DOI:
10.1039/D1RA06590D
(Paper)
RSC Adv., 2021,
11, 32622-32629
An excellent water-stable 3D Zn-MOF with 8-fold interpenetrated diamondoid topology showing “turn-on/turn-off” luminescent detection of Al3+ and SNT in aqueous media†
Received
1st September 2021
, Accepted 27th September 2021
First published on 11th November 2021
Abstract
The effective detection of Al3+ and antibiotics pollutants is crucial for environmental protection and human health due to their high toxicity and nondegradability, but it is still currently challenging. In this work, a methyl-decorated acylamide-containing dicarboxylate ligand H2L was elaborately designed and successfully synthesized, which was further employed to react with Zn(II) ions and dpe ligands to construct a new luminescent Zn-MOF, namely {[Zn(L)(dpe)]·DMF·3H2O}n (1). X-ray crystallography reveals that MOF 1 is a 3D 8-fold [4 + 4] interpenetrated diamondoid framework with isolated DMF and water molecules in the pores, in which the uncoordinated acylamide groups are found and facilitate the Zn-MOF to recognize analyte molecules. The activated solvent-free MOF sample (denoted as 1a) exhibits excellent water stability and good luminescent performance. The luminescence sensing experiments show that 1a could behave as a bi-responsive chemical sensor for “turn-on” and “turn-off” luminescent detection of Al3+ and SNT in aqueous media, respectively. The sensing mechanisms have also been discussed. Furthermore, MOF 1a was successfully applied to the effective determination of Al3+ and SNT in real water samples. This represents, to our knowledge, the first example of a MOF material for “turn-on” and “turn-off” luminescent detection of Al3+ and SNT in water, which will promote the practical application of water-stable luminescent MOF sensors in monitoring metal ions and antibiotics pollutants in the environmental water matrices.
Introduction
Trivalent aluminum ions (Al3+) and antibiotics pollutants are becoming a serious global problem for harming the ecological environment and human health due to their high toxicity and nondegradability.1–4 Al3+ is one of the common cations that can cause drinking water pollution. The excess Al3+ entering the human body would lead to severe illnesses including Alzheimer's disease and Parkinson's disease.5,6 Similarly, the abuse of antibiotics such as sulfanitran (SNT) has brought about high level of antibiotic residue, although the antibiotics have been widely used for the treatment of bacterial infections in human beings and animals. The large amount of antibiotics residues in various aquatic environment would not only generate the drug resistance for microbial strains among mankind but also increase the risk of human carcinogenic and mutagenic diseases by bioaccumulation effect.7–9 Currently, the inductively coupled plasma mass spectrometry (ICP-MS),10 atomic absorption spectrometry (AAS)11 and liquid chromatography tandem mass spectrometry (LC-MS)12 are the conventional methods to detect Al3+ or antibiotics, but they exhibit some disadvantages such as complicated operation, expensive equipment, and time-consuming procedure. Therefore, it is urgent for scientists to develop a simple, inexpensive and time-saving approach for detection of Al3+ and SNT in water.
Luminescent metal–organic frameworks (MOFs), as a new type of crystalline materials, are obviously superior to other luminescent materials due to their excellent optical performance, permanent porosity, high surface area, and easily tailorable structures and functions.13–15 Taking advantage of these unique merits, some luminescent MOFs have been successfully developed into “turn-on” and “turn-off” bi-responsive chemical sensors for detecting different pollutants including metal ions and/or organic pollutants via luminescence enhancing or luminescence quenching mechanism, respectively. For example, Wang and co-workers reported a luminescent 3D Cd-MOF behaving as a bi-responsive chemical sensor in DMF solutions for “turn-on” detecting Al3+ and Cr3+ as well as “turn-off” detecting Fe3+, respectively.16 Li group reported a highly luminescent 3D Zn-MOF acting as a bi-responsive chemical sensor for “turn-on” detecting toluene vapor and “turn-off” detecting nitrobenzene vapor, respectively.17 Zhang et al. reported a luminescent 2D Zn-MOF serving as a bi-responsive chemical sensor in the aqueous phase for “turn-on” detecting Al3+ and “turn-off” detecting nitroaromatic explosives, respectively.18 However, to our knowledge, no study on exploring MOF as “turn-on” and “turn-off” bi-responsive luminescent sensor for detecting Al3+ and antibiotics has been reported so far.
Water stability of luminescent MOFs in various water environment even acid and base aqueous solutions is key factor for practical applications.19–21 Generally, there are two most common methods to improve the water stability of target MOFs. The first one is the use of high-valent metal ions to form strong coordination bonds with organic ligands.22,23 The other one is the incorporation of hydrophobic groups around coordination sites of organic ligands to enhance the hydrophobic property of MOFs, and then to protect coordination bonds from hydrolysis.24 In addition, a comparative study showed that the interpenetrated topological structure would also obviously enhance the water stability of target MOFs.25,26
In this work, a methyl-decorated acylamide-containing dicarboxylate ligand, 4,4′-((naphthalene-1,4-dicarbonyl)bis(azanediyl))bis(2-methylbenzoic acid) (H2L), has been elaborately designed and successfully synthesized, in which two hydrophobic methyl groups are introduced into the ortho-position of the coordination carboxylate groups in order to enhance the water stability of target MOFs, as well as two acylamide groups are also introduced into H2L ligands as guest-accessible functional organic sites (FOS) in order to improve their sensing ability. As expected, a new Zn-MOF {[Zn(L)(dpe)]·DMF·3H2O}n (1) was obtained by reaction of H2L, Zn(NO3)2·6H2O and 1,2-di(pyridin-4-yl)ethane (dpe) under solvothermal condition. X-ray crystallography revealed that MOF 1 exhibited a 3D 8-fold [4 + 4] interpenetrated diamondoid framework with isolated DMF and water molecules in the pores. The activated solvent-free MOF sample 1a was fabricated by the solvent-exchange and vacuum heat treatment technique, showing an enhanced stability in water, boiling water, as well as HCl and NaOH aqueous solution. The luminescence sensing experiments showed that 1a could behave as a bi-responsive chemical sensor for “turn-on” and “turn-off” luminescent detection of Al3+ and SNT in aqueous media (Fig. 1). In addition, the “turn-on” and “turn-off” luminescent sensing mechanisms for Al3+ and SNT have also been discussed. To our knowledge, this represents the first example of bi-responsive MOF sensor for “turn-on” and “turn-off” luminescent detection of Al3+ and SNT in aqueous media.
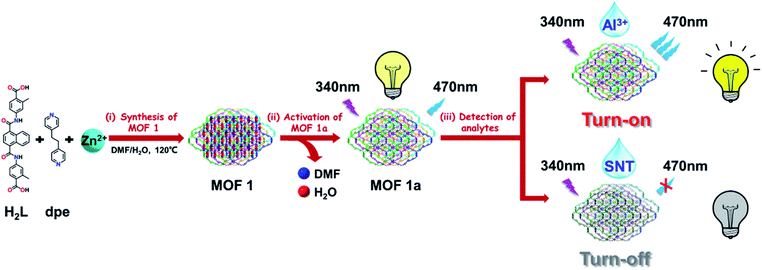 |
| Fig. 1 Schematic diagram of luminescent MOF 1a as a bi-responsive chemical sensor for “turn-on” and “turn-off” detection of Al3+ and SNT respectively, including (i) synthesis of pristine MOF 1, (ii) activation of MOF 1a, and (iii) detection of different analytes. | |
Experimental section
Materials and measurements
All chemicals were of analytical grade and used without further purification. Elemental analyses for C, H, and N were carried out on an Elementar vario EL cube analyzer. Infrared spectra were recorded on a Nicolet-5700 FT-IR spectrophotometer with KBr pellets. Powder X-ray diffraction (PXRD) patterns were recorded on a Rigaku SmartLab 9 kW diffractometer with Cu Kα radiation (λ = 1.5418 Å) at room temperature. Thermogravimetric analyses (TGA) were performed under the nitrogen atmosphere on a Netzsch STA 449F5 thermal instrument. Adsorption isotherms for water vapor were measured with an Hiden intelligent gravimetric sorption analyzer at 298 K. The contact angle toward water was recorded with Krüss DSA 100S instrument. X-ray photoelectron spectroscopy (XPS) were performed on a Thermo Fisher Scientific Escalab Xi+ system. The absorption spectra were recorded on a Persée T9CS UV-Vis spectrophotometer. The photoluminescence spectra were measured on a Hitachi F-4700 spectrophotometer.
Synthesis of {[Zn(L)(dpe)]·DMF·3H2O}n (1)
A mixture of Zn(NO3)2·6H2O (29.8 mg, 0.1 mmol), H2L (48.2 mg, 0.1 mmol) and dpe (18.4 mg, 0.1 mmol) in DMF/H2O (3 mL, v/v = 1
:
2) was sealed in a Teflon-lined stainless steel vessel and heated at 120 °C for 3 days, and then cooled to room temperature at a rate of 5 °C h−1. Colorless block crystals of MOF 1 were obtained with a 60.2% yield based on Zn(II). Elemental anal. calcd for C43H45N5O10Zn (%): C, 60.25; H, 5.29; N, 8.17; anal. found: C, 60.23; H, 5.32; N, 8.21. Selected IR (KBr, cm−1): 3423, 1664, 1615, 1525, 1383, 1311, 1253, 833, 793.
Sample activation of 1a
The as-synthesized sample of 1 was firstly immersed into anhydrous methanol for 3 days, and the methanol was refreshed three times each day. Then the similar procedure was utilized to treat the sample with dichloromethane to remove methanol molecules. After removal of dichloromethane by centrifuging, the sample was dried under vacuum at 80 °C for 12 h to yield activated solvent-free sample 1a. Solid samples of 1a were ground into powder and used for other measurements.
Luminescence measurements
In a typical luminescence experiment, 0.86 mg finely grounded powder of 1a was added to a cuvette containing 10 mL deionized water, and then ultrasonicated for 8 h to form a uniform suspension. All the titration experiments were performed by adding the selected analytes to 1 mL of 1a suspension. The luminescence spectra were recorded with an emission peak around 470 nm upon excitation at 340 nm. All the experiments were performed in triplicate, and the consistent results were reported.
DFT calculation
HOMO–LUMO energies were obtained using Materials Studio 7.0 version. In the DFT calculations, the exchange-correlation functional PW916 within the generalized gradient approximation (GGA) and the double numerical plus polarization (DNP) basis set as implemented in the DMol3 package of MS were used to optimize the geometries and evaluate the HOMO–LUMO energies of the analytes.
X-ray crystallography
Single-crystal X-ray diffraction data were collected on a Bruker SMART-1000 CCD diffractometer with graphite-monochromated Mo Kα (λ = 0.71073 Å) radiation at 298 K. The structure was solved by direct methods and refined by the full-matrix least-squares methods on F2 using the SHELXL-2018 program.27 Crystal and refinement data are summarized in Table S1.† Selected bond lengths and angles are listed in Table S2.†
Results and discussion
Description of crystal structure
Single-crystal X-ray diffraction analysis reveals that MOF 1 crystallizes in the monoclinic space group P21/n and consists of one Zn(II) ion, one L2− ligand, one dpe ligand, three isolated H2O and one isolated DMF molecules in the asymmetric unit (Fig. S1†). In MOF 1, the central Zn(II) ion (Zn1) is four-coordinated by two carboxylic O atoms (O1 and O6A, symmetry codes: A: x − 3/2, −y + 1/2, z − 1/2) from two different L2− ligands and two pyridyl N atoms (N3 and N4B, symmetry codes: B: x − 1/2, −y + 3/2, z + 1/2) from two different dpe ligands, displaying a distorted [ZnO2N2] tetrahedral coordination geometry. All the Zn–O and Zn–N lengths are in the normal range (Table S2†). The deprotonated L2− ligand in 1 acts as a linear μ2-bridging ligand with two monodentate coordination carboxylic O atoms, and the dpe also acts as a linear μ2-bridging ligand with two coordination pyridyl N atoms (Fig. S2†). Interestingly, ten 4-connected tetrahedral Zn(II) centres are linked by six linear μ2-bridging L2− ligands and six linear μ2-bridging dpe ligands to construct an diamondoid cage with a large internal pore diameter of 21.5 Å (Fig. 2a). These adjacent diamondoid cages via sharing 4-connected Zn(II) centres are further extended into form a 3D dia network, which possesses large pores (25.13 × 25.09 Å2) along the crystallographic [10−1] direction (Fig. 2b). Due to the large pores in the single non-interpenetrated 3D network, eight such independent 3D networks interpenetrate with each other to form an 8-fold interpenetration structure in 1 (Fig. 2c). The detailed analyses show that the 8-fold interpenetrated structure in 1 can be best described as the [4 + 4] mode, in which a single network is interpenetrated by three other identical networks to form a 4-fold interpenetration network that then cross each other (Fig. 2d). As reported, the 8-fold interpenetration with [4 + 4] mode is rather rare.28–31 In spite of the high level of interpenetration, MOF 1 still possesses available pores filled with guest DMF and H2O molecules (Fig. S3†). After removal of the guests, the solvent-accessible void volume is up to 23.6% as calculated by PLATON,32 which is close to that observed in 8-fold interpenetrated [Zn(oba)(pip)]n (oba = 4,4′-oxybis(benzenedicarboxylate), pip = 4-pyridyl functionalized benzene-1,3-dicarbohydrazide) (27.2%)33 but much larger than that in [Zn(L1)(1,4-BDC)]·H2O (L1 = N,N′-di(4-pyridyl)adipoamide; 1,4-H2BDC = 1,4-benzenedicarboxylic acid) (4.9%).34
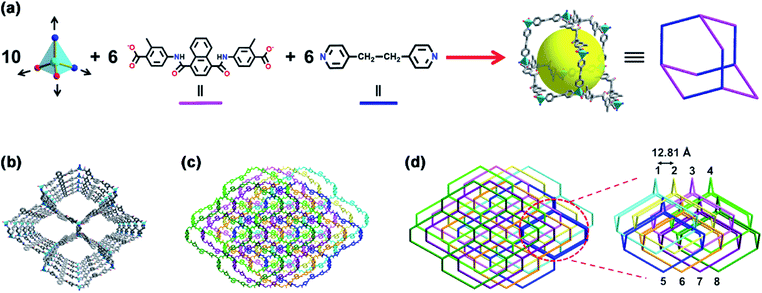 |
| Fig. 2 Illustrating the structure of 1: (a) the assembly of a single diamondoid cage. (b) A single non-interpenetrated 3D framework (c) 8-fold interpenetrated 3D framework. (d) The 8-fold interpenetrated topology with [4 + 4] mode. | |
Stability test
To obtain porous MOFs materials, the solvent-exchange and vacuum heat treatment technique are usually used to removal of isolated solvent molecules. Therefore, the thermal stability of 1 was investigated firstly. As shown in the Fig. S4a,† the thermogravimetric analysis (TGA) of 1 shows an initial weight loss of 14.78% in the range of 120–260 °C, which may be attributed to the loss of one isolated DMF and three isolated H2O molecules (calcd 14.83%). No further weight loss is observed below 305 °C, indicating a good thermal stability. Upon further heating, the framework begins to decompose and the final residue may be attributed to ZnO (obsd 9.45%, calcd 9.50%). The above results are also supported by the variable-temperature PXRD data (Fig. S4b†). Whereas the TGA of activated 1a does not show any weight loss below 305 °C, indicating completely removing the isolated DMF and H2O molecules and successfully obtaining a porous solvent-free MOF material.
Considering the practical application in aqueous media, the water stability of porous 1a was further investigated in water, boiling water, as well as HCl and NaOH aqueous solution. As shown in Fig. S5a,† PXRD patterns of 1a after immersion in water at RT for two weeks and in boiling water for 72 h well match with those of untreated 1a, demonstrating the high water stability. The further PXRD measurements of 1a samples after treated by different HCl and NaOH aqueous solution exhibit that the diffraction peaks don't show apparently change with pH of 3–10 for 12 h, indicating the good water stability under these conditions; whereas the diffraction peaks start to disappear when pH < 2 or pH > 11, indicating the decomposition of 1a occurs (Fig. 3a). Moreover, the water adsorption isotherms of 1a in all five cycles almost overlap with that of origin one, further confirming the excellent water stability of 1a (Fig. 3b). The introduction of methyl groups in H2L ligand and high degree of interpenetrated structure may be the main factors in enhancing the water stability of 1a, although it still exhibits a slight hydrophilic nature with water contact angle of 86° (Fig. S5b†).
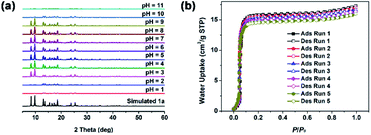 |
| Fig. 3 (a) PXRD patterns of 1a samples after treated by different HCl and NaOH aqueous solution with pH 1–11 for 12 h. (b) Water adsorption isotherm cycles of 1a at 298 K. | |
Luminescence properties
MOFs constructed from d10 metal ions and electron-rich π-conjugated organic ligands are usually considered as the excellent luminescent materials. Thus, the luminescence property of 1a dispersed in water was investigated at room temperature. As shown in Fig. S6,† MOF 1a exhibits a strong emission peak appearing at 470 nm upon excitation at 340 nm. Compared with the free ligand H2L, the emission peak of MOF 1a displays a large red-shift about 66 nm, which may be assigned to the ligand-to-metal charge transfer (LMCT).35–38
Detection of metal ions
Considering the high water stability and good luminescent performance, MOF 1a was applied to detect different metal ions in aqueous solution. In this work, a series of metal ions including Li+, K+, Na+, Ni2+, Co2+, Zn2+, Mg2+, Cu2+ and Al3+, were tested. As shown in Fig. 4a, the luminescence intensity of 1a is obviously increased upon the addition of Al3+, which is nearly 3 times of the initial luminescence intensity when the concentration of Al3+ is up to 167.0 μM. The other metal ions display ignored or slightly reduced the luminescence intensity of 1a under the similar conditions.
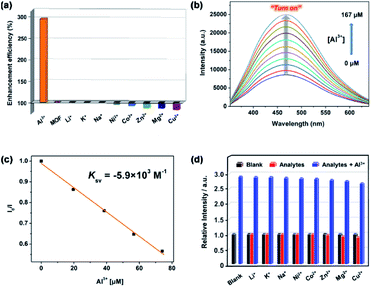 |
| Fig. 4 (a) Enhancement efficiency of 1a toward different metal ions. (b) Emission intensities of 1a upon incremental addition of Al3+. (c) SV plot of Al3+ at lower concentrations. (d) Selective detection of Al3+ in the presence of other interfering metal ions. | |
To further study sensing ability of 1a toward Al3+, the luminescence titrations experiments were performed. The pH is an important parameter in detection of Al3+, because the speciation of Al3+ will be greatly affected by pH values. As shown in Fig. S7,† the Al3+ will be gradually hydrolysed to form [Al(H2O)6]3+, [Al(H2O)5(OH)]2+, [Al(H2O)4(OH)2]+, Al(OH)3, [Al(OH)4]− and so on, with the increase of pH value. Together with consideration of the water stability of 1a, pH = 3 is the optimal detection for the present Al3+ detection. As shown in Fig. 4b, the luminescence intensity of 1a at 470 nm progressively enhances with increasing the concentration of Al3+. The enhanced luminescence efficiency of Al3+ is analysed by using the Stern–Volmer equation18: I0/I = Ksv[Q] + 1, where [Q] is the molar concentration of the analyte and Ksv is the enhancement constant (M−1), I0 and I are the luminescence intensities before and after addition of the analyte, respectively. As shown in Fig. 4c, the SV plot of Al3+ is nearly linear at low concentration and the enhancement constant is about 5.9 × 103 M−1, which is very close to the reported value of Zn-MOF for detection of Al3+ (6.04 × 103 M−1).39 Based on the slope of the calibration curve (K) and the standard deviation (δ) for three repeated luminescence measurements of blank solutions, the limit of detection (LOD, 3δ/K) of 1a toward Al3+ is calculated to be 41.9 ppb, which is better than the reported luminescent MOF sensors for Al3+ detection (Table S3†). It is worth noting that the present LOD toward Al3+ (41.9 ppb) is much lower than the recommended level of Al3+ in drinking water from the United States Environmental Protection Agency (200 ppb). The selective detection of Al3+ in the presence of other interfering metal ions was also examined. As shown in Fig. 4d, the emission intensity of 1a almost does not change in the presence of other interfering metal ions but is significantly enhanced upon the introduction of Al3+ into the mixture of 1a and other interfering metal ions, which indicates that the interference from other metal ions could be neglected and confirms the highly selective detecting ability of 1a toward Al3+. In addition, MOF 1a could be regenerated and reused by centrifuging the dispersed solution after sensing of Al3+ and washing with water several times, which could almost regain the initial luminescence intensity over five cycles (Fig. S8†). Thus, MOF 1a could be used as a highly selective and reversible “turn-on” luminescent sensor for the detection of Al3+ in practical applications.
To better understand the luminescence enhancement effect of 1a toward Al3+, an explanation of the possible mechanism was attempted. As known, the luminescent sensing effect of MOFs caused by metal ions usually derives from the damage of the MOF structures,40 the cation changes between metal centres of MOFs and the target cations,41 or the host–guest interactions between MOFs and analytes.42,43 As shown in Fig. 5a, the PXRD patterns with addition of Al3+ are similar to that of experimental 1a, suggesting that the framework maintains its integrity. Thus, the turn-on sensing mechanism of 1a toward Al3+ could not be due to the collapse of the MOF structure. Furthermore, the luminescence enhanced mechanism of 1a toward Al3+ should also not result from cation-exchange due to the neutral framework of 1a. The XPS data exhibit that there exist Al3+ after the luminescence titration experiments of 1a (Fig. S9†). Therefore, the sensing mechanism may be attributed to the host–guest interactions between Al3+ and the framework of 1a. In IR spectra, the characteristic C
O vibration band of acylamide group is shifted from 1664 (for 1a) to 1630 cm−1 (for 1a + Al3+) and a new absorption peak is observed at 590 cm−1 in 1a + Al3+, both indicate the presence of Al → O contact between Al3+ and the acylamide C
O units (Fig. S10†). As shown in Fig. 5b, the UV-vis spectra show that the absorbance of 1a in range of 320–400 nm almost doesn't change with addition of different metal cations except Al3+, which indicates that the sensing mechanism may be the absorbance caused enhancement (ACE) effect.44 To eliminate the influence of Al3+ itself, the Al3+ absorption spectrum was also measured in aqueous solution (Fig. S11†). The result shows that the Al3+ have no absorbance in the range of 320–400 nm, so we rationally believe that the significant absorbance enhancement of 1a should derive from the complexation of 1a and Al3+. In addition, the UV-vis titration experiments of 1a toward Al3+ were performed to further elucidate the ACE mechanism. As show in Fig. 5c, the absorbance of 1a gradually enhances with the increasing of Al3+ concentrations, which is well consistent with the above luminescence titration results. However, both absorption and emission wavelengths of 1a basically do not change in UV-vis (Fig. 5c) and luminescence (Fig. 4b) titration experiments. Therefore, we speculated the luminescence enhancement mechanism of 1a toward Al3+ should be that 1a + Al3+ absorbs more energy than pristine 1a from the light source in the excitation process, and then it will release more energy than 1a in the emission process, thus yielding the luminescent “turn-on” effect (Fig. 5d). The similar phenomena were also observed in other luminescent MOF sensors for detection of Al3+.43,44
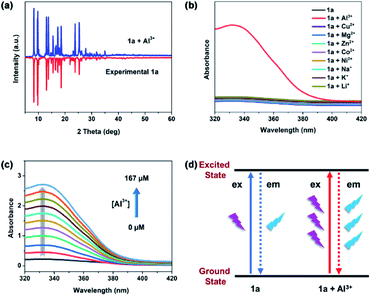 |
| Fig. 5 (a) PXRD patterns of 1a and 1a + Al3+. (b) UV-vis absorption spectra of 1a upon addition of various metal ions. (c) UV-vis absorption spectra of 1a upon incremental addition of Al3+. (d) The schematic illustration of ACE effect for 1a + Al3+. | |
Detection of antibiotics
The similar procedure was used to investigate the sensing ability of 1a for detecting different antibiotics including sulfanitran (SNT), sulfadiazine (SDZ), sulfamethazine (SMZ), sulfamethoxazole (SMX), roxithromycin (ROX), azithromycin (AZM) and gentamicin (GEN) in aqueous media. As shown in Fig. 6a, MOF 1a presents a high quenching efficiency about 96.8% toward SNT, while the quenching efficiencies toward other antibiotics are very low.
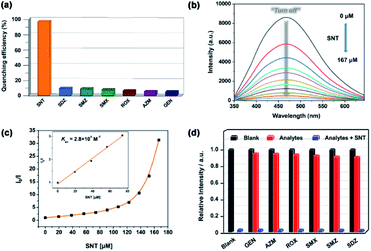 |
| Fig. 6 (a) Quenching efficiency of 1a toward different antibiotics. (b) Emission intensities of 1a upon incremental addition of SNT. (c) SV plot of SNT. Inset: SV plot at lower concentrations. (d) Selective detection of SNT in the presence of other interfering antibiotics. | |
To further study sensing ability of 1a toward SNT, the luminescence titrations experiments were performed. As shown in Fig. 6b, the luminescence intensity of 1a progressively decreases with the gradual addition of SNT. The S–V equation can also be employed to analysed the quenching efficiency of SNT according to the literature.18 As shown in Fig. 6c, the SV plot for SNT was nearly linear below 74.1 μM but subsequently deviated from linearity and bent upward at higher concentrations. The nonlinear nature of SV plot of SNT may be ascribed to an energy-transfer process or self-absorption.45–48 The quenching constant is calculated to be 2.8 × 104 M−1 for SNT, which is close to the reported value of luminescent MOF sensors toward antibiotics analytes.49–52 The LOD could reach 20.2 ppb based on 3δ/K, showing the highly sensitive sensing ability to SNT for 1a. The selective detection of SNT in the presence of other interfering antibiotics was also examined. As shown in Fig. 6d, the emission intensity of 1a almost does not change in the presence of other interfering antibiotics but is significantly quenched upon the introduction of SNT into the mixture of 1a and other interfering antibiotics, which indicates that the interference from other antibiotics could be neglected and confirms the highly selective detecting ability of 1a toward SNT. In addition, MOF 1a could be also regenerated and reused by centrifuging the dispersed solution after sensing of SNT and washing with water several times, which could almost regain the initial luminescence intensity over five cycles (Fig. S12†). Thus, the MOF 1a could be used as a highly selective and reversible “turn-off” luminescent sensor for the detection of SNT in practical applications.
In order to better understand the luminescence quenching effect of 1a toward SNT, the quenching mechanism was investigated. Taking account of the strong π-conjugated effect of H2L ligand and the nature of electron deficiency of analytes, the photoinduced electron transfer (PET) mechanism is proposed. Generally, the excited electrons lying in the higher LUMO of the luminescent ligand are transferred to the lower LUMO of the electron-deficient analytes, resulting in luminescence quenching. The lower the LUMO energy of analytes, the more easily the electrons are transferred to the acceptor.49 As revealed by Fig. 7a and Table S4,† the calculated LUMO energies are in good agreement with the maximum quenching efficiency observed in SNT, but the order of the experimental quenching efficiencies is not fully in accordance with the LUMO energies of the other antibiotics. Therefore, the PET might be not the only mechanism for luminescence quenching in the current system. To further ascertain the possible luminescence quenching reason, the excitation spectrum of 1a and the UV-vis absorption spectra of various antibiotics were investigated. As shown in Fig. 7b, the absorption spectrum of SNT has the greatest degree of overlapping with the excitation spectrum of 1a, but no effective spectral overlaps are observed between 1a and other antibiotics. Thus, the excitation energy from the light source could be partially absorbed by SNT, which causes the luminescence weakening or quenching. The above facts suggest that the inner filter effect (IFE) may be another reason for the luminescence quenching of SNT in this system. Obviously, the coexistence of PET and IFE between 1a and SNT leads to the “turn-off” luminescent effect, and yields highly sensitive and selective detection of SNT in aqueous solution.
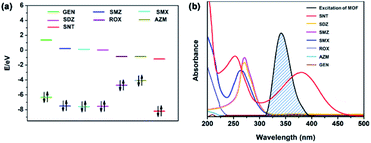 |
| Fig. 7 (a) HOMO and LUMO energies of antibiotics arranged in the descending order of LUMO energy. (b) Spectral overlap of the excitation spectrum of 1a with the absorption spectra of different antibiotics. | |
Application to real water samples
The excellent water stability and good luminescent sensing feature inspired us to explore 1a as an effective chemical sensor for determining the concentrations of Al3+ and SNT in real water samples, such as tap water, the Dongchang Lake and the Yellow River. The water samples spiked with different concentrations of Al3+ and SNT were analysed with the proposed method in this work. As shown in Table S5,† the recoveries are 93.7 to 107.6% with relative standard deviation (RSD) variations from 1.1 to 4.8%, which display satisfactory recoveries and acceptable accuracies. The above results exhibit the feasibility and reliability of the proposed luminescent analysis method for the accurate determination of Al3+ and SNT in environmental water matrices.
Conclusions
In summary, a new luminescent Zn-MOF was successfully synthesized based on an elaborately designed methyl-decorated acylamide-containing dicarboxylate ligand H2L. The as-synthesized MOF 1 shows a three-dimensional 8-fold [4 + 4] interpenetrated diamondoid framework with isolated DMF and water molecules in the pores, in which the uncoordinated acylamide groups are found and facilitated the Zn-MOF to recognize analyte molecules. The activated MOF 1a featured an enhanced water stability due to the introduction of hydrophobic methyl groups in H2L ligand and possession of the high degree of interpenetration structure. The luminescence sensing experiments showed that 1a could behave as a bi-responsive chemical sensor for “turn-on” and “turn-off” luminescent detection of Al3+ and SNT in aqueous media, respectively. The sensing mechanisms have also been discussed. Furthermore, the practical application feasibility of the present luminescent analysis method for the sensing of Al3+ and SNT in real water samples was demonstrated. To our knowledge, this represents the first example of bi-responsive MOF sensor for “turn-on” and “turn-off” luminescent detection of Al3+ and SNT in aqueous media. The present study will provide a new insight in design and syntheses of excellent water-stable luminescent MOFs as bi-responsive luminescent sensors for monitoring metal ions and antibiotics pollutants in the environmental water matrices.
Author contributions
X. T. Gao and X. H. Wang contributed equally to this paper.
Conflicts of interest
There are no conflicts to declare.
Acknowledgements
This work was supported by the National Natural Science Foundation of China (Grant No. 21771096).
References
- W. Adams, R. Blust, R. Dwyer, D. Mount, E. Nordheim, P. H. Rodriguez and D. Spry, Environ. Toxicol. Chem., 2020, 39, 48–59 CrossRef CAS PubMed.
- M. Qiao, G. G. Ying, A. C. Singer and Y. G. Zhu, Environ. Int., 2018, 110, 160–172 CrossRef CAS PubMed.
- Z. Li, M. Li, Z. Zhang, P. Li, Y. Zang and X. Liu, Ecotoxicol. Environ. Saf., 2020, 199, 110668 CrossRef CAS PubMed.
- K. M. Sta Ana, J. Madriaga and M. P. Espino, Environ. Pollut., 2021, 275, 116624 CrossRef CAS PubMed.
- E. Žerovnik, Curr. Alzheimer Res., 2010, 7, 74–83 CrossRef PubMed.
- S. C. Bondy, Neurotoxicology, 2010, 31, 575–581 CrossRef CAS PubMed.
- K. M. A. Akhali, A. K. Alzomar, N. A. Khan and S. S. Alavudeen, Pharm. Globale, 2013, 1, 1–4 Search PubMed.
- Y. Wang, Advances in Social Science, Education and Humanities Research, 2018, vol. 283, pp. 958–960 Search PubMed.
- M. Bacanlı and N. Başaran, Food Chem. Toxicol., 2019, 125, 462–466 CrossRef PubMed.
- S. Murko, J. Ščančar and R. Milačič, J. Anal. At. Spectrom., 2011, 26, 86–93 RSC.
- M. D. Amos and P. E. Thomas, Anal. Chim. Acta, 1965, 32, 139–147 CrossRef CAS.
- D. A. Bohm, C. S. Stachel and P. Gowik, Anal. Chim. Acta, 2010, 672, 103–106 CrossRef CAS PubMed.
- M. D. Allendorf, C. A. Bauer, R. K. Bhakta and R. J. T. Houk, Chem. Soc. Rev., 2009, 38, 1330–1352 RSC.
- T. Rasheed and F. Nabeel, Coord. Chem. Rev., 2019, 401, 213065 CrossRef CAS.
- Y. Liu, X. Y. Xie, C. Cheng, Z. S. Shao and H. S. Wang, J. Mater. Chem. C, 2019, 7, 10743–10763 RSC.
- Y. Yu, Y. Wang, H. Yan, J. Lu, H. Liu, Y. Li, S. Wang, D. Li, J. Dou, L. Yang and Z. Zhou, Inorg. Chem., 2020, 59, 3828–3837 CrossRef CAS PubMed.
- S. Pramanik, C. Zheng, X. Zhang, T. J. Emge and J. Li, J. Am. Chem. Soc., 2011, 133, 4153–4155 CrossRef CAS PubMed.
- X. Zhang, X. Luo, N. Zhang, J. Wu and Y.-Q. Huang, Inorg. Chem. Front., 2017, 4, 1888–1894 RSC.
- N. C. Burtch, H. Jasuja and K. S. Walton, Chem. Rev., 2014, 114, 10575–10612 CrossRef CAS PubMed.
- C. Wang, X. Liu, N. Keser Demir, J. P. Chen and K. Li, Chem. Soc. Rev., 2016, 45, 5107–5134 RSC.
- M. Ding, X. Cai and H. L. Jiang, Chem. Sci., 2019, 10, 10209–10230 RSC.
- G. Férey, C. Mellot-Draznieks, C. Serre, F. Millange, J. Dutour, S. Surblé and I. Margiolaki, Science, 2005, 309, 2040–2042 CrossRef PubMed.
- H. Jiang, K. Yang, X. Zhao, W. Zhang, Y. Liu, J. Jiang and Y. Cui, J. Am. Chem. Soc., 2021, 143, 390–398 CrossRef CAS PubMed.
- K. Wang, H. Huang, X. Zhou, Q. Wang, G. Li, H. Shen, Y. She and C. Zhong, Inorg. Chem., 2019, 58, 5725–5732 CrossRef CAS PubMed.
- H. Jasuja and K. S. Walton, Dalton Trans., 2013, 42, 15421–15426 RSC.
- H. Jasuja, Y. Jiao, N. C. Burtch, Y. G. Huang and K. S. Walton, Langmuir, 2014, 30, 14300–14307 CrossRef CAS PubMed.
- G. M. Sheldrick, Acta Crystallogr C Struct. Chem., 2015, 71, 3–8 CrossRef PubMed.
- L. Carlucci, G. Ciani, D. M. Proserpio and S. Rizzato, Chem.–Eur. J., 2002, 8, 1520–1526 CrossRef.
- H. Kim and M. P. Suh, Inorg. Chem., 2005, 44, 810–812 CrossRef CAS PubMed.
- X.-M. Guo, Y.-N. Yan, H.-d. Guo and N. Wang, Inorg. Chem. Commun., 2015, 61, 105–108 CrossRef CAS.
- J. Li, Y. Ren, C. Qi and H. Jiang, Dalton Trans., 2017, 46, 7821–7832 RSC.
- A. L. Spek, Acta Crystallogr D Biol. Crystallogr., 2009, 65, 148–155 CrossRef CAS PubMed.
- K. Roztocki, F. Formalik, A. Krawczuk, I. Senkovska, B. Kuchta, S. Kaskel and D. Matoga, Angew. Chem., Int. Ed., 2020, 59, 4491–4497 CrossRef CAS PubMed.
- J.-J. Cheng, Y.-T. Chang, C.-J. Wu, Y.-F. Hsu, C.-H. Lin, D. M. Proserpio and J.-D. Chen, CrystEngComm, 2012, 14, 537–543 RSC.
- W. P. Lustig, S. Mukherjee, N. D. Rudd, A. V. Desai, J. Li and S. K. Ghosh, Chem. Soc. Rev., 2017, 46, 3242–3285 RSC.
- Y. Guo, X. Feng, T. Han, S. Wang, Z. Lin, Y. Dong and B. Wang, J. Am. Chem. Soc., 2014, 136, 15485–15488 CrossRef CAS PubMed.
- S. Dawood, A. Dorris, K. Davis, N. I. Hammer and H. Rathnayake, J. Phys. Chem. C, 2021, 125, 792–802 CrossRef CAS.
- P. Majee, D. K. Singha, S. K. Mondal and P. Mahata, Photochem. Photobiol. Sci., 2019, 18, 1110–1121 CrossRef CAS PubMed.
- M. Arici, New J. Chem., 2019, 43, 3690–3697 RSC.
- L.-H. Cao, F. Shi, W.-M. Zhang, S.-Q. Zang and T. C. Mak, Chem.–Eur. J., 2015, 21, 15705–157112 CrossRef CAS PubMed.
- C.-X. Yang, H.-B. Ren and X.-P. Yan, Anal. Chem., 2013, 85, 7441–7446 CrossRef CAS PubMed.
- S.-Y. Zhu and B. Yan, Dalton Trans., 2018, 47, 1674–1681 RSC.
- X.-M. Tian, S.-L. Yao, C.-Q. Qiu, T.-F. Zheng, Y.-Q. Chen, H. Huang, J.-L. Chen, S.-J. Liu and H.-R. Wen, Inorg. Chem., 2020, 59, 2803–2810 CrossRef CAS PubMed.
- M. Wang, L. Guo and D. Cao, Sens. Actuators, B, 2018, 256, 839–845 CrossRef CAS.
- Q. F. Zhang, A. J. Geng, H. N. Zhang, F. L. Hu, Z. H. Lu, D. Z. Sun, X. L. Wei and C. L. Ma, Chem.–Eur. J., 2014, 20, 4885–4890 CrossRef CAS PubMed.
- Z.-Q. Shi, Z.-J. Guo and H.-G. Zheng, Chem. Commun., 2015, 51, 8300–8303 RSC.
- W. Yan, C. Zhang, S. Chen, L. Han and H. Zheng, ACS Appl. Mater. Interfaces, 2017, 9, 1629–1634 CrossRef CAS PubMed.
- Y. Du, H. Yang, R. Liu, C. Shao and L. Yang, Dalton Trans., 2020, 49, 13003–13016 RSC.
- B. Wang, X.-L. Lv, D. Feng, L.-H. Xie, J. Zhang, M. Li, Y. Xie, J.-R. Li and H.-C. Zhou, J. Am. Chem. Soc., 2016, 138, 6204–6216 CrossRef CAS PubMed.
- X.-D. Zhu, K. Zhang, Y. Wang, W.-W. Long, R.-J. Sa, T.-F. Liu and J. Lü, Inorg. Chem., 2018, 57, 1060–1065 CrossRef CAS PubMed.
- C. Li, C. Zeng, Z. Chen, Y. Jiang, H. Yao, Y. Yang and W. T. Wong, J. Hazard. Mater., 2020, 384, 121498 CrossRef CAS PubMed.
- C. Li, W. Yang, X. Zhang, Y. Han, W. Tang, T. Yue and Z. Li, J. Mater. Chem. C, 2020, 8, 2054–2064 RSC.
Footnote |
† Electronic supplementary information (ESI) available: Crystallographic data, supplementary figures and tables. CCDC 2104369. For ESI and crystallographic data in CIF or other electronic format see DOI: 10.1039/d1ra06590d |
|
This journal is © The Royal Society of Chemistry 2021 |