESI (S-4).†
2.4. Statistical analysis
Data are presented as mean ± SD. One-way analysis of variance (ANOVA) followed by Tukey's comparison test was performed to compare parameters among groups. Data were analyzed by using R software (version 3.5.2) for biochemical analysis and parametric histological parameters. For non-parametric data (histological damage score), the statistics are presented as median ± SD using Kruskal–Wallis test, followed by Dunn's Multiple Comparison Test. A P-value of <0.05 was considered to be statically significant.
3. Results and discussion
Functional foods have had always played a pivotal role in developing countries as a potential source of alternative medicine. In Africa, several plants have been used traditionally for the management of diabetes mellitus. The present study aimed to characterize metabolites in baobab fruits via UHPLC/PDA/ESI-MS, and GC-MS techniques. While the quantification of the major metabolites was accomplished through NMR metabolite fingerprinting for future standardization. Further, the antidiabetic potential of A. digitata L. fruit extract in streptozotocin (STZ) induced diabetic rats model was investigated.
3.1. Phytochemical investigation
3.1.1. Secondary metabolites profiling of A. digitata L. fruit via UHPLC-HRMS/MS. In light of the reported biological properties of A. digitata L. fruits, a comprehensive investigation of its metabolites profile was achieved via reversed-phase UPLC/PDA/ESI-qTOF-MS using both positive and negative ionization modes targeting its secondary metabolites. Overlaid UPLC-MS base peak chromatograms (BPC) of A. digitata fruit extract in both ionization modes are shown in Fig. 1.
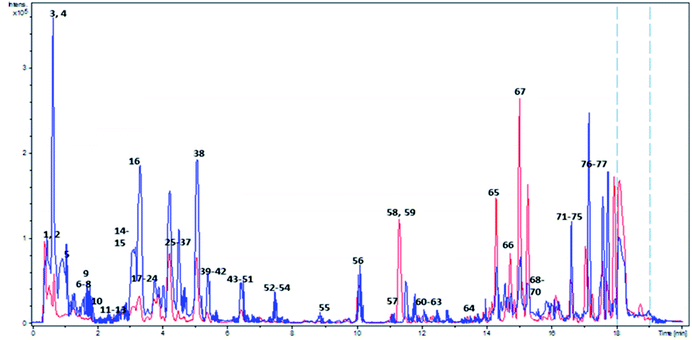 |
| Fig. 1 Overlaid BPC of Adansonia digitata L. fruit crude extract in both ionization modes. Red and blue colors correspond to positive and negative ionization modes respectively. Peaks numbering follow that listed in Table 2. | |
3.1.2. Molecular networking aided dereplication of A. digitata metabolites. Molecular networks assist the visual examination of mass spectrometry dataset, allowing for the propagation of metabolites annotation via the different metabolite classes, similarities among detected metabolites, and allowing for isomers detection. In this study, 2 molecular networks were generated for the positive and negative ionization modes dataset individually through GNPS 2 platform. The positive network had 287 nodes grouped into 26 clusters and 109 single nodes whereas the negative afforded a total of 161 nodes in 13 clusters and 47 isolated nodes (ESI Fig. S1†).Metabolites dereplication was carried out based on their retention times, molecular formula, UV absorption maxima, and their fragmentation pattern in comparison to previously reported data. 77 compounds were annotated belonging to different classes including organic acids, sugars, alcohols, phenolics, coumarins, and fatty acids. Almost 50% of the identified metabolites (40 compounds) are reported for the first time in A. digitata fruits (Table 1).
Table 1 Metabolites identified in A. digitata fruits via UHPLC-MS/MS in 2 negative and positive ionization modes
Peak |
Rt |
Metabolite class |
M − H |
MS2 |
M + H |
MS2 |
Chemical formula |
Error (ppm) |
Name |
References |
Metabolits detected for the first time from Adansonia digitata fruits. |
1 |
0.48 |
Organic acid |
191.0198 |
129, 111 |
|
|
C6H8O7 |
−0.14 |
Citric acid |
67 |
2 |
0.48 |
Organic acid |
133.0142 |
115 |
|
|
C4H6O5 |
0.3 |
Malic acid |
25 |
3 |
0.62 |
Saccharides |
341.1091 |
179, 133 |
343.1234 |
163 |
C12H22O11 |
0.06 |
Disaccharide (sucrose) |
25 |
4 |
0.66 |
Saccharides |
371.1196 |
325, 193 |
|
|
C13H24O12 |
0.16 |
Unknown diasaccharidea |
|
5 |
0.75 |
Organic acid |
191.0200 |
111 |
|
|
C6H8O7 |
−1.87 |
Citric acid isomer |
67 |
6 |
1.0 |
Organic acid |
205.0354 |
111 |
|
|
C7H10O7 |
−0.7 |
Citric acid methyl estera |
23 |
7 |
1.3 |
Saccharides |
|
|
385.1699 |
163, 145 |
C15H28O11 |
1.36 |
Butandiol pentoside hexosidea |
26 |
8 |
1.39 |
Phenolic |
|
|
127.0393 |
109 |
C6H6O3 |
−4.05 |
Pyrogallola |
28 |
9 |
1.53 |
Benzoic acid |
167.0353 |
123 |
|
|
C8H8O4 |
2.05 |
Vanillic acida |
35 |
10 |
1.82 |
Benzoic acid |
153.0193 |
109 |
155.0342 |
111 |
C7H6O4 |
−2.02 |
Dihydroxy benzoic acida (protocatechuic acid) |
34 |
11 |
2.48 |
Nitrogenous compound |
|
|
188.0701 |
|
C11H9NO2 |
2.51 |
Unknown |
|
12 |
2.6 |
Benzenoids |
137.0243 |
109 |
|
|
C7H6O3 |
−2.6 |
Dihydroxybenzaldehydea |
29 |
13 |
2.84 |
Organic acid |
175.0615 |
131, 157 |
|
|
C7H12O5 |
−1.8 |
Hydroxyglutaric acida |
24 |
14 |
3.32 |
Saccharides derivatives |
|
|
369.1753 |
295, 163, 145, 133, 115 |
C15H28O10 |
0.38 |
Butanol hexopyranosyl-deoxypyranosidea |
27 |
15 |
3.32 |
Saccharides derivatives |
|
|
295.1012 |
163, 145, 133 |
C11H18O9 |
2.55 |
Dihydroxy—methylenebutanoate-O-hexosidea |
Tuliposide B68 |
16 |
3.39 |
Flavan-3-ol |
289.0717 |
245, 203 |
291.0861 |
207, 139 |
C15H14O6 |
2.42 |
Catechin/epicatechin |
34 and 67 |
17 |
3.5 |
Cinnamic acid ester |
353.0891 |
191 |
|
|
C16H18O9 |
−2.1 |
O-Caffeoyl quinic acid ester clorogenic acid |
34 |
18 |
3.53 |
Nitrogenous compound |
352.1034 |
191 |
|
|
C16H19NO8 |
0.85 |
Unknowna |
|
19 |
3.72 |
Cinnamic acid ester |
281.0660 |
193, 163, 145 |
|
|
C13H14O7 |
4.39 |
O-feruloyl lactic acid estera |
36 |
20 |
3.79 |
Proanthocyanidin |
577.1345 |
407, 289 |
|
|
C30H26O12 |
−0.98 |
Procyanidn dimer isomer |
Xx34,67 |
21 |
3.8 |
Cinnamic acid glycoside |
327.1082 |
165, 147 |
|
|
C15H20O8 |
2.8 |
Dihydrocoumaroyl O-hexoside (dihydromelilotoside)a |
39 |
22 |
3.9 |
Proanthocyanidin |
577.0346 |
407, 289 |
579.1472 |
409, 301, 291 |
C30H26O12 |
4.25 |
Procyanidn dimer isomer I |
34 and 67 |
23 |
3.91 |
Proanthocyanidin |
865.1987 |
|
|
289 |
C45H38O18 |
0.2 |
Procyanidin trimer isomer I |
34 and 67 |
24 |
3.98 |
Cinnamic acids glycosides |
341.0893 |
179 [M-H-162], 161 |
|
|
C15H18O9 |
4.5 |
Caffeoyl–O-hexoside |
40 |
25 |
4.03 |
Saccharides derivatives |
|
|
295.1018 |
163, 145, 133 |
C11H18O9 |
1.88 |
Dihydroxy–methylenebutanoate–O-hexoside isomer |
68 |
26 |
4.1 |
Cinnamic acid glycosides |
487.1457 |
193[M − H − 132-162] |
|
|
C21H28O13 |
−1.3 |
Feruloyl O-pentosyl hexosidea |
41 |
27 |
4.23 |
Cinnamic acids |
|
|
165.0544 |
119, 107 |
C9H8O3 |
3.67 |
Coumaric acid |
34 |
28 |
4.24 |
Flavan-3-ol |
289.0716 |
245, 203 |
291.0860 |
|
C15H14O6 |
−0.27 |
Catechin/epicatechin |
34 and 67 |
29 |
4.33 |
Saccharides derivatives |
|
|
295.1018 |
163, 145, 133 |
C11H18O9 |
1.9 |
Dihydroxy-methylenebutanoic acid-O-hexoside |
68 |
30 |
4.47 |
Cinnamic acid glycoside |
325.0925 |
163 |
|
|
C15H18O8 |
−0.29 |
Coumaroyl-O-hexosidea |
41 |
31 |
4.52 |
Saccharides derivatives |
|
|
383.1905 |
163, 145, 133, 155 |
C16H30O10 |
1.7 |
Methylbutyl-O-pentosyl-hexosidea |
68 |
32 |
4.52 |
Saccharides derivatives |
|
|
295.1008 |
163, 145, 133 |
C11H18O9 |
0.9 |
Dihydroxy-methylenebutanoic acid-O-hexoside |
68 |
33 |
4.52 |
Proanthocyanidin |
865.1992 |
577, 407, 289 |
|
|
C45H38O18 |
1.03 |
Procyanidin trimer isomer II |
Xx34,67 |
34 |
4.7 |
Proanthocyanidin |
865.1994 |
|
867.1867 |
579, 289 |
C45H38O18 |
0.77 |
Procyanidin trimer isomer |
Xx34,67 |
35 |
4.73 |
Cinnamic acid ester selflooped |
367.1024 |
191, 173, 133 |
|
|
C17H20O9 |
−2.77 |
O-feruloyl quinic acid |
Xx67 |
36 |
4.8 |
Phenolic |
461.1653 |
149 |
|
|
C20H30O12 |
4.5 |
Homovanillyl alcohol-O-pentosyl hexosidea |
30 |
37 |
4.8 |
Cinnamic acid |
163.0401 |
119 |
|
|
C9H8O3 |
−0.1 |
Coumaric acid isomer |
34 |
38 |
5.02 |
Proanthocyanidin |
1153.2595 |
865, 577, 289 |
|
|
C60H50O24 |
−2.04 |
Procyanidin tetramer |
34 |
39 |
5.1 |
Cinnamate |
|
|
163.0391 |
135 |
C9H8O3 |
−0.5 |
Unknowna |
|
40 |
5.49 |
Cinnamic acid ester |
367.1031 |
191, 173, 133 |
|
|
C17H20O9 |
−0.52 |
O-feruloyl quinic acid isomer |
67 |
41 |
5.59 |
Furanochromones |
|
|
409.1111 |
247 |
C19H20O10 |
4.37 |
Khellol-O-hexosidea |
46 |
42 |
5.66 |
Flavonoid |
609.1457 |
301 |
|
|
C27H30O16 |
−0.65 |
Quercetin-O-deoxyhexosyl hexoside |
67 |
43 |
6.2 |
Cinnamic acid glycoside |
325.0916 |
163 |
|
|
C15H18O8 |
−3.57 |
Coumaroyl-O-hexoside |
40 |
44 |
6.27 |
Flavonoid |
609.1467 |
301 |
|
|
C27H30O16 |
1.04 |
Quercetin-O-deoxyhexosyl hexoside |
67 |
45 |
6.43 |
Proanthocyanidin |
577.1346 |
407, 289 |
|
|
C30H26O12 |
−0.93 |
Procyanidn dimer isomer III |
34 and 67 |
46 |
6.46 |
Flavonoid |
|
|
449.1073 |
287 |
C21H20O11 |
1.17 |
Kaempferol–O-hexoside |
67 |
47 |
6.44 |
Flavonoid |
593.1505 |
447, 285 |
595.1617 |
449, 287 |
C27H30O15 |
1.04 |
Kaempferol–O-deoxyhexosyl hexosidea |
43 |
48 |
6.47 |
Flavonoid |
|
|
287.0543 |
217, 151 |
C15H10O6 |
3.07 |
Kaempferol |
67 |
49 |
6.52 |
Flavonoid |
593.1505 |
447, 285 |
|
|
C27H30O15 |
−2.46 |
Kaempferol–O-deoxyhexosyl hexoside isomera |
43 |
50 |
6.62 |
Cinnamic acid ester |
293.0671 |
163, 145, 119 |
|
|
C14H14O7 |
1.28 |
O-coumaroyl malic acid methyl estera |
37 |
51 |
6.88 |
Cinnamic acid glycosides |
355.1022 |
193 |
|
|
C16H20O9 |
−3.48 |
Feruloyl–O-hexoside |
40 |
52 |
7.3 |
Cinnamic acid amide |
326.1032 |
206, 163, 145 |
|
|
C18H17NO5 |
−0.52 |
Coumaroyl–N-tyrosinea |
38 |
53 |
7.5 |
Cinnamic acid |
279.0870 |
163 |
|
|
C14H16O6 |
1.6 |
Unknowna |
|
54 |
7.63 |
Flavonoid |
447.09314 |
285 |
|
|
C21H20O11 |
0.34 |
Kaempferol-O-hexoside |
67 |
55 |
8.9 |
Megastigmane |
405.2123 |
225 |
|
|
C19H34O9 |
3.8 |
Megastigmane–O-hexosidea |
69 |
56 |
10.1 |
Steroid |
507.2582 |
345 |
|
|
C27H40O9 |
0.9 |
Unknown |
|
57 |
11.2 |
Phenolic |
149.0605 |
122 |
|
|
C9H10O2 |
−1.68 |
Allyl catechola |
31 |
58 |
11.32 |
Furanochromones |
|
|
261.0753 |
230 |
C14H12O5 |
0.36 |
Khellina |
70 |
59 |
11.38 |
Furanochromones |
|
|
231.0648 |
203, 216 |
C13H10O4 |
1.35 |
Visnagina |
70 |
60 |
12.29 |
Phenolic |
|
|
137.0600 |
122, 109 |
C8H8O2 |
−2.2 |
Methoxy benzaldehyde |
32 |
61 |
12.31 |
Phenolic |
|
|
133.0651 |
105 |
C9H8O |
2.94 |
Cinnamaldehyde |
33 |
62 |
12.32 |
Phenolic |
|
|
165.0907 |
137, 124 |
C10H12O2 |
1.63 |
Eugenol |
32 |
63 |
13.6 |
Cinnamic acid |
|
|
207.1017 |
165, 150 |
C12H14O3 |
−0.9 |
Unknowna |
|
64 |
13.72 |
Cinnamic acid |
|
|
211.0961 |
193, 181, 165 |
C11H14O4 |
1.55 |
Unknowna |
|
65 |
14.97 |
Coumarins |
|
|
411.1410 [M + Na] |
329, 245, 227, 199 |
C21H24O7 |
0.81 |
Isovaleryl-acetyl khellactone (dihydrosamidin)a |
47 |
66 |
14.98 |
Coumarins |
|
|
329.1377 |
245, 227 |
C19H20O5 |
1.9 |
Decursina |
51 |
67 |
15.1 |
Fatty acid |
295.2274 |
277 (−H2O), 233 (−CO2) |
|
|
C18H32O3 |
1.3 |
Hydroxyoctadecadienoic acida |
71 |
68 |
15.1 |
Fatty acid |
271.2278 |
253 |
|
|
C16H32O3 |
1.2 |
Hydroxyhexadecanoic acida |
72 |
69 |
15.4 |
Fatty acid |
269.2108 |
251 |
|
225 |
C16H30O3 |
5.1 |
Oxohexadecanoic acida |
73 |
70 |
16.1 |
Fatty acid |
295.2271 |
277 (−H2O), 233 (−CO2) |
|
|
C18H32O3 |
−3.1 |
Hydroxyoctadecadienoic acida |
74 |
71 |
16.1 |
Fatty acid |
297.2423 |
|
|
|
C18H34O3 |
|
Hydroxy-octadecanoic acida |
71 |
72 |
16.3 |
Fatty acid |
311.2225 |
293 (−H2O), 275 (−H2O), 223 |
|
|
C18H32O4 |
1.1 |
Dihydroxy-octadecadienoic acida |
74 |
73 |
16.6 |
Fatty acid |
277.2171 |
259 |
|
|
C18H30O2 |
1.8 |
Octadecatrienoic acida |
75 |
74 |
16.93 |
Fatty acid |
355.3216 |
309 |
|
|
C22H44O3 |
0.22 |
Hydroxydocosanoic acida |
72 |
75 |
17.1 |
Fatty acid |
279.2331 |
261 |
|
|
C18H32O2 |
−0.6 |
Octadecadienoic acid (linoleic acid) |
75 |
76 |
17.5 |
Fatty acid |
279.2331 |
261 |
|
|
C18H32O2 |
−1.8 |
Octadecadienoic acid (linoleic acid) |
75 |
77 |
17.6 |
Fatty acid |
255.2333 |
|
|
|
C16H32O2 |
−0.8 |
Hexadecanoic acid (palmitic acid) |
76 |
3.1.2.1. Organic acids. Citric acid and its isomer were detected in peaks 1 & 5 with [M − H]− at m/z 191.0198, C6H8O7, and characteristic fragment at m/z 111. The negative molecular network (ESI Fig. S1,† cluster a) revealed a directly correlated ion at peak 6 [M − H]− 205.0354, C7H10O7, with a mass difference of 14 Da (CH2) and was annotated as citric acid methyl ester.23Other organic acids included peak 2 with [M − H]− 133.0142, C4H6O5 annotated as malic acid, and peak 13 with [M − H]− 175.0615, C7H12O5.24
3.1.2.2. Saccharides and their derivatives. Cluster b of the negative spectral network (ESI Fig. S1†) compromised sugars and their derivatives from which peaks 3 & 4 corresponded to two disaccharides, where 3 had a molecular ion [M − H]− at m/z 341.1107, C12H22O11 and was assigned as sucrose previously identified in Adansonia fruits.25Butanediol-O-pentosyl-hexoside, peak 7 in the positive mode, showed a molecular ion at m/z 385.1699 [M + H]+, C15H28O11.26 Similarly, peak 14 showed a molecular ion at m/z 369.1753 [M + H]+, C15H28O10 and a fragment at m/z 295 for the loss of C4H9O was assigned as butanol-pentosyl-hexoside.27
Additionally, the positive molecular network revealed the presence of 4 isomers of acylated hexoses; peaks 15, 25, 29 & 32 which shared a molecular ion at m/z 295.1018 [M + H]+ and corresponded to the elemental composition of C11H18O9.
3.1.2.3. Benzenoids & aromatics. Several phenolic metabolites were detected in both ionization modes and revealed themselves mostly as single nodes within both spectral networks. The first eluted at peak 8 was observed at [M + H]+ at m/z 127.0393, C6H6O3 with a fragment at m/z 109 for H2O loss and was annotated as pyrogallol.28 Following at peak 12, was dihydroxybenzaldehyde with [M − H]− at m/z 137.0243, C7H6O3 and a characteristic fragment at m/z 109 for the cleavage of CO.29 Lately eluted, peak 36 exhibited a molecular ion at m/z 461.1653 [M − H]− and elemental composition of C20H30O12 and showing a daughter ion at m/z 149 [M − H − 162 − 132]− corresponding to the loss of a hexoside and pentoyl moieties.30 Hence it was annotated as homovanillyl alcohol-O-pentosyl–O-hexoside. While peak 57 showed a molecular ion at m/z 149.0605 [M − H]−, C9H10O2 and a fragment at m/z 122 [M − H − C2H2]− was assigned as allylcatechol.31Methoxybenzaldehyde, eluted in peak 60 with a molecular ion at m/z 137.0600 [M + H]+, C8H8O2 and fragment ion at m/z 122 and 109 for the consecutive cleavage of CH3 and CO.32 Similarly, peaks 61 & 62 with [M − H]+ at m/z 133.0651, C9H8O, & 165.0907, C10H12O2 and fragments at m/z 105 & 137 accounting for the loss of CO and were ascribed as cinnamaldehyde33 and eugenol,32 respectively.
The presence of benzoic acids in A. digitata fruits was previously reported34 and was firstly observed in peak 9 assigned as vanillic acid from its molecular ion [M − H]− at m/z 167.0353, C8H8O4 and fragment at m/z 123 [M − H − 44]− for the loss of CO2.35 Likewise, dihydroxybenzoic acid in peak 10 confirmed by its molecular ion at m/z 153.0193 [M − H]−, C7H6O4 and fragment at m/z 109 for the loss of CO2.
3.1.2.4. Hydroxy cinnamates. Peaks 27 & 37 were annotated as hydroxyl cinnamic acid, p-coumaric acid isomers as concluded from their molecular ions at m/z 163.0401 [M − H]−, 165.0544 [M + H]+ and formula of C9H8O3.34 Quinic acid esters were detected in peaks 17 annotated as O-caffeoyl quinic acid ester (chlorogenic acid) and 35 & 40 as two isomers of O-feruloyl quinic acid. Peak 17 [M − H]−at m/z 353.0891, C16H18O9 and a base peak at m/z 191 suggestive for O-caffeoyl quinic acid ester (chlorogenic acid).34 While peaks 35 & 40 showed same molecular ion at m/z 367.1024 [M − H]−, C17H20O9 and a base peak at m/z 191 confirming them to be isomers of O-feruloyl quinic acid esters.Other identified cinnamate esters included peak 19 annotated as O-feruloyl lactic acid ester based on its [M − H]− at m/z 281.0660, C13H14O7, and daughter ions at m/z 193 for the loss of lactic acid moiety (C3H6O3).36 Peak 50 with a [M − H]−at m/z 293.0671 [M − H]−, C14H14O7 and fragment ions at m/z 163 [M − H − 130]− for the cleavage of C5H6O4 (methyl malic acid) and m/z 145 & 119 for dehydration and decarboxylation was annotated as O-p-coumaroyl malic acid methyl ester.37 Whereas, coumaroyl tyrosine conjugate was detected in peak 52 with [M − H]−at m/z 326.1032, and fragments at m/z 206 for tyrosine and subsequent fragments at m/z 163 and 145 for deamidation and dehydration.38
Cinnamic acid glycosides in peaks 21, 24, 30, 43, 51, all showed fragment ions [M − H − 162]− indicative of a hexoside moiety. Peak 21 was annotated as dihydrocoumaroyl O-hexoside (dihydromelilotoside) with [M − H]−at m/z 327.1082, C15H20O8,39 and peak 24 as caffeoyl acid O-hexoside showing a molecular ion at m/z 341.0893 [M − H]−, C15H18O9.40 Ferulic acid O-hexoside was observed in peak 51 with molecular ion at m/z 355.1022 [M − H]−, C16H20O9.41 Additionally, feruloyl O-pentosyl hexoside peak 26 assignment was based on [M − H]− at m/z 487.1457, C21H28O13 and fragment at m/z 193 corresponding to the loss of a hexoside and pentoside moieties.41
3.1.2.5. Proanthocyanidin and their monomers flavan-3-ol. Monomeric proanthocyanidins were detected in peaks 16 & 28 with molecular ions at m/z 289.0716 [M − H]−, 291.0860 [M + H]+, C15H14O6, assigned as catechin/epicatechin.34 In contrast, their dimeric procyanidins were observed in peaks 20, 22 & 45 with molecular ions at m/z 577.1346, C30H26O12 with fragments at m/z 407, and 289 which are characteristic of type B proanthocyanidin previously reported in A. digitata fruits.34 While, isomers of proanthocyanidin trimers were detected in peaks 23, 33 & 34 exhibiting molecular ions at m/z 865.1992, C45H38O18 and distinctive fragments at m/z 577, 407, 289.34 A procyanidin tetramer was recognized in peak 38 with a molecular ion at m/z 1153.2595 [M − H]−, following the same fragmentation pattern as the dimers and trimers.34 All detected proanthocyanidins were grouped in the negative molecular network as group c (ESI Fig. S1†) except for the tetramer which appeared as a self-looped node.Proanthocyanidins are well known for their antidiabetic potential and to protect against diabetic complications to include oxidative stress, lipid peroxidation, diabetic encephalopathy, and retinopathy.42
3.1.2.6. Flavonoids. Aside from the flavan-3-ols, flavonols and their glycosides were depicted in peaks 42, 44, 46, 47, 48, 49 & 54. Quercetin-O-deoxyhexosyl hexoside isomers, 42 & 44 with a molecular ion at m/z 609.1457 [M − H]−, C27H30O16 showed the characteristic fragmentation for the loss of a hexoside and deoxyhexoside moieties to yield aglycone moiety at m/z 301.34 Similarly, isomers of kaempferol-O-deoxyhexosyl hexoside were detected in peaks 47 & 49 with m/z 593.1505 [M − H]−, C27H30O15 and fragments at m/z 447 and 285.43 While, peaks 46 & 54 were annotated as kaempferol-O-hexoside isomers at m/z 447.09314, C21H20O11 and fragment at m/z 285. Several studies have affirmed the potential of dietary flavonoids in diabetes management through improving glucose metabolism and the lipid profile, thus protecting against diabetes and its aggravations.44
3.1.2.7. Furano- & pyrano-coumarins. Furanochromones were observed for the first time in peaks 41, 58 & 59 as proved from their molecular ions and fragmentation pattern detected solely in the positive ionization mode.45Peak 41 detected in the positive ionization mode exhibited [M + H]+ at m/z 409.1111, C19H20O10 and a distinct fragment at m/z 247 for the loss of a hexoside moiety,46 annotated as khellol-O-hexoside. While, peaks 58 & 59 with molecular ions at m/z 261.0753 [M + H]+, C14H12O5 and m/z 231.0648 [M + H]+, C13H10O4 were annotated as khellin and visnagin, respectively as concluded from their fragmentation pattern.
Likewise, pyranocoumarins were also detected for the first time in A. digitata fruits. An acylated derivative of the pyranocoumarin, khellactone was recognized at peak 65 as a Na adduct at m/z 411.1410 corresponding to C21H24O7 as previously detected by.47 Its MS2 spectrum showed fragments at m/z 329 [M + Na − C2H3O2]+ for the cleavage of an acetic acid moiety, m/z 245 for the consecutive loss of an isovaleryl moiety (C5H8O) followed by m/z 227 and 199 for the elimination of H2O and CO. Consequently, it was annotated as isovaleryl-acetyl khellactone.47 O-Substituted esters of khellactone are reported to exhibit numerous biological activities i.e., anti-obesity,48 anti-HIV,49 and anti-cancer50 activities.
Similarly, peak 66 found in the positive ionization mode, with molecular ion at m/z 329.1377, C19H20O5 was annotated as decursin following its fragmentation pattern with fragment ions at m/z 245 [M + H − C5H8O]+.51
3.1.3. Primary metabolites profiling of A. digitata fruits via GC-MS post-silylation. To provide an overview of primary metabolites accounting for fruits' nutritional value, GC-MS analysis was employed post-silylation. A total of 74 peaks (Table 2 and Fig. 2) were annotated, including nitrogenous compounds, organic acids, sugars (mono- and disaccharides), sugar alcohols, and fatty acids, in addition to minor sterols and phenolics.
Table 2 The relative percentage of silylated metabolites in A. digitata fruits analyzed via GC-MS, n = 3
No |
Class |
RT |
KI |
Name |
Relative percentile (n = 2) |
1 |
Nitrogenous compounds |
8.203 |
1135.3 |
Hydroxylamine (3TMS) |
0.32 ± 0.14 |
2 |
11.4365 |
1264.4 |
Urea (2TMS) |
0.023 ± 0.01 |
3 |
13.7775 |
1355.4 |
Uracil (2TMS) |
0.001 ± 0.0 |
4 |
18.5075 |
1545.7 |
4-Aminobutanoic acid (3TMS) |
0.35 ± 0.07 |
5 |
25.5705 |
1864.6 |
N-Acetyl-galactosamine (TMS) |
1.09 ± 0.46 |
6 |
35.3 |
2357.7 |
9-Octadecenamide (TMS) |
2.55 ± 0.26 |
7 |
35.7485 |
2380.5 |
5-Methyluridine, (3TMS) |
0.169 ± 0.12 |
8 |
37.4285 |
2466 |
(Z)-Docos-9-enenitrile (TMS) |
2.369 ± 0.01 |
9 |
41.7965 |
2688.2 |
13-Docosenamide (TMS) |
1.97 ± 0.37 |
Total nitrogenous compounds |
8.842 |
10 |
Acids |
11.588 |
1270.3 |
Benzoic acid (TMS) |
0.76 ± 0.34 |
11 |
12.9275 |
1322.4 |
Maleic acid, (2TMS) |
0.17 ± 0.16 |
12 |
13.5745 |
1347.5 |
Glyceric acid, (3TMS) |
0.094 ± 0.06 |
13 |
13.905 |
1360.3 |
Itaconic acid, (2TMS) |
0.19 ± 0.07 |
14 |
14.134 |
1369.2 |
Fumaric acid, (2TMS) |
0.27 ± 0.21 |
15 |
14.403 |
1379.6 |
Nonanoic acid (TMS) |
0.114 ± 0.04 |
16 |
15.5195 |
1423.7 |
Pentanedioic acid (2TMS) |
0.0155 ± 0.01 |
17 |
17.5605 |
1506 |
Malic acid, (3TMS) |
4.80 ± 2.5 |
18 |
21.061 |
1655.6 |
Tartaric acid (4TMS) |
0.71 ± 0.22 |
19 |
23.601 |
1770.6 |
Aconitic acid (3TMS) |
0.90 ± 0.088 |
20 |
24.961 |
1835 |
Shikimic acid (4TMS) |
1.00 ± 0.23 |
21 |
25.067 |
1840.2 |
Citric acid (3TMS) |
2.51 ± 3.55 |
22 |
25.818 |
1876.6 |
Quininic acid (5TMS) |
0.994 ± 0.23 |
Total acids |
12.52 |
23 |
Amino acids |
11.775 |
1277.6 |
L-Serine (3TMS) |
0.17 ± 0.02 |
24 |
12.677 |
1312.7 |
L-Threonine (2TMS) |
0.054 ± 0.01 |
25 |
15.9605 |
1441.4 |
L-Aspartic acid (3TMS) |
0.68 ± 0.23 |
26 |
18.2905 |
1536.6 |
Pyroglutamic acid (3TMS) |
0.67 ± 0.04 |
27 |
18.642 |
1551.4 |
L-Glutamic acid (3TMS) |
0.11 ± 0.05 |
Total amino acids |
1.69 |
28 |
Sugars |
15.212 |
1411.4 |
Ribonic acid (4TMS) |
0.097 ± 0.01 |
29 |
19.2745 |
1577.9 |
Erythronic acid (4-TMS) |
0.64 ± 0.15 |
30 |
20.551 |
1633.1 |
Ribofuranose, (4TMS) |
0.12 ± 0.03 |
31 |
20.801 |
1644.1 |
Xylonic acid, (5TMS) |
0.60 ± 0.03 |
32 |
21.6245 |
1680.4 |
Arabinose, (4TMS) |
0.48 ± 0.19 |
33 |
22.9725 |
1741.7 |
Rhamnose (4TMS) |
0.30 ± 0.12 |
34 |
23.2415 |
1754.1 |
Ribono-1,4-lactone (3TMS) |
0.21 ± 0.01 |
35 |
24.4295 |
1809.3 |
Fructofuranoside (5TMS) |
2.52 ± 1.85 |
36 |
25.9845 |
1884.7 |
Galactopyranose (5TMS) |
0.40 ± 0.07 |
37 |
26.118 |
1891.2 |
Fructose (5TMS) |
5.19 ± 0.83 |
38 |
26.398 |
1904.9 |
Arabinose (4TMS) isomer |
6.47 ± 2.69 |
39 |
26.554 |
1912.9 |
Galactopyranose (5TMS) isomer |
0.14 ± 0.03 |
40 |
26.730 |
1921.8 |
Glucose (5TMS) |
12.16 ± 0.08 |
41 |
27.030 |
1937.1 |
Glucose (5TMS) |
3.18 ± 4.5 |
42 |
27.161 |
1943.8 |
Glucono-1,4-lactone (4TMS) |
0.14 ± 0.19 |
43 |
27.399 |
1955.9 |
Allose (5TMS) |
0.43 ± 0.02 |
44 |
27.532 |
1962.6 |
Galactaric acid (6TMS) |
1.43 ± 0.16 |
45 |
28.3115 |
2002.3 |
Talose (5TMS) |
1.86 ± 0.49 |
46 |
28.566 |
2015.2 |
Gluconic acid (6TMS) |
0.05 ± 0.06 |
47 |
37.7745 |
2483.6 |
Sucrose (8TMS) |
0.66 ± 0.43 |
48 |
42.444 |
2721.1 |
Sedoheptulose anhydride (4TMS) |
0.25 ± 0.18 |
49 |
43.6855 |
2784.3 |
Lactose (8TMS) |
0.51 ± 0.38 |
50 |
44.253 |
2813.1 |
Melibiose (8TMS) |
2.88 ± 0.15 |
51 |
44.4365 |
2822.5 |
Galactinol (9TMS) |
4.07 ± 1.54 |
52 |
44.5525 |
2828.4 |
Melibiose (8TMS) isomer |
2.22 ± 2.60 |
Total sugars |
47.00 |
53 |
Sugar alcohol |
17.834 |
1517.5 |
Threitol (4TMS) |
0.11 ± 0.04 |
18.017 |
1525.2 |
Threitol (4TMS) isomer 1 |
0.21 ± 0.08 |
54 |
21.624 |
1680.4 |
Threitol (4TMS) isomer 2 |
0.17 ± 0.23 |
55 |
27.262 |
1948.9 |
Mannitol (6TMS) |
6.17 ± 8.39 |
56 |
29.185 |
2046.7 |
Myo-inositol (6TMS) |
1.09 ± 1.55 |
57 |
30.361 |
2106.5 |
Myo-inositol (6TMS) isomer |
4.60 ± 0.62 |
58 |
36.0015 |
2393.4 |
Myo-inositol, (5TMS) phosphate |
0.87 ± 0.25 |
Total sugar alcohols |
13.22 |
59 |
Phenolics |
18.6975 |
1553.7 |
Pyrogallol (3TMS) |
0.032 ± 0.0 |
60 |
20.194 |
1617.3 |
Pyrogallol (3TMS) (isomer) |
0.07 ± 0.07 |
61 |
42.7805 |
2738.2 |
Catechin (5TMS) |
4.90 ± 0.06 |
Total phenolics |
5.0 |
62 |
Fatty acids/glycerides |
29.6455 |
2070.1 |
Palmitic acid (TMS) |
0.65 ± 0.91 |
63 |
32.77 |
2229 |
Oleic acid (TMS) |
0.92 ± 0.04 |
64 |
33.248 |
2253.4 |
Stearic acid (TMS) |
0.45 ± 0.1 |
65 |
36.0975 |
2398.3 |
Oleamide (TMS) |
1.06 ± 1.09 |
66 |
36.505 |
2419 |
Stearamide (TMS) |
0.80 ± 0.34 |
67 |
38.8645 |
2539 |
Monopalmitin (2TMS) |
1.24 ± 0.11 |
68 |
42.5505 |
2726.5 |
Lignoceric acid (TMS) |
0.08 ± 0.05 |
Total fatty acids/glycerides |
5.2 |
69 |
Steroids/terpenes |
44.6665 |
2834.2 |
α-Tocopherol (TMS) |
0.83 ± 0.22 |
70 |
48.153 |
3011.5 |
Campesterol (TMS) |
0.25 ± 0.02 |
71 |
48.557 |
3032 |
Stigmasterol (TMS) |
0.15 ± 0.03 |
72 |
49.603 |
3085.2 |
Stigmast-5-ene (TMS) |
2.13 ± 0.22 |
Total steroids/terpenes |
3.36 |
73 |
Inorganics |
12.1065 |
1290.5 |
Phosphoric acid (3TMS) |
2.94 ± 0.83 |
Total inorganics |
2.94 |
74 |
Lactones |
|
|
Dihydro-2(3H)-furanone (2TMS) |
0.01 ± 0.02 |
Total lactones |
0.01 |
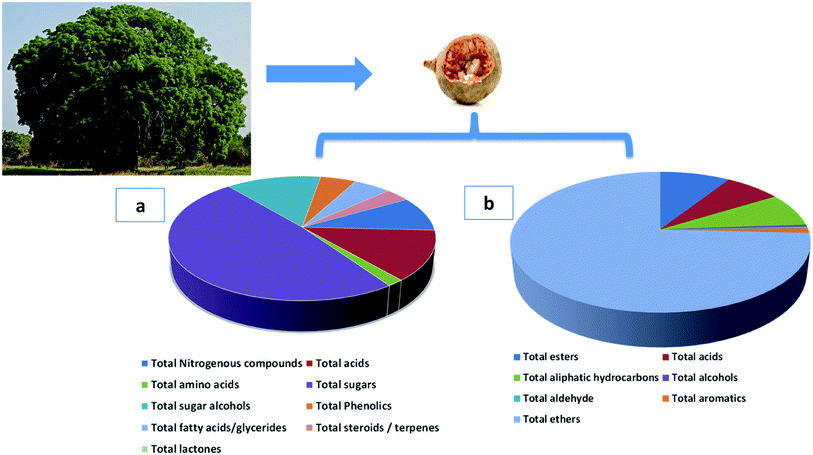 |
| Fig. 2 Adansonia digitata tree and its fruit and pie charts of different metabolites classes. (a) The major non-volatile metabolites groups identified by GC-MS/MS post-silylation (b) the groups of identified aroma metabolites in A. digitata fruits using HS-SPME-GC-MS/MS. | |
3.1.3.1. Sugars/sugar alcohols. Sugars, mostly mono-saccharides represented the most dominant primary metabolite class at ca. 47% of total detected metabolites, comprising 25 different sugars. Glucose (peaks 40 and 41) was the main sugar (15%) detected in baobab fruit followed by arabinose (peak 38) and fructose (peak 37) detected at 6.5 and 5.2%, respectively. Such high level of glucose and other monosaccharides accounts for baobab fruits sweet taste. In contrast, major disaccharides in baobab were found to be melibiose (peak 50 and 52) and galactinol (peak 51) to amount for ca. 5% of the total sugars. Galactinol is one of the raffinose family oligosaccharides that was identified in baobab fruit, with potential antioxidant action.52Sugar alcohols amounted for 13.2% of baobab fruit total metabolites detected using GC/MS. Mannitol (6.2%) and myo-inositol amounted for the major sugar alcohols in the fruit. Myo-inositol (peaks 56 and 57), the most abundant inositol isomer in cereals, and fruits, improves insulin sensitivity.53 Consequently, baobab fruit could represent a potential functional food for diabetic patients based on these results.
3.1.3.2. Organic acids. Baobab fruit showed a considerable level of organic acids to account for the fruits acidic taste (12.5%) represented by malic acid (4.8%) and citric acid (2.5%). Malic acid is a common acid in unripe fruit, is used to enhance beverages flavor and to act as a food acidulant.54
3.1.3.3. Nitrogenous compounds/amino acids. Nitrogenous compounds were detected in A. digitata fruit at 8.8% represented mostly by 9-octadecenamide (peak 6) and docos-9-enenitrile (peak 8) at 2.55 and 2.37%, respectively. N-Acetyl-D-galactosamine (peak 5), an amino sugar derivative of galactose was detected in baobab fruit which plays a role in mucin formation, aside for its effect on immune system and cancer.55 The low amino acids level in baobab fruit (1.7%) suggests for its low pool in free amino acids, represented by pyroglutamic (peak 26), aspartic (peak 25), and glutamic (peak 27) acids.
3.1.3.4. Fatty acids/acyl esters/sterols. Fatty acids were detected in baobab fruit reaching 5.2% represented mostly by palmitic and oleic acids. Concerning fatty acyl esters, 1-monopalmitin (peak 67) was detected at 1.2%. Minor steroids were detected in baobab fruit to reach 3.36%, with stigmast-5-ene (peak 72, 2.13%) was found to be the dominant steroid.
3.1.3.5. Inorganic acids. Phosphoric acid (peak 73) was the only inorganic detected in A. digitata fruits at (2.9%). Phosphoric acid was reported to improve the antioxidant activity of tocopherols, aside from functioning as food preservative.56
3.1.3.6. Phenolics. Another considerable metabolite class detected in A. digitata fruits were phenolics detected at 5%. The major identified phenolics included catechin (peak 61), also detected using LC/MS. Catechin, a flavan-3-ol derivative common with antioxidant, antihyperlipidemic, and antidiabetic activities, and likely to account for fruit effects.57
3.1.4. Aroma analysis of A. digitata fruits using headspace SPME-GC-MS. A. digitata was subjected to volatile analysis likely to account for its organoleptic characters. HS-SPME led to the identification of 16 volatiles belonging to organic acids, alcohols, aldehydes, esters, ketones, aliphatic hydrocarbons, aromatics, and ethers (Table 3 and Fig. 2).
Table 3 The relative percentage of volatile constituents in A. digitata fruits analyzed using SPME–GC-MS, n = 2
No |
Class |
RT |
KI |
Name |
Relative percentile |
1 |
Ester |
2.095 |
398 |
Acetic acid, methyl ester |
0.45 ± 0.0 |
2 |
11.8654 |
1468 |
Terpinene 4-acetate |
0.57 ± 0.0 |
3 |
16.1491 |
1916 |
Hexadecanoic acid methyl ester |
8.05 ± 0.0 |
Total esters |
9.07 |
4 |
Acids |
2.3652 |
430 |
Acetic acid |
6.80 ± 0.0 |
5 |
8.9189 |
1166 |
Octanoic acid |
0.34 ± 0.0 |
Total acids |
7.14 |
6 |
Aliphatic hydrocarbon |
5.3152 |
784 |
1,3-Butadiene, 2,3-dimethyl |
3.17 ± 0.0 |
7 |
7.1919 |
1007 |
2,4-Hexadiene |
1.81 ± 0.0 |
8 |
9.283 |
1200 |
Dodecane |
0.57 ± 0.0 |
9 |
11.163 |
1399 |
Tetradecane |
0.68 ± 0.0 |
10 |
12.0777 |
1489 |
Pentadecane |
0.79 ± 0.0 |
11 |
13.2017 |
1598 |
Hexadecane |
0.79 ± 0.0 |
Total aliphatic hydrocarbons |
7.82 |
12 |
Alcohol |
8.2609 |
1106 |
β-Linalool |
0.45 ± 0.0 |
Total alcohols |
0.45 |
13 |
Aldehyde |
8.304 |
1110 |
Nonanal |
0.34 ± 0.0 |
Total aldehyde |
0.34 |
14 |
Aromatic |
8.5455 |
1132 |
o-Cymene |
0.03 ± 0.0 |
15 |
12.0261 |
1484 |
p-Cymene |
1.10 ± 0.0 |
Total aromatics |
1.13 |
16 |
Ether |
10.8708 |
1368 |
Isoeugenol |
74.49 ± 0.0 |
Total ethers |
74.49 |
Ethers were detected as the major class in A. digitata fruit (74.5%), represented mostly by isoeugenol (peak 18). Isoeugenol is a common flavoring agent used as a food preservative owing to its antimicrobial activity.58 The relative amounts of both organic acids and esters in A. digitata were at ca. 9.1 and 7.1% of total detected aroma compounds, respectively. Hexadecanoic acid methyl ester (peak 3, 8.1%) was the most abundant ester in A. digitata fruit to mediate for its antimicrobial properties.59
In contrast, alcohols were detected at trace levels of 0.5% represented by linalool (peak 14) a monoterpene alcohol used as flavoring and antibacterial agent.60 Aliphatic hydrocarbons accounted for 7.8% of the volatile composition in baobab fruit. Major forms included 1,3-butadiene, 2,3-dimethyl (peak 6), and 2,4-hexadiene (peak 7) at 3.2 and 1.8%, respectively. Aromatic compounds were also detected at a low level at 1.13% of the total volatiles represented by o-cymene (peak 16) and p-cymene (peak 17).
3.1.5. NMR-based metabolites fingerprinting of A. digitata fruits. NMR fingerprinting was employed for the analysis of A. digitata fruits. Peaks assignment was established on the previously reported chemical shifts in literature. Further, 2D-NMR experiments were employed including 1H–1H-COSY, 1H–13C HSQC (heteronuclear single quantum coherence spectroscopy), and HMBC (heteronuclear multiple bond correlation). 15 Metabolites were identified and listed with their respective chemical shifts in ESI Table 1.† The illustrative 1H-NMR spectrum of A. digitata fruit extract is shown in ESI Fig. 2.†Typically, 1H-NMR spectrum of A. digitata fruits compromised two main regions, a low field region (δ 0.5–5.5 ppm) with more abundant signals of the primary metabolites (ESI Fig. 2a†), and a high field region of much lower abundance corresponding to secondary metabolites to account more for fruits health effects (ESI Fig. 2b†). Primary metabolites identified included fatty acids (N1 & N2), amino acids (N3–N8), organic acids (N9 & N10), and sugars (N11–N13). While secondary metabolites belonged to flavonoids (N14–N15), ESI Table S1.†
The first recognized metabolites in spectrum belonged to ω-6 fatty acids (N1) as evident from the broad signal at δ 0.87–0.91 ppm for the terminal methyl, δ 2.77 ppm for the allylic methylene and δ 5.36 for the olefinic protons.13 A fatty acid ester (N2) was recognized from the upfield shifts of H-2 and H-3 at δ 2.26 and 1.53 ppm, respectively. Several amino acids were detected including (N5) leucine (δ 0.96 ppm, d, 5.4), (N6) alanine (δ 1.41 ppm, d, 7.2), and (N8) proline (δ 4.17 ppm, dd, 2.4, 7.2). Further, downfield region revealed the presence of aromatic amino acid i.e.,(N7) phenylalanine via its characteristic signals at δ 7.55 ppm (d, 1.8), δ 7.57 ppm (d, 2.4), and δ 7.58 ppm (d, 2.4).
The presence of other nitrogenous compounds i.e., γ-aminobutyric acid (N3) was also evident from its signals at δ 1.973 ppm (m), 2.209 ppm (t, 7.2), and δ 2.72 ppm along with choline (N4) through the signal at δ 3.202 ppm (s). Besides the presence of organic acids were evident from signals ascribed to (N9) citric acid detected at δ 2.540 ppm (d, 15.6) and δ 2.567 ppm (d, 15.6), and (N10) malic acid with its signal at δ 4.091 ppm (dd, 12, 6). Citric and malic acids were detected also in GC-MS which indicate the efficiency of identification process, Table 2.
Sugars abundance observed from GC/MS Table 2, was also confirmed using 1H-NMR spectrum, and in agreement with previous reports.25 Sugars were assigned based on their characteristic anomeric signals at δ 4.401 ppm (d, 7.8) for β-glucose (N11), δ 5.033 ppm (d, 3.6) for α-glucose (N12), and δ 5.319 ppm (d, 3.6) for sucrose (N13).
Comparably, the aromatic region revealed much less abundant signals, mostly for flavonoids. As previously described, catechin and its conjugates (N11), were the major flavonoids assigned from signals at δ 5.841 ppm (d, 2.4), δ 5.86 ppm (d, 2.4) for H-6 & H-8, and characteristic multiplet at δ 3.965 ppm for H-3. Quercetin, a common flavonol in planta was also detected based on signals at δ 6.924 ppm (d, 2.4, H-6), δ 6.936 ppm (d, 2.4, H-8), and δ 7.148 ppm (d, 2.4). The enrichment of flavonoids is likely to account for fruits reported antioxidant and antidiabetic effects.61
3.1.6. Quantification of major metabolites detected via 1H-NMR. 1H-NMR was employed for the quantification of major primary and secondary metabolites in the A. digitata fruits for future standardization purposes (ESI Table S2†). Recently, NMR was successfully used for the quantification of metabolites with no standards required as in case of LC/MS.62,63 Total sugars in A. digitata fruits were detected and quantified at 58.9 μg mg−1 with sucrose as the predominant sugar at 40.04 μg mg−1, contributing to fruit sweetness. Another class to account for the taste were organic acids with a content of 5.5 μg mg−1, mainly as malic acid (3.4 μg mg−1 dry powder) and citric acid (2.1 μg mg−1 dry powder). A. digitata fruits showed high choline level at 4.1 μg mg−1 dry powder adding to its nutritional value, being recognized as an essential nutrient for the body function.64 In addition, flavonoids were detected and quantified in A. digitata fruits at 3.97 μg mg−1 dry powder and quercetin was detected as the major flavonoid. The abundance of flavonoids in the fruit add to its biological value as a potent antioxidant and antidiabetic phytochemicals.
3.2. Antidiabetic activity
A. digitata fruits' antidiabetic and its effect on tissues histopathology and different biochemical parameters related to diabetes were investigated in vivo.
3.2.1. Histopathological and immunohistochemistry analysis. Diabetes mellitus is often associated with pancreatic tissue damage, necrosis and microvascular vacuolization of the liver and nephropathy.65 Histopathological examination of the pancreas, kidney, and liver tissues showed no significant difference between diabetic rats treated with a low or high dose of A. digitata (Fig. 3a and b). The elevated expression of insulin in β-cells depicted in (Fig. 3c) was observed in both diabetic groups treated with 150 mg kg−1 and 300 mg kg−1 compared to the control group and metformin-treated group. These results are in accordance with the hypoglycemic effect of A. digitata through the enhancement of insulin production by β cells, as previously reported.66
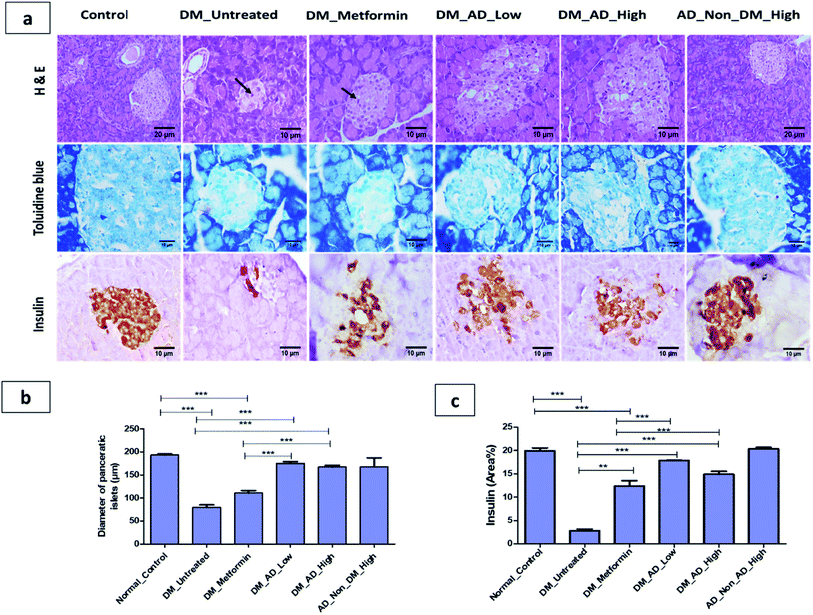 |
| Fig. 3 (a)Photomicrographs of pancerase stained by hematoxylin and eosin, toluidine blue and insulin immunohistochemical staining. (b) The diameter of pancreatic islets (μm). (c) Immunohistochemical analysis for area% of insulin expression. Data expressed as mean ± SD (**P < 0.01, ***P < 0.001). Notable arrows on the figure indicate necrobiotic changes in β-cells. | |
Similarly, vacuolar degeneration, necrobiotic changes, and interstitial nephritis were observed in diabetic untreated and metformin-treated groups. Treatment of diabetic rats with either low or high doses of A. digitata upgraded the histological structure of the kidney to normal (Fig. 4a), while the scoring description of kidney damage is depicted in (Fig. 4b).
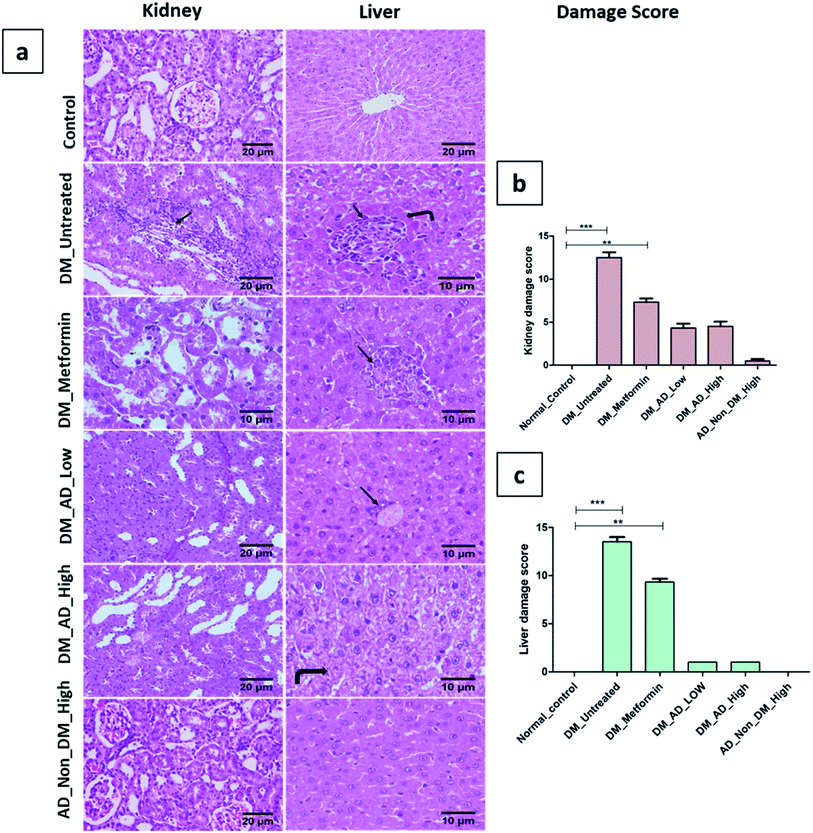 |
| Fig. 4 (a) Photomicrographs of kidney and liver sections stained by (H&E). (b and c): histological damage score in kidney and liver tissues respectively. Data presented as median ± SD. **P < 0.01, ***P < 0.001. Remarkable features are indicated on the figure as follows: inflammatory cell infiltration (arrow), coagulative necrosis (curved arrow). | |
In the same context, administration of A. digitata at both low and high doses improved the histological structure of the liver tissue of diabetic rats. Rats receiving 150 mg kg−1 of A. digitata showed restoration of cellular structure with minimal infiltration of inflammatory cells (Fig. 4a). The normal structure was observed in the group treated with only a high dose of A. digitata extract (Fig. 4a).
3.2.2. Biochemical assays. Administration of both metformin and A. digitata fruit at a high dose (300 mg kg−1) sustained the blood glucose levels when compared to diabetic rats (P < 0.01). Unexpectedly, the lower dose treatment of the A. digitata fruit (150 mg kg−1) recorded better results compared to the higher dose (Fig. 5a). Additionally, the ALT, AST, and lipid profile markers in significantly increased in the untreated diabetic group as illustrated in Fig. 5a. Contrariwise, the diabetic group treated with A. digitata L. at both doses showed a diminution in the ALT, AST (P < 0.05), triglycerides, and HDL (P < 0.05) compared to other groups. Moreover, cholesterol level was considerably reduced with A. digitata L. fruit extract at a dose of 300 mg kg−1, and a more profound reduction was observed at the dose of 150 mg kg−1 (P < 0.01). Likewise, regarding the renal markers, A. digitata L. treated groups with both 150 mg kg−1 and 300 mg kg−1 showed a significant reduction in the creatinine level compared to the diabetic group (P < 0.05).
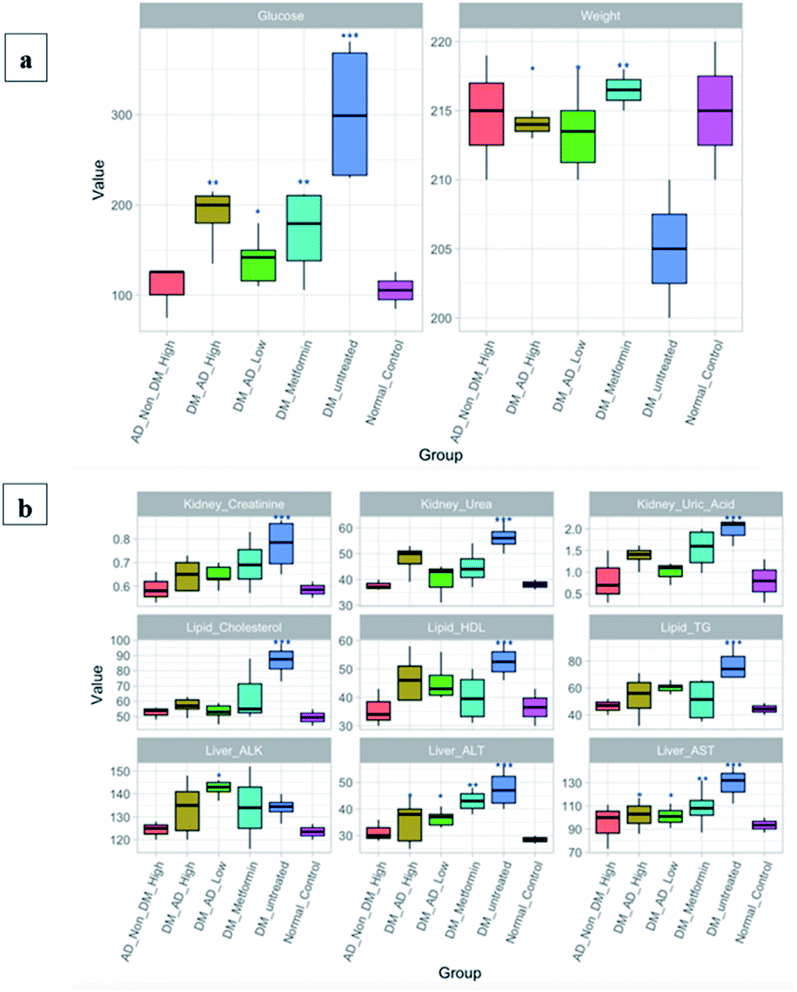 |
| Fig. 5 (a) Fasting blood glucose (FBG) and body weight of rats. (b) Biochemical analyses of the lipid profile, liver, and kidney functions. The groups are normal healthy rats (Normal_Control), diabetic rats without any treatment (DM_Untreated), diabetic rats administrated metformin orally (DM_Metformin), diabetic rats i.p. injection with a low dose, which is 150 mg kg−1, of A. digitata L. (DM_AD_Low), diabetic rats i.p. injection with high dose, which is 300 mg kg−1, of A. digitata L. (DM_AD_High), and healthy rats i.p. injection with high dose of A. digitata L. (AD_Non_DM_High). Figure generated with R software version 3.5.2. Data expressed as mean ± SD (**P < 0.01, ***P < 0.001). The Y-axis is representing the values of each measured parameter; glucose, creatinine, urea, uric acid, cholesterol, HDL, and TG are measured in mg dL−1, whereas ALK, ALT, and AST are measured in IU L−1. Body weight is measured in grams. | |
4. Conclusion
In the present study, holistic metabolites profiling of A. digitata fruits was achieved via different metabolomics platforms. UPLC-MS/MS permitted for the dereplication of 77 compounds belonging to different classes, including organic acids, sugars, alcohols, phenolics, coumarins, and fatty acids. Almost 50% of the identified metabolites (40 compounds) are reported for the first time in A. digitata fruits. GC/MS investigation for both non-volatile and volatile metabolites revealed the identification of 74 non-volatile and 16 volatile compounds. Lastly, identification and quantification of the major metabolites was possible through the employment of 1D-NMR and 2D-NMR spectroscopy. While the investigated fruit is from one origin, same metabolomics platform can be used to assess fruits from other origins for comparative analysis. Simultaneously, the antidiabetic potential of A. digitata fruits extract was also investigated revealing potent activity owing to the abundance of bioactive phytochemicals in the fruits. Histopathological and immunohistochemical studies demonstrated the hepatoprotective and renoprotective effects of A. digitata fruits in STZ induced diabetic rats. Biochemical assays revealed its capacity to lower the fasting glucose, lipid profile, hepatic and renal markers. Further investigations are required for deeper pharmacological, physiological, and biochemical insights and the determination of the optimum therapeutic dose.
Funding
The work was funded by AUC financial support.
Author contributions
Mostafa H. Baky: data curation; writing – review. Marwa T. Badawy: data curation, writing – review. Alaa F. Bakr: writing – review. Nesrine M. Hegazi: data curation; writing – review. Ahmed Abdellatif: writing – review. Mohamed A. Farag: supervision, writing – review & editing.
Conflicts of interest
There is no conflict of interest.
Acknowledgements
Dr Mohamed Farag would like to thank the Alexander Von Humboldt foundation, Germany for funding. The authors would like to acknowledge the contribution of Ms. Eman A. Khalil in facilitating some procedures in histopathology investigation.
References
- G. Kamatou, I. Vermaak and A. Viljoen, S. Afr. J. Bot., 2011, 77, 908–919 CrossRef.
- A. Lamien-Meda, C. E. Lamien, M. M. Compaoré, R. N. Meda, M. Kiendrebeogo, B. Zeba, J. F. Millogo and O. G. Nacoulma, Molecules, 2008, 13, 581–594 CrossRef CAS PubMed.
- F. Chadare, A. Linnemann, J. Hounhouigan, M. Nout and M. Van Boekel, Crit. Rev. Food Sci. Nutr., 2008, 49, 254–274 CrossRef PubMed.
- J. Scheuring, M. Sidibé and M. Frigg, Sight and Life Newsletter, 1999, 1, 21–24 Search PubMed.
- C. Buchmann, S. Prehsler, A. Hartl and C. R. Vogl, Ecol. Food Nutr., 2010, 49, 145–172 CrossRef PubMed.
- Y. Shukla, S. Dubey, S. Jain and S. Kumar, J. Med. Aromat. Plant Sci., 2001, 23, 429–434 CAS.
- M. Y. Gwarzo and H. u. Y. Bako, Int. J. Anim. Vet. Adv., 2013, 5, 108–113 CrossRef.
- M. A. Farag, A. R. Khattab, A. A. Maamoun, M. Kropf and A. G. Heiss, Food Res. Int., 2019, 115, 379–392 CrossRef CAS PubMed.
- S. M. Afifi, A. El-Mahis, A. G. Heiss and M. A. Farag, ACS Omega, 2021, 6, 5775–5785 CrossRef CAS PubMed.
- M. A. Farag, S. M. Afifi, D. M. Rasheed and A. R. Khattab, J. Food Compos. Anal., 2021, 104073 CrossRef CAS.
- M. A. Farag, D. Fathi, S. Shamma, M. S. A. Shawkat, S. M. Shalabi, H. R. El Seedi and S. M. Afifi, LWT, 2021, 142, 111046 CrossRef CAS.
- M. H. Baky, M. A. Farag and D. M. Rasheed, ACS omega, 2020, 5, 31370–31380 CrossRef CAS PubMed.
- M. A. Farag, A. Otify, A. Porzel, C. G. Michel, A. Elsayed and L. A. Wessjohann, Anal. Bioanal. Chem., 2016, 408, 3125–3143 CrossRef CAS PubMed.
- M. Farag, E. Mahrous, T. Lübken, A. Porzel and L. Wessjohann, Metabolomics, 2013, 10, 21–32 CrossRef.
- M. A. Farag, A. Otify, A. Porzel, C. G. Michel, A. Elsayed and L. A. Wessjohann, Anal. Bioanal. Chem., 2016, 408, 3125–3143 CrossRef CAS PubMed.
- L. Olmo-García, K. Wendt, N. Kessler, A. Bajoub, A. Fernández-Gutiérrez, C. Baessmann and A. Carrasco-Pancorbo, Eur. J. Lipid Sci. Technol., 2019, 121, 1800336 CrossRef.
- M. E. Smoot, K. Ono, J. Ruscheinski, P. L. Wang and T. Ideker, Bioinformatics, 2011, 27, 431–432 CrossRef CAS PubMed.
- M. A. Farag, A. R. Khattab, S. Shamma and S. M. Afifi, Foods, 2021, 10, 728 CrossRef CAS PubMed.
- N. A. Qinna and A. A. Badwan, Drug Des., Dev. Ther., 2015, 9, 2515–2525 CrossRef CAS PubMed.
- D. Ahmed, V. Kumar, A. Verma, G. S. Shukla and M. Sharma, Springerplus, 2015, 4, 1–17 CrossRef CAS PubMed.
- R. Klopfleisch, BMC Vet. Res., 2013, 9(1), 1–15 CrossRef PubMed.
- G. B. Ha, E. J. Park, J. E. Shin and H. Y. Shon, PloS one, 2014, 9(7), 1–12 CrossRef PubMed.
- L. Makila, O. Laaksonen, A.-L. Alanne, M. Kortesniemi, H. Kallio and B. Yang, J. Agric. Food Chem., 2016, 64, 4584–4598 CrossRef CAS PubMed.
- P. Lorenz, J. Conrad, J. Bertrams, M. Berger, S. Duckstein, U. Meyer and F. C. Stintzing, Phytochem. Anal., 2012, 23, 60–71 CrossRef CAS PubMed.
- A. J. T. Sokeng, A. P. Sobolev, A. Di Lorenzo, J. Xiao, L. Mannina, D. Capitani and M. Daglia, Food Chem., 2019, 272, 93–108 CrossRef PubMed.
- H. Sun, J. Liu, A. Zhang, Y. Zhang, X. Meng, Y. Han, Y. Zhang and X. Wang, J. Sep. Sci., 2016, 39, 496–502 CrossRef CAS PubMed.
- E. Fujimatu, T. Ishikawa and J. Kitajima, Phytochemistry, 2003, 63, 609–616 CrossRef CAS PubMed.
- H. Tohma, İ. Gülçin, E. Bursal, A. C. Gören, S. H. Alwasel and E. Köksal, J. Food Meas. Charact., 2017, 11, 556–566 CrossRef.
- M. Lutter, A. C. Clark, P. D. Prenzler and G. R. Scollary, Food Chem., 2007, 105, 968–975 CrossRef CAS.
- Y. Zhang, L.-J. Yang, K. Jiang, C.-H. Tan, J.-J. Tan, P.-M. Yang and D.-Y. Zhu, Carbohydr. Res., 2012, 361, 114–119 CrossRef CAS PubMed.
- N. F. Abdullah and R. Mohamad Hussain, J. Liq. Chromatogr. Relat. Technol., 2015, 38, 289–293 CrossRef CAS.
- O. Razanamaro, E. Rasoamanana, B. Rakouth, J. R. Randriamalala, E. Rabakonadrianina, A. Clément-Vidal, J.-M. L. P. Tsy, C. Menut and P. Danthu, Biochem. Syst. Ecol., 2015, 60, 238–248 CrossRef CAS.
- J. A. Seukep, A. G. Fankam, D. E. Djeussi, I. K. Voukeng, S. B. Tankeo, J. A. Noumdem, A. H. Kuete and V. Kuete, Springerplus, 2013, 2, 363 CrossRef PubMed.
- B. B. Ismail, Y. Pu, M. Guo, X. Ma and D. Liu, Food Chem., 2019, 277, 279–288 CrossRef CAS PubMed.
- J. Gruz, O. Novák and M. Strnad, Food Chem., 2008, 111, 789–794 CrossRef CAS.
- S. Wu, A. E. Wilson, L. Chang and L. Tian, Molecules, 2019, 24, 3814 CrossRef CAS PubMed.
- M. K. Lee, H. Y. Jeon, K. Y. Lee, S. H. Kim, C. J. Ma, S. H. Sung, H.-S. Lee, M. J. Park and Y. C. Kim, Planta Med., 2007, 73, 782–786 CrossRef CAS PubMed.
- M. N. Clifford and S. Knight, Food Chem., 2004, 87, 457–463 CrossRef CAS.
- L. Yang, N. Nakamura, M. Hattori, Z. Wang, S. A. Bligh and L. Xu, Rapid Commun. Mass Spectrom., 2007, 21, 1833–1840 CrossRef CAS PubMed.
- X.-N. Li, J. Sun, H. Shi, L. L. Yu, C. D. Ridge, E. P. Mazzola, C. Okunji, M. M. Iwu, T. K. Michel and P. Chen, Food Res. Int., 2017, 99, 755–761 CrossRef CAS PubMed.
- I. M. Abu-Reidah, D. Arráez-Román, A. Segura-Carretero and A. Fernández-Gutiérrez, Food Res. Int., 2013, 51, 354–362 CrossRef CAS.
- A. Rauf, M. Imran, T. Abu-Izneid, S. Patel, X. Pan, S. Naz, A. S. Silva, F. Saeed and H. A. R. Suleria, Biomed. Pharmacother., 2019, 116, 108999 CrossRef CAS PubMed.
- F. Ferreres, S. Magalhães, A. Gil-Izquierdo, P. Valentão, A. R. Cabrita, A. J. Fonseca and P. B. Andrade, Food Chem., 2017, 214, 678–685 CrossRef CAS PubMed.
- R. Vinayagam and B. Xu, Nutr. Metab., 2015, 12, 1–20 CrossRef PubMed.
- M. Ganzera, S. Sturm and H. Stuppner, Chromatographia, 1997, 46, 197–203 CrossRef CAS.
- R. Schuster, Chromatographia, 1980, 13, 379–385 CrossRef CAS.
- D. N. Olennikov, I. A. Fedorov, N. I. Kashchenko, N. K. Chirikova and C. Vennos, Molecules, 2019, 24, 2286 CrossRef CAS PubMed.
- N. Taira, R. N. Nugara, M. Inafuku, K. Takara, T. Ogi, T. Ichiba, H. Iwasaki, T. Okabe and H. Oku, J. Funct. Foods, 2017, 29, 19–28 CrossRef CAS.
- L. Xie, Y. Takeuchi, L. M. Cosentino and K.-H. Lee, J. Med. Chem., 1999, 42, 2662–2672 CrossRef CAS PubMed.
- J. Y.-C. Wu, W.-F. Fong, J.-X. Zhang, C.-H. Leung, H.-L. Kwong, M.-S. Yang, D. Li and H.-Y. Cheung, Eur. J. Pharmacol., 2003, 473, 9–17 CrossRef CAS PubMed.
- H. J. Lee, H. J. Lee, E. O. Lee, J. H. Lee, K. S. Lee, K. H. Kim, S.-H. Kim and J. Lü, Am. J. Chin. Med., 2009, 37, 127–142 CrossRef CAS PubMed.
- A. Nishizawa, Y. Yabuta and S. Shigeoka, Plant Physiol., 2008, 147, 1251–1263 CrossRef CAS PubMed.
- M. L. Croze and C. O. Soulage, Biochimie, 2013, 95, 1811–1827 CrossRef CAS PubMed.
- C. Pereira, L. Barros, A. M. Carvalho and I. C. Ferreira, Food Anal. Methods, 2013, 6, 1337–1344 CrossRef.
- L. Wells, in Handbook of Glycomics, Elsevier, 2010, pp. 45–57 Search PubMed.
- A. Saba, O. Benini and A. Cupisti, Clinical Aspects of Natural and Added Phosphorus in Foods, 2017, pp. 133–141 Search PubMed.
- T. Chanadiri, T. Sanikidze, M. Esaishvili, I. Chkhikvishvili and I. Datunashvili, Georgian Med. News, 2005, 61–63 CAS.
- T. Koeduka, E. Fridman, D. R. Gang, D. G. Vassão, B. L. Jackson, C. M. Kish, I. Orlova, S. M. Spassova, N. G. Lewis and J. P. Noel, Proc. Natl. Acad. Sci., 2006, 103, 10128–10133 CrossRef CAS PubMed.
- M. T. Shaaban, M. F. Ghaly and S. M. Fahmi, J. Basic Microbiol., 2021, 61, 557–568 CrossRef CAS PubMed.
- M. Y. Issa, E. Mohsen, I. Y. Younis, E. S. Nofal and M. A. Farag, Ind. Crops Prod., 2020, 144, 112002 CrossRef CAS.
- R. G. C. Barros, U. C. Pereira, J. K. S. Andrade, C. S. de Oliveira, S. V. Vasconcelos and N. Narain, Food Res. Int., 2020, 136, 109614 CrossRef CAS PubMed.
- A. M. Otify, A. M. El-Sayed, C. G. Michel and M. A. Farag, Metabolomics, 2019, 15, 119 CrossRef PubMed.
- M. A. Farag, M. G. Sharaf El-Din, M. A. Selim, A. I. Owis, S. F. Abouzid, A. Porzel, L. A. Wessjohann and A. Otify, Molecules, 2021, 26, 761 CrossRef CAS PubMed.
- T. C. Wallace, J. K. Blusztajn, M. A. Caudill, K. C. Klatt, E. Natker, S. H. Zeisel and K. M. Zelman, Nutr. Today, 2018, 53, 240 CrossRef PubMed.
- Y. Jaiswal, P. Tatke, S. Gabhe and A. Vaidya, J. Tradit. Complement. Med., 2017, 7, 421–427 CrossRef CAS PubMed.
- M. Y. Gwarzo, Int. J. Anim. Vet. Adv., 2013, 5, 108–113 CrossRef.
- A. Braca, C. Sinisgalli, M. De Leo, B. Muscatello, P. L. Cioni, L. Milella, A. Ostuni, S. Giani and R. Sanogo, Molecules, 2018, 23, 3104 CrossRef PubMed.
- I. M. Abu-Reidah, D. Arráez-Román, M. Al-Nuri, I. Warad and A. Segura-Carretero, Food Chem., 2019, 279, 128–143 CrossRef CAS PubMed.
- K. Singh, B. K. Dubey, A. C. Tripathi, A. P. Singh and S. K. Saraf, Nat. Prod. Res., 2014, 28, 1313–1317 CrossRef CAS PubMed.
- P. Vanachayangkul, V. Butterweck and R. F. Frye, J. Chromatogr. B, 2009, 877, 653–656 CrossRef CAS PubMed.
- A. Napolitano, A. Cerulli, C. Pizza and S. Piacente, Food Chem., 2018, 269, 125–135 CrossRef CAS PubMed.
- K. Feussner and I. Feussner, in High-Throughput Metabolomics, Springer, 2019, pp. 167–185 Search PubMed.
- K.-H. Nam, H. J. Kim, I.-S. Pack, H. J. Kim, Y. S. Chung, S. Y. Kim and C.-G. Kim, Appl. Biol. Chem., 2019, 62, 15 CrossRef.
- Y. Liang, G. Y. Yan, J. L. Wu, X. Zong, Z. Liu, H. Zhou, L. Liu and N. Li, Phytochem. Anal., 2018, 29, 398–405 CrossRef CAS PubMed.
- M. A. Osman, Plant Foods Hum. Nutr., 2004, 59, 29–33 CrossRef CAS PubMed.
- G. Razafimamonjison, J. M. Leong Pock Tsy, M. Randriamiarinarivo, P. Ramanoelina, J. Rasoarahona, F. Fawbush and P. Danthu, Chem. Biodiversity, 2017, 14, e1600441 CrossRef PubMed.
Footnotes |
† Electronic supplementary information (ESI) available. See DOI: 10.1039/d1ra08277a |
‡ Equally contributed to the work. |
|
This journal is © The Royal Society of Chemistry 2021 |
Click here to see how this site uses Cookies. View our privacy policy here.