DOI:
10.1039/D1RA06317K
(Paper)
RSC Adv., 2021,
11, 34456-34461
Effect of hydroxyl on antioxidant properties of 2,3-dihydro-3,5-dihydroxy-6-methyl-4H-pyran-4-one to scavenge free radicals†
Received
20th August 2021
, Accepted 12th October 2021
First published on 25th October 2021
Abstract
It is well known that 2,3-dihydro-3,5-dihydroxy-6-methyl-4H-pyran-4-one (DDMP) is usually formed in the Maillard reaction and it contributes to the antioxidant properties of Maillard reaction intermediates. A series of hydroxyl group protected DDMP derivatives were synthesized to further understand the source of antioxidant activity. Antioxidant abilities of the DDMP derivatives were evaluated by scavenging the 2,2′-azinobis(3-ethylbenzothiazoline-6-sulfonate) cationic radical (ABTS˙+), 2,2′-diphenyl-1-picrylhydrazyl radical (DPPH), and galvinoxyl radical, respectively. It was found that the introduction of protecting groups to the free hydroxyl groups of DDMP decreases their reducing abilities. In particular, the hydroxyl group at the olefin position exhibited a remarkable impact on the antioxidant activity of DDMP, indicating that the unstable enol structure in the DDMP moiety is the key factor for its antioxidant activity.
1. Introduction
Since its discovery in 1912, the Maillard reaction (MR) has attracted much attention because of its influence on the color, flavor, and nutritional components of heat-treated food.1–4 Complex reactions take place to produce a wide range of compounds in the presence of reducing carbohydrates and amino acids or proteins.5–7 Previous studies have shown that both volatile compounds and high molecular weight compounds make contributions to the antioxidant activity of Maillard reaction products (MRPs).8–10 Typical heterocyclic compounds, such as pyrroles, furans, thiophenes, thiazoles and pyrazines, which were the major flavor compounds formed in the Maillard reaction, were reported to possess certain antioxidative activities.11–13 It is difficult to confirm the exact source of antioxidant activity of MRPs because of its complex composition, which is greatly affected by reactants, pH, temperature, and other factors.14 So far, the major studies on antioxidant Maillard reaction intermediates focused on reaction mixtures or fractions of these, and only a small number analysed isolated substances.
2,3-Dihydro-3,5-dihydroxy-6-methyl-4H-pyran-4-one (DDMP) is found to be produced in the intermediate stage of Maillard reaction.15–17 It also exists in natural extracts and foods, such as whole wheat bread,18 prunes,19 rose tea20 and roasted tigernut oil.21 It is usually considered that DDMP is generated through the degradation of Amadori or Heyns rearrangement products (ARPs/HRPs) via 2,3-enolization.22,23 Additionally, the strong antioxidant activity of DDMP was also reported by many researchers. Velisek found that DDMP played a key role in the antioxidant capacity of plums and prunes.19 Hu reported the major contribution of DDMP to the antioxidant activity of in glucose-histidine Maillard reaction products.24
However, the influence of substituents at DDMP moiety on the antioxidant effectiveness remains unclear. It is worth a further study on the relationship between structures and antioxidant activity of DDMP. In this work, we synthesized a series of hydroxyl group protected DDMP derivatives. The antioxidant abilities of synthesized compounds were evaluated by scavenging ABTS˙+, DPPH and galvinoxyl radical, respectively, to reveal the effect of different groups in DDMP skeleton in the generation of antioxidant effectiveness.
2. Results and discussion
2.1 Synthesis
The synthesis of DDMP was carried out as described by Kroh11 and Ma.25 As shown in Scheme 1, DDMP-5-carboxylates 2a–2d were synthesized by the reaction of DDMP with acid chloride reagents in excellent yields (88–93%) with good regioselectivity. DDMP-3-carboxylates 5a–5b were synthesized through multi-step reactions with DDMP as starting material. Dihydromaltol (6) was synthesized according to the reported literature.26
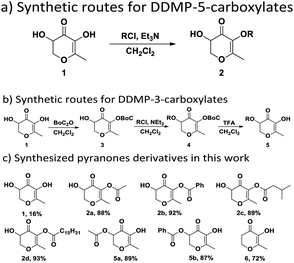 |
| Scheme 1 Synthetic routes for DDMP derivatives. | |
2.2 Scavenging ABTS˙+ radical
ABTS˙+ was usually used to test the ability of an antioxidant to reduce radicals.27–29 The results of scavenging ABTS˙+ radical by the DDMP derivatives are shown in Fig. 1. At a concentration (17.5 μM), DDMP 1 showed a good ABTS˙+ radical scavenging activity (81.1%) within one hour, better than that of butylated hydroxytoluene (BHT, 58.4%) which is often used as a free radical scavenger. DDMP exhibited stronger antioxidant capacity than reducing compounds, such as, 2-furaldehyde, 2-acetylpyrrole, 2-methylpyrazine and 2-furanmethanol generated in the Maillard reaction.13 The introduction of protecting groups to the hydroxyl groups at C-5 position of DDMP could nearly eliminate their reducing abilities. DDMP-5-carboxylates 2a–2d demonstrated poor antioxidant activities (9.1–20.3%). Compared with 2a, reactions of 2b–2d are slowed by the bulky side groups that impeded diffusion and orientation toward ABTS˙+. DDMP-3-carboxylate 5a–5b showed a moderate radical scavenging activity (44.5 and 58.5%). The percentage of 2,3-dihydromatol 6 (35.8%) is also higher than those of compounds 2a–2d. These results indicated that the hydroxyl group at the olefin position played a more important role on the antioxidant activity of DDMP.
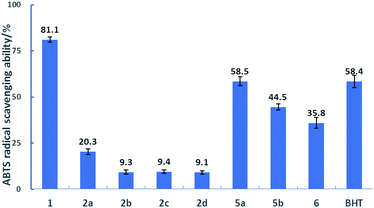 |
| Fig. 1 The percentages of scavenging ABTS+˙ radicals in the presence of 17.5 μM substrates at 25 °C for 1 hour. | |
2.3 Scavenging DPPH radical
DPPH was a nitrogen-centred radical and quenched by a hydrogen atom transferred from the antioxidant.27,30 As shown in Fig. 2, the results of scavenging DPPH radical by the DDMP derivatives are similar to those of ABTS˙+ radical. At a concentration (350 μM), DDMP 1 exhibited a great DPPH radical scavenging activity (90.7%) within one hour, comparable to that of BHT at the same concentration (87.6%). We also investigated the ability of DDMP to scavenge DPPH radicals in lipophilic solvents such as ethyl acetate and dichloromethane. The results showed that the ability of DDMP with the same concentration to scavenge DPPH radical in ethyl acetate and dichloromethane within one hour was 82.1% and 70.5%, respectively. The antioxidant capacity decreased slightly compared with that in ethanol solvent. The antioxidant capacities of 2a–2d decreased sharply with the introduction of protective groups to the free hydroxyl group at C-5 position of DDMP. Compared with 2a–2d, compounds 5a–5b and 2,3-dihydromatol 6 showed a good radical scavenging activity (75.2–87.5%), indicating that the introduction of protective groups to the free hydroxyl group at C-3 position of DDMP has a weak influence on the antioxidant activity of DDMP. Thus, the hydroxyl group at the olefin position played the main role in donating H atom to the DPPH radical.
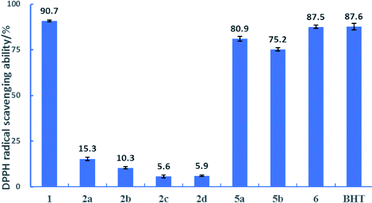 |
| Fig. 2 The percentages of scavenging DPPH radicals in the presence of 350 μM substrates at 25 °C for 1 hour. | |
2.4 Scavenging galvinoxyl radical
Galvinoxyl radical was widely employed to evaluate the ability of an antioxidant to contribute the hydrogen atom to the oxygen radical.31 As shown in Fig. 3, DDMP 1 showed a good galvinoxyl radical scavenging activity (88.7%) within one hour. The introduction of protecting groups to the hydroxyl groups at C-5 position of DDMP could nearly eliminate their reducing abilities. DDMP-5-carboxylates 2a–2d demonstrated poor antioxidant activities (6.2–12.6%). DDMP-3-carboxylates 5a–5b and 2,3-dihydromatol 6 showed moderate radical scavenging activities (46.9–53.6%). These results show that the protons of 5-hydroxyl groups are easier to transfer to oxygen radicals.
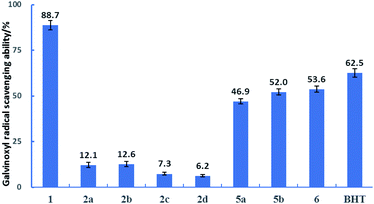 |
| Fig. 3 The percentages of scavenging galvinoxyl radical in the presence of 17.5 μM substrates at 25 °C for 1 hour. | |
2.5 Rate constant of DDMP derivatives to scavenge ABTS˙+, DPPH and galvinoxyl radicals
As DDMP and its derivatives exhibited good radical scavenging activities, the decay of the concentrations of ABTS˙+, DPPH and galvinoxyl radicals in the presence of these compounds are investigated. Fig. 4–6 outlined that the concentration of radicals decreased with the reaction period (t, in the unit of s) in the presence of DDMP and its derivatives. The concentration of radicals decreased rapidly in the first few minutes, and then decreased gradually with the increase of reaction time. According to the mathematical method established in the previous literature,32,33 the decrease of radical concentration with the reaction period (t) can be expressed by the double exponential function as shown in eqn (1). |
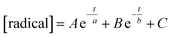 | (1) |
The differential operation is performed on eqn (1) to obtain the reaction rate (r), as shown in eqn (2). At the same time, the reaction rate (r) is also related to the concentrations of the radical and the antioxidant, in which the coefficient is the rate constant (k).
|
 | (2) |
The reaction rate at t = 0 (r0) can be calculated by eqn (2), and the rate constant, k, can be calculated by eqn (3) with the used value of every item at t = 0. The eqn (1) and (2) together with r0 in the case of DDMP and its derivatives scavenging ABTS˙+, DPPH and galvinoxyl radical are listed in Tables 1S–3S (see ESI†), only rate constants are collected in Table 1 for comparing the abilities of DDMP and its derivatives to scavenge these radicals.
|
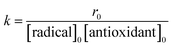 | (3) |
Table 1 The rate constants (k) for DDMP derivatives in scavenging ABTS+˙, DPPH and galvinoxyl radicals
Compound |
Rate constants forDDMP derivatives to scavenge radicals, k (mM−1 s−1) |
ABTS˙+ |
DPPH |
Galvinoxyl |
1 |
2.40 |
1.15 |
2.25 |
2a |
6.19 × 10−2 |
0.11 |
0.06 |
2b |
4.99 × 10−2 |
0.10 |
0.08 |
2c |
4.34 × 10−2 |
0.12 |
0.06 |
2d |
4.53 × 10−2 |
0.12 |
0.07 |
5a |
1.26 |
0.92 |
0.71 |
5b |
1.06 |
0.96 |
0.43 |
6 |
1.99 |
1.00 |
0.82 |
Fig. 4 and 6 outlines that the concentration of ABTS˙+ or galvinoxyl radicals decreased with the reaction period in the presence of DDMP and its derivatives. DDMP got the highest k values. The k values of 2a–2d are only one thirtieth to one fortieth of 1. After rapid initial absorbance drop, reactions are slowed by the presence of the bulky side groups that impeded diffusion and orientation toward O-centered radicals. Moreover, k values from compound 2a to 2d ranged a narrow scale, demonstrating that the introduction of different substituents on C-5 position led to similar effects on scavenging different radicals. The difference of k values of 2a–2d and 5a–5b, demonstrated that the single hydroxyl group at DDMP moiety exhibits different properties. The hydroxyl group on C-3 position cannot donate its hydrogen atom to O-centered radical as quickly as the he hydroxyl group on C-5 position. The k value of dihydromaltol (6) is little lower than that of 1, revealing that the ability of single hydroxyl group to reduce radical is lower than that of double hydroxyl groups.
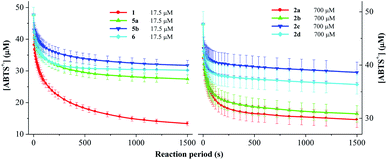 |
| Fig. 4 Decay of 47.69 μM ABTS+˙ in the presence of DDMP derivatives. | |
Fig. 5 outlines that the concentration of DPPH radical decreased with the reaction period in the presence of DDMP and its derivatives. The k values of 2a–2d are about one tenth of 1, higher than those in scavenging ABTS˙+ or galvinoxyl radicals. The k values of 5a–5b and 6 decreased only slightly. These results indicated that the chemical mechanisms were different for a redox-active radical (ABTS˙+) and for a H acceptor (DPPH and galvinoxyl radical). Hydrogen atom transfer (HAT) is dominated in the process of scavenging DPPH radicals.
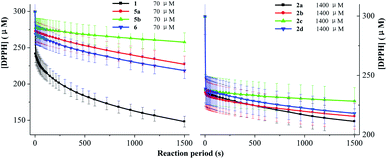 |
| Fig. 5 Decay of 299.51 μM DPPH in the presence of DDMP derivatives. | |
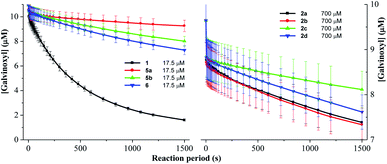 |
| Fig. 6 Decay of 10.95 μM galvinoxyl in the presence of DDMP derivatives. | |
2.6 Proposed antioxidant mechanism of DDMP
Our results show that DDMP has strong ability to scavenge free radicals. Once the C-5 position enol hydroxyl group is bonded, the scavenging ability will decrease a lot, indicating that the C-5 position hydroxyl group is crucial to its scavenging effect. Furthermore, the C-3 position hydroxyl esterified compounds 5a and 5b exhibit moderate antioxidant capacities compare to DDMP. The above information seems to indicate that the antioxidant capacity of DDMP is related to both the 5- and 3-hydroxyl groups. Early literature studies on the antioxidant properties of Maillard reaction intermediates showed that compounds with enol form have better activity, such as furaneol and maltol. It seems likely that the oxidation of reductone is initiated by the abstraction of a proton and an electron from the free hydroxyl group. DDMP itself is an unstable compound. In addition to the enol-type instability, the C-3 position hydroxyl group of DDMP is also unstable and is easily oxidized by oxidants such as chromium trioxide or even oxygen in the air. The resulting ketones are rearranged to form hydroxymaltol, which also has strong antioxidant properties due to its enolone unit. This seems to indicate that the 3-hydroxyl group is also involved in the antioxidant effect.
From the Fig. 4 and 5, we observed that when the DDMP concentration was 17.5 μM, about 34 μM ABTS˙+ free radicals were scavenged, and when the DDMP concentration was 70 μM, about 150 μM DPPH free radicals were scavenged, indicating that one molecule of DDMP can scavenge two free radicals. Correspondingly, one molecule of 5a, 5b or 6 can only scavenge one free radical. The same situation could be observed by 1H NMR spectrum similarly. When the ratio of DDMP to DPPH was 1
:
1, DDMP consumed about half, and when the ratio of DDMP to DPPH was 1
:
2, DDMP was almost completely consumed. The amount of DPPH2 produced was also twice that of the former. It could be assumed that DDMP could be oxidized two times in a row and show the 2-fold antioxidant capacity of 5a, 5b and 6.
Based on the results, the degradation pathway of DDMP proposed is shown in Scheme 2. Firstly, DDMP is oxidized to 5-hydroxyl-6-methyl-2H-pyran-3,4-dione (7), which is easily isomerized to 5-hydroxymaltol (8).34 The latter continues to be oxidized that may lead to the ring opening products. We found several carbon signals at chemical shift δ206 ∼ 197 and δ174 ∼ 168 on the 13C NMR spectrum (see ESI†), indicating that the ring opening products are mainly ketones and acids, which is consistent with the degradation pattern of reductones described in the literature.34,35
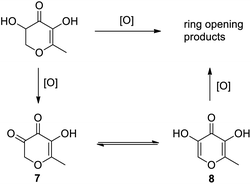 |
| Scheme 2 Proposed antioxidant mechanism of DDMP. | |
3. Conclusions
A series of hydroxyl group protected DDMP derivatives were synthesized to further understand the source of antioxidant activity. DDMP shows excellent free radical scavenging ability. The introduction of protecting groups to the free hydroxyl groups of DDMP decrease their reducing abilities. In particular, the hydroxyl group at olefin position exhibited a more remarkable impact than the hydroxyl group at C-3 position of DDMP, indicating that the unstable enol structure in the DDMP moiety is the really source of its antioxidant activity. The experiment of DDMP preventing DNA oxidation in vitro is in progress. Considering that DDMP can be generated by Maillard reaction in food it can be used as a harmless potential food additive. It provides a promising protocol for the design and synthesis of highly active antioxidant molecules in the field of food and medicine.
4. Experimental section
4.1 General considerations
Diammonium salt of 2,2′-azinobis(3-ethylbenzothiazoline-6-sulfonate) (ABTS salt), DPPH, and galvinoxyl radical were purchased from Fluka Chemie GmbH, Buchs, Switzerland. Other agents were of analytical grade and used directly. The solvents were dried and distilled prior to use by the literature methods. 1H and 13C{1H} NMR spectra were recorded on a Bruker DRX-600 spectrometer and all chemical shift values refer to δTMS = 0.00 ppm, CDCl3 ((1H), 7.26 ppm; (13C), 77.16 ppm). TLC analysis was performed by using glass-backed plates coated with 0.2 mm silica gel. Flash column chromatography was performed on silica gel (200–300 meshes). All chemical reagents were purchased from commercial sources and used as received unless otherwise indicated.
4.2 Synthesis
4.2.1 Synthesis of 2,3-dihydro-3,5-dihydroxy-6-methyl-4H-pyran-4-one (DDMP, 1). The synthesis of DDMP was carried out as described by Kanzler.11 A solution of acetic acid (11.4 mL, 0.2 mol) in 20 mL ethanol was added dropwise to a stirred mixture of D-glucose (18.0 g, 0.1 mol) and piperidine (9.2 mL, 0.1 mol) in 100 mL ethanol at 65 °C. The reaction was continued at 73 °C for 36 h. Then, the resulting mixture was evaporated under reduced pressure and the resultant residue was washed with 100 mL water. The pH of the filtrate was adjusted to 5–6 by the addition of saturated sodium bicarbonate solution. Then, the mixture was extracted with ethyl acetate at room temperature, and the organic layer was dried over sodium sulfate. After the solvent was removed under reduced pressure, the crude product was purified by silica gel column chromatography with dichloromethane and methanol (50
:
1, v/v) to afford DDMP as a yellow solid (2.3 g, 16%). 1H NMR (600 MHz, CDCl3) δ 4.49 (dd, J = 10.5, 5.9 Hz, 1H), 4.44 (dd, J = 12.1, 5.9 Hz, 1H), 4.06 (dd, J = 12.1, 10.5 Hz, 1H), 2.12 (s, 3H). 13C NMR (150 MHz, CDCl3) δ 188.1, 160.3, 131.3, 70.9, 67.1, 15.8. HR-MS-ESI m/z calcd for C6H8O4 [M + H]+ 145.0495, found 145.0494.
4.2.2 Synthesis of DDMP-5-carboxylate esters (2). Acid chloride (10.5 mmol) was added to a stirred mixture of DDMP (1.44 g, 10 mmol) and triethylamine (1.21 g, 12 mmol) in 10 mL CH2Cl2 at 0 °C. The reaction was continued for 3 h at room temperature. Then, the reaction mixture was evaporated all the volatiles under reduced pressure and the resultant residue was purified by silica gel column chromatography with petroleum ether and ethyl acetate (4/1, v/v) to afford the products 2a–2d.
4.2.3 3-Hydroxy-6-methyl-4-oxo-3,4-dihydro-2H-pyran-5-yl acetate (2a). White solid, yield 88%. 1H NMR (600 MHz, CDCl3) δ 4.57 (dd, J = 11.1, 5.9 Hz, 1H), 4.42 (dd, J = 12.5, 5.9 Hz, 1H), 4.18 (dd, J = 12.4, 11.1 Hz, 1H), 2.26 (s, 3H), 2.00 (s, 3H). 13C NMR (150 MHz, CDCl3) δ 186.6, 168.7, 168.6, 126.7, 71.2, 67.2, 20.2, 16.3. HR-MS-ESI m/z calcd for C8H10O5 [M + H]+ 187.0601, found 187.0601.
4.2.4 3-Hydroxy-6-methyl-4-oxo-3,4-dihydro-2H-pyran-5-yl benzoate (2b). White solid, yield 92%. 1H NMR (600 MHz, CDCl3) δ 8.18–8.09 (m, 2H), 7.64 (t, J = 7.5 Hz, 1H), 7.50 (t, J = 7.8 Hz, 2H), 4.65 (dd, J = 10.9, 6.2 Hz, 1H), 4.52 (dd, J = 13.5, 6.2 Hz, 1H), 4.19 (dd, J = 13.3, 11.1 Hz, 1H), 2.06 (s, 3H). 13C NMR (150 MHz, CDCl3) δ 186.5, 169.0, 164.1, 133.9, 130.4, 128.6, 128.4, 126.7, 71.2, 67.1, 16.5. HR-MS-ESI m/z calcd for C13H12O5 [M + H]+ 249.0757, found 249.0764.
4.2.5 3-Hydroxy-6-methyl-4-oxo-3,4-dihydro-2H-pyran-5-yl-3-methylbutanoate (2c). White solid, yield 89%. 1H NMR (600 MHz, CDCl3) δ 4.59 (dd, J = 10.9, 6.2 Hz, 1H), 4.44 (dd, J = 13.3, 6.2 Hz, 1H), 4.12 (dd, J = 13.3, 11.0 Hz, 1H), 2.41 (d, J = 7.7 Hz, 2H), 2.20 (dt, J = 13.6, 6.8 Hz, 1H), 2.00 (s, 3H), 1.04 (d, J = 6.7 Hz, 6H). 13C NMR (150 MHz, CDCl3) δ 186.6, 170.5, 168.7, 126.5, 71.1, 67.1, 42.6, 25.9, 22.4, 16.4. HR-MS-ESI m/z calcd for C11H16O5 [M + H]+ 229.1071, found 229.1071.
4.2.6 3-Hydroxy-6-methyl-4-oxo-3,4-dihydro-2H-pyran-5-yl palmitate (2d). White solid, yield 93%. 1H NMR (600 MHz, CDCl3) δ 4.60 (dd, J = 10.9, 6.3 Hz, 1H), 4.44 (dd, J = 13.6, 6.2 Hz, 1H), 4.11 (dd, J = 13.6, 10.9 Hz, 1H), 2.52 (t, J = 7.5 Hz, 2H), 1.99 (s, 3H), 1.81–1.60 (m, 2H), 1.46–1.12 (m, 24H), 0.88 (t, J = 7.0 Hz, 3H). 13C NMR (150 MHz, CDCl3) δ 186.6, 171.3, 168.7, 126.5, 71.0, 67.0, 33.6, 31.9, 29.7, 29.7, 29.7, 29.7, 29.6, 29.6, 29.4, 29.4, 29.2, 29.0, 24.9, 22.7, 16.3, 14.1. HR-MS-ESI m/z calcd for C22H38O5 [M + H]+ 383.2792, found 383.2794.
4.2.7 Synthesis of 3. To a mixture of DDMP (1.44 g, 10 mmol), triethylamine (1.11 g, 11 mmol) and 4-dimethylaminopyridine (0.06 g, 0.5 mmol) in THF (30 mL) was added di-tert-butyl-dicarbonate (2.30 g, 10.5 mmol). The reaction was stirred at 25 °C for 3 h. Then, the reaction mixture was evaporated under reduced pressure and the resultant residue was purified by silica gel column chromatography with petroleum ether and ethyl acetate (3/1, v/v) to afford the product 3 as a white solid (2.32 g, 95%).
4.2.8 tert-Butyl(3-hydroxy-6-methyl-4-oxo-3,4-dihydro-2H-pyran-5-yl) carbonate (3). White solid, yield 95%. 1H NMR (600 MHz, CDCl3) δ 4.59 (dd, J = 10.9, 6.2 Hz, 1H), 4.43 (dd, J = 13.5, 6.2 Hz, 1H), 4.11 (dd, J = 13.5, 10.9 Hz, 1H), 2.06 (s, 3H), 1.53 (s, 9H). 13C NMR (150 MHz, CDCl3) δ 186.6, 169.1, 151.1, 127.3, 84.2, 71.1, 67.1, 27.5, 16.3. HR-MS-ESI m/z calcd for C11H16O6 [M + Na]+ 267.0839, found 267.0832.
4.2.9 Synthesis of 4. To a stirred solution of 3 (0.73 g, 3.0 mmol) in 20 mL CH2Cl2 was added triethylamine (0.36 g, 3.6 mmol) and acid chloride (3.6 mmol) at 0 °C. After stirring for 3 h at room temperature, the reaction mixture was treated with saturated sodium bicarbonate solution. The separated organic layer was washed by brine, dried over sodium sulfate, and removed under reduced pressure to afford the pure product 4.
4.2.10 5-((tert-Butoxycarbonyl)oxy)-6-methyl-4-oxo-3,4-dihydro-2H-pyran-3-yl acetate (4a). Colorless oil, yield 96%. 1H NMR (600 MHz, CDCl3) δ 5.43 (dd, J = 8.8, 4.8 Hz, 1H), 4.50 (dd, J = 11.9, 4.8 Hz, 1H), 4.42 (dd, J = 11.9, 8.8 Hz, 1H), 2.16 (s, 3H), 2.08 (s, 3H), 1.53 (s, 9H). 13C NMR (150 MHz, CDCl3) δ 180.0, 169.6, 167.9, 150.9, 129.0, 84.1, 69.4, 68.0, 27.5, 20.7, 16.1. HR-MS-ESI m/z calcd for C13H18O7 [M + H]+ 287.1125, found 287.1135.
4.2.11 5-((tert-Butoxycarbonyl)oxy)-6-methyl-4-oxo-3,4-dihydro-2H-pyran-3-yl benzoate (4b). White solid, yield 92%. 1H NMR (600 MHz, CDCl3) δ 8.07–8.05 (m, 2H), 7.60–7.57 (m, 1H), 7.44 (t, J = 7.8 Hz, 2H), 5.67 (dd, J = 8.7, 4.8 Hz, 1H), 4.59 (ddd, J = 20.6, 11.9, 6.8 Hz, 2H), 2.12 (s, 3H), 1.54 (s, 9H). 13C NMR (150 MHz, CDCl3) δ 180.0, 168.0, 165.3, 151.0, 133.7, 130.1, 129.1, 128.8, 128.5, 84.1, 69.5, 68.5, 27.6, 16.2. HR-MS-ESI m/z calcd for C18H20O7 [M + H]+ 349.1282, found 349.1281.
4.2.12 Synthesis of DDMP-3-carboxylate esters (5). A mixture of 4 (1 mmol) and trifluoroacetic acid (2 mL) in 8 mL CH2Cl2 was stirred at 25 °C for 40 min. Then, the reaction mixture was evaporated all the volatiles under reduced pressure to afford the pure product 5.
4.2.13 5-Hydroxy-6-methyl-4-oxo-3,4-dihydro-2H-pyran-3-yl acetate (5a). Colorless oil, yield 89%. 1H NMR (600 MHz, CDCl3) δ 5.47 (dd, J = 7.9, 4.7 Hz, 1H), 4.38 (ddd, J = 20.1, 12.1, 6.3 Hz, 2H), 2.17 (s, 3H), 2.15 (s, 3H). 13C NMR (150 MHz, CDCl3) δ 181.6, 169.7, 158.8, 132.8, 69.1, 67.8, 20.7, 15.6. HR-MS-ESI m/z calcd for C8H10O5 [M + H]+ 187.0601, found 187.0602.
4.2.14 5-Hydroxy-6-methyl-4-oxo-3,4-dihydro-2H-pyran-3-yl benzoate (5b). Colorless oil, yield 87%. 1H NMR (600 MHz, CDCl3) δ 8.05 (dd, J = 8.3, 1.2 Hz, 1H), 7.60 (t, J = 7.5 Hz, 1H), 7.45 (t, J = 7.9 Hz, 2H), 5.71 (dd, J = 7.4, 5.2 Hz, 1H), 4.51 (dd, J = 6.3, 3.6 Hz, 1H), 2.18 (s, 1H). 13C NMR (150 MHz, CDCl3) δ 182.0, 165.5, 161.1, 133.8, 132.9, 130.4, 130.1, 128.6, 69.3, 68.2, 15.9. HR-MS-ESI m/z calcd for C13H12O5 [M + H]+ 249.0757, found 249.0761.
4.3 Scavenging radical
Scavenging DPPH, ABTS˙+ and galvinoxyl radical. A 2.0 mL of aqueous solution containing 4.0 mM ABTS and 1.41 mM K2S2O8 was kept for 20 h to form ABTS˙+ and then diluted by 100 mL of ethanol. The absorbance of ABTS˙+ was around 1.00 at 734 nm (εABTS˙+ = 1.6 × 104 M−1 cm−1). DPPH and galvinoxyl radicals were dissolved in ethanol directly. The absorbances of DPPH and galvinoxyl radicals were around 1.00 at 517 nm (εDPPH = 4.09 × 103 M−1 cm−1) and 428 nm (εgalvinoxyl = 1.4 × 105 M−1 cm−1), respectively. A certain concentration of ethanol solution of DDMP or DDMP derivatives (0.1 mL) was added to 1.9 mL of ABTS˙+, DPPH, and galvinoxyl radical solutions, respectively. The decreases of the absorbance of these radicals were recorded at 25 °C at a certain time interval.
4.4 Statistical analysis
All the data were the average value from at least three independent measurements with the experimental error within 10%. The equations were analyzed by one-way ANOVA in Origin 6.0 professional Software, and p < 0.05 indicated a significance difference.
Author contributions
Zhifei Chen and Qingfu Wang proposed and designed the experiment; Qingfu Wang and Gaolei Xi wrote the paper; Qiang Liu, Zhiwei Zhao, Bing Bai, Zhitao Sun and Lili Cai processed the data; Yufeng Fu and Yuping Ma corrected the language of the paper. All authors have read and agreed to the published version of the manuscript.
Conflicts of interest
There are no conflicts to declare.
Acknowledgements
This work was supported by Technology Center, China Tobacco Henan Industrial Co., Ltd.
Notes and references
- L. C. Maillard and C. R. Hebd, Seances Acad. Sci., 1912, 154, 66–68 CAS.
- C. Billaud and J. Adrian, Food Rev. Int., 2003, 19, 345–374 CrossRef CAS.
- M. Hellwig and T. Henle, Angew. Chem., Int. Ed., 2014, 53, 10316–10329 CrossRef CAS PubMed.
- A. Raza, H. Song, J. Raza, P. Li, K. Li and J. Yao, Food Funct., 2020, 11, 8583–8601 RSC.
- M. Starowicz and H. Zielinski, Food Rev. Int., 2019, 35, 707–725 CrossRef CAS.
- H. Y. Wang, H. Qian and W. R. Yao, Food Chem., 2011, 128, 573–584 CrossRef CAS.
- F. Wang, H. Shen, X. Yang, T. Liu, Y. Yang, X. Zhou, P. Zhao and Y. Guo, RSC Adv., 2021, 11, 27772–27781 RSC.
- K. EI-massry, A. Farouk and A. EI-Ghorab, Amino Acids, 2003, 24, 171–177 CrossRef PubMed.
- J. P. Eiserich and T. Shibamoto, J. Agric. Food Chem., 1994, 42, 1060–1063 CrossRef CAS.
- X. M. Chen and D. D. Kitts, J. Food Sci., 2011, 76, 831–837 CrossRef PubMed.
- C. Kanzler, P. T. Haase, H. Schestkowa and L. W. Kroh, J. Agric. Food Chem., 2016, 64, 7829–7837 CrossRef CAS PubMed.
- K. Yanagimoto, K. G. Lee, H. Ochi and T. Shibamoto, J. Agric. Food Chem., 2002, 50, 5480–5484 CrossRef CAS PubMed.
- X. Yu, M. Zhao, F. Liu, S. Zeng and J. Hu, LWT-Food Sci. Technol., 2013, 53, 22–28 CrossRef CAS.
- J. M. Ames, R. C. E. Guy and G. J. Kipping, J. Agric. Food Chem., 2001, 49, 4315–4323 CrossRef CAS PubMed.
- F. D. Mills, D. Weisleder and J. E. Hodge, Tetrahedron Lett., 1970, 11, 1243–1246 CrossRef.
- M. O. Kim and W. Baltes, J. Agric. Food Chem., 1996, 44, 282–289 CrossRef CAS.
- H. Li, C. J. Wu, X. Y. Tang and S. J. Yu, LWT-Food Sci. Technol., 2019, 105, 156–163 CrossRef CAS.
- Q. Bin, D. Jiang, I. H. Cho and D. G. Peterson, Flavour Fragr. J., 2012, 27, 454–458 CrossRef CAS.
- L. Cechovska, K. Cejpek, M. Konecny and J. Velisek, Eur. Food Res. Technol., 2011, 233, 367–376 CrossRef CAS.
- H. Qin, B. Li, W. Dai, C. Xiang, Y. Qin, S. Jiao and M. Zhang, J. Food Sci. Technol., 2019, 56, 4009–4015 CrossRef CAS PubMed.
- O. Lasekan, J. Sci. Food Agric., 2013, 93, 1055–1061 CrossRef CAS PubMed.
- M. Voigt and M. A. Glomb, J. Agric. Food Chem., 2009, 57, 4765–4770 CrossRef CAS PubMed.
- H. D. Alexis, A. Y. Varoujan and A. Keyhani, J. Agric. Food Chem., 1994, 42, 2519–2524 CrossRef.
- X. Yu, M. Zhao, F. Liu, S. Zeng and J. Hu, Food Res. Int., 2013, 51, 397–403 CrossRef CAS.
- Z. F. Chen, G. L. Xi, Y. F. Fu, Q. F. Wang, L. L. Cai, Z. W. Zhao, Q. Liu, B. Bai and Y. P. Ma, Food Chem., 2021, 361, 130052 CrossRef CAS PubMed.
- F. D. Mills, Carbohydr. Res., 1972, 23, 433–436 CrossRef CAS.
- K. M. Schaich, X. Tian and J. Xie, J. Funct. Foods, 2015, 14, 111–125 CrossRef CAS.
- G. L. Xi and Z. Q. Liu, Eur. J. Med. Chem., 2013, 68, 385–393 CrossRef CAS PubMed.
- G. L. Xi and Z. Q. Liu, Eur. J. Med. Chem., 2014, 86, 759–768 CrossRef CAS PubMed.
- G. L. Xi and Z. Q. Liu, Eur. J. Med. Chem., 2015, 95, 416–423 CrossRef CAS PubMed.
- G. L. Xi and Z. Q. Liu, J. Agric. Food Chem., 2015, 63, 3516–3523 CrossRef CAS PubMed.
- G. Litwinienko and K. U. Ingold, J. Org. Chem., 2005, 70, 8982–8990 CrossRef CAS PubMed.
- R. Wang and Z. Q. Liu, J. Org. Chem., 2012, 77, 3952–3958 CrossRef CAS PubMed.
- C. Kanzler, P. T. Haase and L. W. Kroh, J. Agric. Food Chem., 2014, 62, 2837–2844 CrossRef CAS PubMed.
- J. Beck, F. Ledl, M. Sengl and T. Severin, Z. Lebensm.-Unters. Forsch., 1990, 190, 212–216 CrossRef CAS PubMed.
Footnote |
† Electronic supplementary information (ESI) available. See DOI: 10.1039/d1ra06317k |
|
This journal is © The Royal Society of Chemistry 2021 |
Click here to see how this site uses Cookies. View our privacy policy here.