DOI:
10.1039/D1RA05877K
(Paper)
RSC Adv., 2021,
11, 35607-35613
Remarkable diastereomeric effect on thermoresponsive behavior of polyurethane based on lysine and tartrate ester derivatives
Received
3rd August 2021
, Accepted 23rd October 2021
First published on 3rd November 2021
1. Introduction
Thermoresponsive polymers have been developed in a wide range of molecular designs because of their potential application as smart materials.1–4 Stereoregularity is a helpful molecular design strategy for controlling thermoresponsive properties using conformational entropy. In nature, proteins can form their precise folding structure by controlling their conformations using the stereoregularity of an L-amino acid. Interestingly, the change of a particular amino acid residue from the L-form to the D-form sometimes leads to amyloid fibril formation and inhibition.5 This long-distance diastereomeric effect along polymer chains inspires a new platform for thermoresponsive polymer design because a small amount of chirality reversal is amplified into macroscopic change.
The stereoregularity of artificial polymers has been related to their thermoresponsive properties.6–10 For instance, poly(N-isopropyl acrylamide) (PNIPAm) shows different thermoresponsive behavior through its tacticity. It has been reported that syndiotactic rich PNIPAm exhibits sharp phase transition behavior due to the enhancement of cooperative hydration.7 In contrast, isotacticity of PNIPAm leads to a broader phase transition because of increasingly strong hydrogen bonding interaction within polymer chains.8,9 This different behavior in water media mostly depends on whether the hydrogen bonding of amide side chains strongly interacts with water molecules or another amide side chain.7,9,10 On the other hand, poly oligo(ethylene glycol) (OEG) methacrylate derivatives exhibit no stereoregular effect on thermoresponsive behavior except for a small difference in cloud point (Tcp).11 Furthermore, stereoregular polyether bearing OEG at the side chains also acts similarly in water media to an atactic polymer.12 Thus, the absence of strong intramolecular hydrogen bonds may desensitize the stereoregular effect despite the close distance between asymmetric carbon atoms. In addition, amino acid-based polymers have been associated with thermoresponsive helical conformation in water.13–19 Only a few studies of amino acid-based polymers have reported a macroscopic difference in turbidity due to their chirality.18,19 In short, studies on the thermoresponsive behavior in stereoregular polymers have been focused on the hydrogen bond and polymer systems based on close asymmetric atoms. The octadiene backbone polymer has been reported to exhibit a slightly different Tcp by the regio- and stereo-regularity in an example associated with the long-distance diastereomeric effect.20 However, the long-distance diastereomeric effect was not the focus of the study.
Therefore, stereoregular thermoresponsive polymers with a long distance between asymmetric carbons are an undeveloped area, and are important in deepening our understanding of thermoresponsive behavior. In this context, we previously reported thermoresponsive polyurethane (PU) consisting of hexamethylene diisocyanate (HDI) and chiral L-tartrate ester with OEG side chains.21 However, the long-distance diastereomeric effect did not appear because the chiral relationship between reported PUs was composed of an achiral diisocyanate and symmetric chiral L-/D-tartrate diols.
In this study, we propose the long-distance diastereomeric effect on thermoresponsive behavior in polyurethane diastereomers consisting of an asymmetric L-lysine-based diisocyanate (LDI) and a symmetric diol OEG L-/D-tartrate ester bearing two chiral carbons. This diastereomeric PU system is imagined as different spatial arrangements of ethyl esters in the mirror image, although the actual difference in chirality is derived from tartrates (Fig. 1). Surprisingly, the slight spatial difference of the long-range ethyl ester groups was amplified, leading to different thermoresponsive behavior in water media.
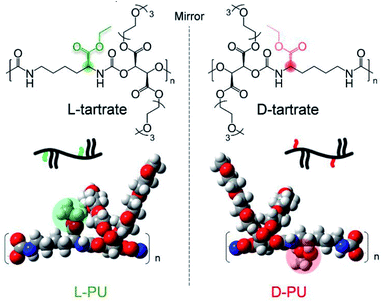 |
| Fig. 1 Chemical structure of diastereomeric water-soluble polyurethanes, L-PU and D-PU. | |
2. Experimental
2.1. Materials
L-Lysine ethyl ester diisocyanate (LDI) was purchased from Alfa Aesar Co. Dibutyltin dilaurate (DBTDL), triethylene glycol monomethyl ether (mOEG3), p-toluenesulfonic acid monohydrate (TsOH) were purchased from Tokyo Chemical Industry. L-Tartaric acid and D-tartaric acid were purchased from Nacalai Tesque, Inc. Anhydrous tetrahydrofuran (THF) and anhydrous N,N-dimethylformamide (DMF) were purchased from Kanto Chemical Co., Inc. Diethyl ether and benzene were purchase from Wako Pure Chemical Industries. The chiral diol monomer, L-OEG3TA and D-OEG3TA, were synthesized following the literature procedure21 by condensation between tartaric acid and mOEG3 using TsOH as an acid catalyst.
2.2. Polymerization for L-PU and D-PU
The general procedure was conducted according to our previous report.20 OEG3TA (1.77 g, 4 mmol) was dried in a 20 mL vial equipped with septum rubber at 80 °C overnight. Next, the vial was filled with N2 gas and injected anhydrous solvent (THF or DMF), DBTDL (1 drop), and LDI (0.8 mL, 4 mmol) with the syringe. After the mixture was stirred at 50 °C for 8 h, the polymer was precipitated into an excess amount of diethyl ether. Finally, the resultant precipitated polymer was dried in vacuo at 60 °C.
2.3. Measurements
1H NMR spectra were measured using a JEOL JNM-ECX400 apparatus. The Fourier transform infrared spectrometry (FT-IR) spectra were measured using a Shimadzu IRAffinity-1S spectrometer. Size-exclusion chromatography (SEC) was performed using a JASCO LC-2000 Plus series system equipped with a PU-2080 Plus Intelligent HPLC pump, a RI-2031 Plus Intelligent RI detector, a CO-2065 Plus Intelligent column oven, an AS-2055 Plus Intelligent Sampler, and two linearly connected commercial column (TSKgel Super3000 and GMHXL, Tosoh Corporation) using THF as an eluent at 40 °C at a flow rate of 0.1 mL min−1. The molecular weight calibration curve was calculated using polystyrene standard (Shodex). Differential Scanning Calorimetry (DSC) was performed using Hitachi DSC6200 at a rate of 2 °C min−1 for polymer aqueous solution (1/1, w/w). UV-vis transmittance curves for polymer aqueous solution was obtained on UV-2600 (Shimadzu) system at 500 nm with heating and cooling from 10 to 80 °C at a rate of 1 °C min−1. The cloud point temperature (Tcp) was defined as temperature at 50% UV-vis transmittance. Dynamic light scattering (DLS) measurements were performed on a Malvern ZEN 3600 Zetasizer Nano ZS equipped with a monochromatic coherent He–Ne laser with a fixed wavelength of 633 nm and 20 °C.
3. Results and discussion
3.1. Synthesis and characterization of diastereomeric PUs
We designed and synthesized the diastereomeric water-soluble PUs by polyaddition between commercially available L-lysine diisocyanate ethyl ester (LDI) and one-step synthetic three units of OEG L-/D-tartrate ester diol monomer (OEG3TA) (Scheme 1). The previous PUs composed of HDI and OEG3TA were difficult to control polymerization, with a number average molecular weight (Mn) up to 6500 and a large Mw/Mn of about 4.21 We attributed this problem to OEG3TA impurity mixing a small amount of mOEG3 and a low reaction rate and addressed it by changing the eluent of column chromatography and extending the polymerization time.
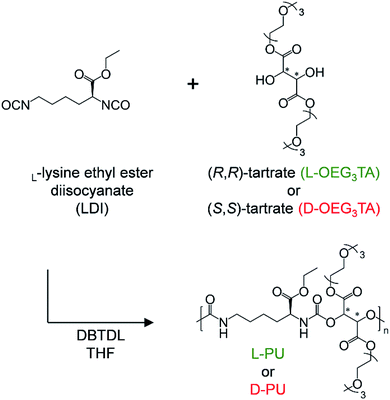 |
| Scheme 1 Synthesis of L-PU and D-PU. | |
In addition, it is noteworthy that L-lysine and tartaric acid are an abundant chiral source and have low toxicity due to their natural origin. This novel chiral PU system is the diastereomeric relationship derived from only the ethyl ester spatial position under the mirror image. According to the Ramachandran plot, this slight spatial position difference probably amplifies the conformational behavior because the lysine residue prefers twisted dihedral angles are prone to form a helix structure.22
Synthesized PUs based on L-/D-tartrate ester, named L-PU and D-PU, were characterized by 1H NMR, SEC, and FT-IR. Fig. 2 shows 1H NMR spectra of L-PU and D-PU. As to our previous reports,21 the methine proton peak j adjacent to the alcohol group in OEG3TA shifted from 4.7 ppm to 5.7 ppm with the formation of urethane bonds. There was no noticeable spectral difference between L-PU and D-PU because of the chemical compositional equivalent (Fig. 2a and b). In addition, since end group peaks observed in the previous work were not detected, the resultant PUs were successfully polymerized with high Mn than the previous OEG3TA based PU. Fig. 3 shows the SEC profiles with polystyrene equivalent for L-PU and D-PU. Polymerization was successfully controlled, as the Mw/Mn and Mn were about 2 for all PUs and up to 13
100. We polymerized these diastereomeric PUs with a different Mn, ranging from 4700 to 13
100 to highlight the diastereomeric effect on thermoresponsive behavior. In the following discussion, we compared PUs with almost the same average molecular weight and dispersity samples (L-PU: Mn = 7,100, Mw/Mn = 1.76 and D-PU: Mn = 7,500, Mw/Mn = 1.49).
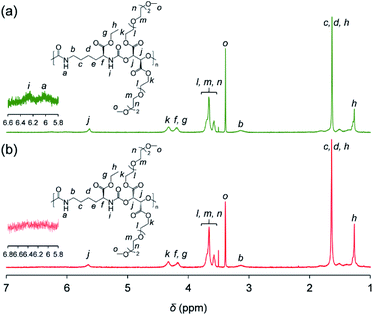 |
| Fig. 2 1H NMR (400 MHz) spectra of (a) L-PU and (b) D-PU in CDCl3. | |
Next, we characterized FT-IR spectra for L-PU and D-PU to confirm the formation of urethane bonds and their hydrogen bonding (Fig. 4a).23–27 Both L-PU and D-PU exhibited the N–H and C
O stretching vibration peaks around 3300 and 1730 cm−1 in FT-IR spectra, suggesting the urethane bond formation. In addition, the FT-IR peaks of the ether C–O–C bonds in the OEG chains the urethane C–N bonds supported the successful synthesis of the desired PUs bearing OEG side chains. Furthermore, we attributed the C
O stretching vibration peaks to the free and hydrogen-bonded ester bonds at around 1768 and 1730 cm−1, free and hydrogen-bonded urethane bonds at around 1730 and 1708 cm−1, according to the literature (Fig. 4b).25–27 The broad peak at around 1730 cm−1 probably contains the hydrogen-bonded ester C
O and the free urethane stretching vibration with slightly different peak tops. We considered the urethane hydrogen bonding by the area of the C
O stretching vibration. Both L-PU and D-PU showed the small area of the hydrogen-bonded urethane C
O stretching vibration peaks (<10%), which are much lower than that of a series of our previous HMDI-based PUs.27 This is because the steric repulsion of ethyl and OEG ester groups on the carbon adjacent to the urethane bond prevents hydrogen bonding between the urethane bonds. However, though the C
O stretching vibration peaks of L-PU and D-PU were almost identical, D-PU showed a slightly smaller free ester bond shoulder proportion than L-PU (Fig. 4c). Considering that the Mn of L-PU and D-PU is comparable, the ratio of urethane bonds to ester bonds should match between L-PU and D-PU. Thus, the difference in the proportion of the free ester C
O stretching vibration at 1768 cm−1 is caused by shifting to the hydrogen-bonded ester C
O stretching vibration. In other words, D-PU has more hydrogen-bonded ester bonds. This reflects the difference in interaction based on the ethyl ester groups' spatial arrangement in mirror image, leading to a unique diastereomeric effect on thermoresponsive properties.
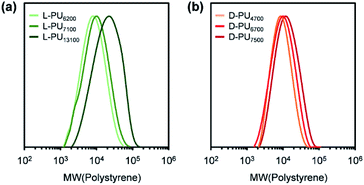 |
| Fig. 3 SEC profiles for L-PU and D-PU with polystyrene equivalent using THF as an eluent. | |
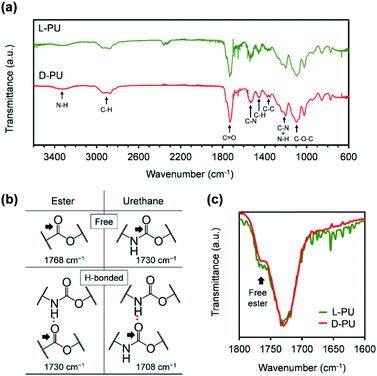 |
| Fig. 4 (a) FT-IR spectra for L-PU and D-PU. (b) Assignment of the C O stretching vibration. (c) Enlarged spectra showing the C O stretching vibration region for L-PU and D-PU. | |
3.2. Thermoresponsive behavior of diastereomeric PUs
First, we compared the turbidimetry of L-PU and D-PU. Fig. 5a and b show UV-vis transmittance curves of 500 nm light for L-PU and D-PU in 2 g L−1 aqueous solution from 10 to 80 °C at a rate of 1 °C min−1. Surprisingly, we observed an entirely different thermoresponsive behavior between the L-PU and D-PU diastereomers. The transmittance curve of the L-PU was steep in the range of 5–10 °C, comparable to that of well-studied typical thermoresponsive polymers, including PNIPAm and POEGMA.28 On the other hand, the D-PU showed a gentle transmittance curve, not to reach 0%T at even 80 °C. We observed the same tendency in the steepness of L-PU and D-PU transmittance curves in all samples with different Mn (Fig. 5c and d).
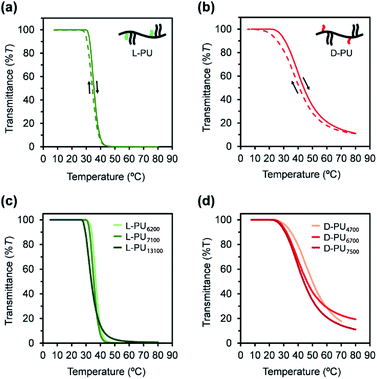 |
| Fig. 5 UV-vis transmittance curves for the (a) L-PU and (b) D-PU (Mn ∼7000) with 2 g L−1 aqueous solution in heating cycle (solid line) and cooling cycle (dotted line). UV-vis transmittance curves for aqueous solution of (c) L-PU and (d) D-PU with different Mn. | |
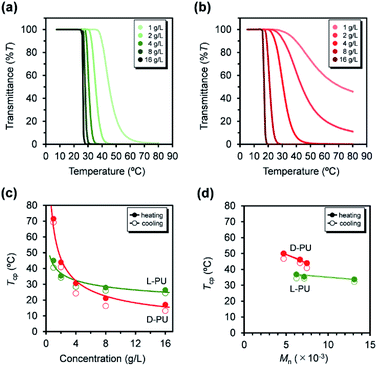 |
| Fig. 6 UV-vis transmittance curves at various concentrations from 1 to 16 g L−1 for (a) L-PU and (b) D-PU. Plots of the measured temperature at cloud point (Tcp) for L-PU and D-PU as a function of (c) polymer concentration in water and (d) the number average molecular weight (Mn) of polymer aqueous solution at 2 g L−1 (solid line, heating; dotted line, cooling). | |
In addition, we investigated the concentration effect from 1 g L−1 to 16 g L−1 on UV-vis transmittance of L-PU and D-PU in aqueous solutions during the heating process (Fig. 6a and b). Both L-PU and D-PU showed a similar steep phase transition at the high concentration, and their cloud point (Tcp) was 28 °C and 19 °C at 16 g L−1. However, for the dilute region, L-PU still showed steep transition curves at up to 2 g L−1, whereas D-PU lost steepness below 4 g L−1. This apparent difference in the transmittance curves depending on concentration is related to the polymer agglutination process. We compared the Tcp plots for each concentration of L-PU and D-PU (Fig. 6c). The phase boundary curve of D-PU decreased more steeply with increasing concentration than the L-PU counterpart. However, the decaying decrease in the L-PU and D-PU phase boundary curves differed from the stereoregular PNIPAm. According to the literature, the meso-rich PNIPAm consisting of 60% of meso carbon showed an almost plateau phase boundary curve, whereas the 46% counterpart showed a monotonically decreasing curve with concentration.6,9,29 Here, the monotonic decrease of Tcp with concentration is associated with a cooperative hydration structure leading to a steep UV-vis transmittance curve. In short, the plateau phase boundary curve of only meso rich PNIPAm tends to result in broad UV-vis transmittance curves. However, when the comparison is only above the 10 g L−1 region, the boundary curve of D-PU is a plateau rather than monotonically decreasing, suggesting that both L-PU and D-PU do not form cooperative hydration. Therefore, the difference in thermoresponsive behavior between the L-PU and D-PU may not be due to the cooperative hydrogen bond. Furthermore, to generalize the diastereomeric effect between L-PU and D-PU, we investigated the molecular weight effect, which influences thermoresponsive behavior. Table 1 summarized the Tcp of different Mn samples from 6200 to 13
100 for L-PU and from 4700 to 7500 for D-PU with Mw/Mn controlled around 2. Fig. 6d shows plots of Tcp as a function of Mn. The Tcp for L-PU and D-PU does not overlap on the plots. Moreover, the molecular weight dependence of D-PU was more significant than L-PU, suggesting that D-PU is affected by the end group and particle size.
Table 1 Summary of thermoresponsive properties for chiral polyurethanes
Entry |
Sample |
Mna (×10−3) |
Mw/Mn |
Tcpb (°C) |
Determined by SED based on PS standard, eluted by THF. Determined by 50% transmittance for 2 g L−1 polymer aqueous solution on UV-vis transmittance curves at a heating rate of 1 °C min−1. |
A |
L-PU6200 |
6.2 |
1.64 |
37 |
B |
L-PU7100 |
7.1 |
1.76 |
35 |
C |
L-PU13100 |
13.1 |
1.64 |
34 |
D |
D-PU4700 |
4.7 |
1.98 |
50 |
E |
D-PU6700 |
6.7 |
2.16 |
46 |
F |
D-PU7500 |
7.5 |
1.58 |
44 |
The L-PU and D-PU 2 g L−1 aqueous solution also showed different salting-out effects with NaCl. Tcp of L-PU gradually decreased for NaCl concentration, comparable to PNIPAm and previous thermoresponsive PUs consisting of HDI and OEG3TA (Fig. 7a and c).21,28 On the other hand, the Tcp of D-PU showed a steep decrease at low NaCl concentration and moderated as the concentration increased (Fig. 7b and d). According to the literature, the preferred cation coordination to the amide group in PNIPAm partially offsets the salting-out effect.30 Based on this idea, the Na+ cations may not reach the coordination sites of ester and urethane groups in D-PU at the low concentration, and the salting-out is not sufficiently offset. Therefore, we speculated that D-PU particles have weak inter-particle interaction due to urethane groups embedded inside.
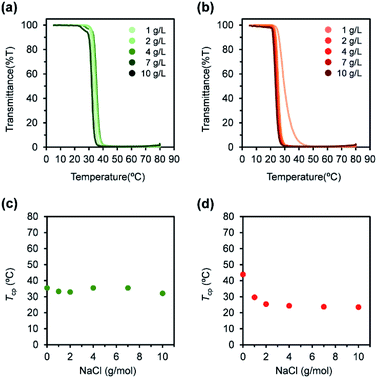 |
| Fig. 7 UV-vis transmittance curves for (a) L-PU and (b) D-PU with different NaCl concentration. Plots of the measured temperature at cloud point (Tcp) for (c) L-PU and (d) D-PU with different NaCl concentration. Polymer concentration in water was 2 g L−1. | |
3.3. Differential scanning calorimetry
Next, we considered the microscopic thermoresponsive mechanism using differential scanning calorimetry (DSC) to understand the different macroscopic turbidimetry of L-PU and D-PU. DSC reflects a phase transition behavior as an endothermic peak caused by the dehydration process surrounding thermoresponsive polymers. Fig. 8a and b show DSC curves for aqueous solutions of L-PU and D-PU. We observed broad endotherm peaks at 38 °C and 29 °C for L-PU and D-PU, respectively. The enthalpy of the monomer units along the chain was estimated to be 0.52 and 0.42 kcal mol−1 for L-PU and D-PU, respectively. These enthalpy values are lower than hydrogen bonding PNIPAm, typically with a 0.8–1.9 kcal mol−1, similar to non-hydrogen bonding POEGMA derivatives.31–34 Moreover, the peak shapes of L-PU and D-PU are broad and almost the same, which is identical to POEGMA.27 Therefore, the hydrophobicity of the OEG side chains rather than the hydrogen bonds of the urethane bonds may be dominantly involved in the aggregation process. According to the literature,7 the cooperative hydration of syndiotactic rich PNIPAm shows sharper endothermic peaks than isotactic rich PNIPAm. Thus, L-PU and D-PU probably do not have a cooperative hydration because of the lysine alkyl spacer. From the above, the difference in visible turbidity is not ascribe to cooperative hydration on the molecular scale as in PNIPAm.
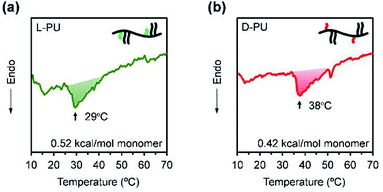 |
| Fig. 8 DSC curves for (a) L-PU and (b) D-PU 50 wt% aqueous solution. | |
3.4. Dynamic light scattering
To clarify the aggregation process of L-PU and D-PU on the particle scale, we measured the particle size at the temperature at each transmittance (%T = 90, 50, 0, or 15) by dynamic light scattering (DLS). The aggregation process of L-PU is similar to traditional thermoresponsive hydrogen-bonding polymers that significantly change their hydrodynamic diameter (Dh) ∼1000 nm over Tcp (Fig. 9a).16,35 At 32 °C, corresponding to 90%T of 2 g L−1 aqueous solutions, L-PU exhibited broad and bimodal peaks that included particles of many sizes because of insufficient particle interaction. Above 35 °C, L-PU showed a monomodal aggregate peak and gradually increased in size depending on the transmittance. On the other hand, the D-PU showed almost constant Dh ∼200 nm over a wide temperature range (Fig. 9b). D-PU showed a monomodal peak in 160 nm even at 90% transmittance whereas L-PU was bimodal. Even raising the temperature to 70 °C, the Dh of D-PU only increased to 210 nm. Retaining the Dh at high temperatures supports a strong dependence of polymer and NaCl concentration on UV-vis transmittance, which is attractive in designing colloidal stability using chirality. This stable Dh of D-PU is due to the strength of the interaction of the particles after dehydration. Indeed, polymers consisting of OEG tend to aggregate to a Dh ∼200–300 nm ceiling because the driving force is only hydrophobic interaction.36,37 On the basis of the results mentioned above, we propose a possible mechanism for the long-distance diastereomeric effect on thermoresponsive behavior (Fig. 9c). Here, we focused on the growth process of the formed micelles Dh ∼100 nm. The L-PU aggregates efficiently once a small micelle appears due to more vital interparticle interaction than D-PU. On the other hand, D-PU forms weak interparticle interaction particles up to the Dh ∼200 nm ceiling despite agglomerating earlier than L-PU. According to the literature,38–40 when a dehydrated polymer chain end leaves from a micelle by thermal motion, the chain is likely to migrate to another micelle and lead to intermicellar bridging. Given that this process is accelerated, particle size grows to form an agglomerate with a huge particle size (Dh ∼1000 nm). Thus, L-PU promoted intermicellar bridging because of more easily releasing polymer chains from the micelles, whereas D-PU kept polymer chains in the micelle and remained stable micelle size. This assumption was supported by the higher proportion of hydrogen-bonded ester of D-PU than that of L-PU in FT-IR spectra. Therefore, the slight difference in the ethyl ester group spatial arrangement was reflected in the retention of the dehydrated polymer chain in the micelle via hydrogen bonding to the ester group, resulting in the long-distance diastereomeric effect.
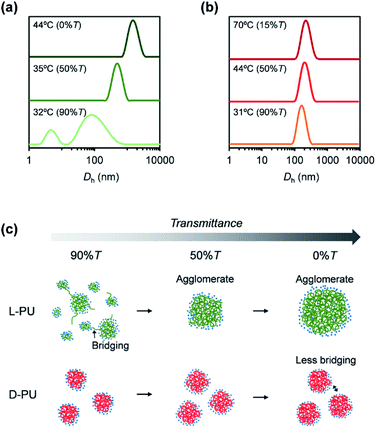 |
| Fig. 9 Temperature-dependent DLS traces for (a) L-PU and (b) D-PU in water at a concentration of 2 g L−1. (c) Schematic representation for the possible thermoresponsive mechanism of L-PU and D-PU during turbidimetry. | |
4. Conclusions
In conclusion, we found a long-distance diastereomeric effect on thermoresponsive behavior in chiral PUs consisting of L-lysine diisocyanate and L-/D-OEG tartrate ester diol. L-PU and D-PU displayed the clearly different thermoresponsive behavior on UV-vis transmittance despite the lysine alkyl spacer. L-PU showed steep transmittance curves (<10 °C) over a wide range of concentrations (2–16 g L−1), whereas D-PU was broad (>10 °C) below 4 g L−1 concentration. The DSC curves of L-PU and D-PU showed small endothermic peaks with almost the same enthalpy (L-PU: ΔH = 0.52 kcal mol−1 and D-PU: ΔH = 0.42 kcal mol−1), suggesting that the dehydration process is the same on the molecular scale almost without the cooperative hydration. Furthermore, DLS showed that the particle size of D-PU was nearly constant over a wide range of temperatures (31–70 °C). In contrast, L-PU significantly increased in particle size, consistent with typical thermoresponsive polymers. Thus, the interparticle interactions were altered by the slight chirality of the ethyl groups, producing differences in turbidimetry. This study is the first report of the long-distance diastereomeric effect reflected in macroscopic thermoresponsive behavior despite the flexible lysine spacer. Given the ease of introducing chirality into step-growth polymerization systems, the long-distance diastereomeric effect is expected to be a new platform for molecular design.
Conflicts of interest
There are no conflicts to declare.
Acknowledgements
This work was supported by the Grant-in-Aid for JSPS Fellows, JP19J15174. This work was also supported by JSPS KAKENHI Grant Numbers JP19KK0277, JP20H02799, JP20H05223, and JPJSBP120189206.
Notes and references
- A. Bordat, T. Boissenot, J. Nicolas and N. Tsapis, Adv. Drug Delivery Rev., 2019, 138, 167 CrossRef CAS PubMed.
- M. A. C. Stuart, W. T. Huck, J. Genzer, M. Müller, C. Ober, M. Stamm and S. Minko, Nat. Mater., 2010, 9, 101 CrossRef PubMed.
- D. Roy, W. L. Brooks and B. S. Sumerlin, Chem. Soc. Rev., 2013, 42, 7214 RSC.
- D. Schmaljohann, Adv. Drug Delivery Rev., 2006, 58, 1655 CrossRef CAS PubMed.
- T. Ban, M. Hoshino, S. Takahashi, D. Hamada, K. Hasegawa, H. Naiki and Y. Goto, J. Mol. Biol., 2004, 344, 757 CrossRef CAS PubMed.
- A. Halperin, M. Kröger and F. M. Winnik, Angew. Chem., Int. Ed., 2015, 54, 15342 CrossRef CAS PubMed.
- T. Mori, T. Hirano, A. Maruyama, Y. Katayama, T. Niidome, Y. Bando and Y. Maeda, Langmuir, 2009, 25, 48 CrossRef CAS PubMed.
- B. Ray, Y. Okamoto, M. Kamigaito, M. Sawamoto, K. I. Seno, S. Kanaoka and S. Aoshima, Polym. J., 2005, 37, 234 CrossRef CAS.
- Y. Katsumoto and N. Kubosaki, Macromolecules, 2008, 41, 5955 CrossRef CAS.
- K. Nishi, T. Hiroi, K. Hashimoto, K. Fujii, Y. S. Han, T. H. Kim and M. Shibayama, Macromolecules, 2013, 46, 6225 CrossRef CAS.
- S. Han, M. Hagiwara and T. Ishizone, Macromolecules, 2003, 36, 8312 CrossRef CAS.
- T. Isono, K. Miyachi, Y. Satoh, S. I. Sato, T. Kakuchi and T. Satoh, Polym. Chem., 2017, 8, 5698 RSC.
- K. Bauri, M. Nandi and P. De, Polym. Chem., 2018, 9, 1257 RSC.
- C. Chen, Z. Wang and Z. Li, Biomacromolecules, 2011, 12, 2859 CrossRef CAS PubMed.
- G. Hu, W. Li, Y. Hu, A. Xu, J. Yan, L. Liu and A. Zhang, Macromolecules, 2013, 46, 1124 CrossRef CAS.
- G. Shi, S. Wang, X. Guan, J. Zhang and X. Wan, Chem. Commun., 2018, 54, 12081 RSC.
- F. Wang, C. Zhou, K. Liu, J. Yan, W. Li, T. Masuda and A. Zhang, Macromolecules, 2019, 52, 8631 CrossRef CAS.
- T. Aoki, M. Muramatsu, T. Torii, K. Sanui and N. Ogata, Macromolecules, 2001, 34, 3118 CrossRef CAS.
- S. Kuroyanagi, N. Shimada, S. Fujii, T. Furuta, A. Harada, K. Sakurai and A. Maruyama, J. Am. Chem. Soc., 2018, 141, 1261 CrossRef PubMed.
- M. Li, F. Cui, Y. Li, Y. Tao and X. Wang, Macromolecules, 2016, 49, 9415 CrossRef CAS.
- D. Aoki and H. Ajiro, Macromol. Rapid Commun., 2018, 39, 1800239 CrossRef.
- P. Chakrabarti and D. Pal, Prog. Biophys. Mol. Biol., 2001, 76, 1 CrossRef CAS.
- A. V. Raghu, H. M. Jeong, J. H. Kim, Y. R. Lee, Y. B. Cho and K. Sirsalmath, Macromol. Res., 2008, 16, 194 CrossRef CAS.
- A. V. Raghu, G. S. Gadaginamath, M. Priya, P. Seema, H. M. Jeong and T. M. Aminabhavi, J. Appl. Polym. Sci., 2008, 110, 2315 CrossRef CAS.
- W. B. Fischer, P. Pötschke, G. Pompe, K. J. Eichhorn and H. W. Siesler, Macromol. Chem. Phys., 1997, 198, 2057 CrossRef CAS.
- B. Fernández d'Arlas, L. Rueda, K. De la Caba, I. Mondragon and A. Eceiza, Polym. Eng. Sci., 2008, 48, 519 CrossRef.
- D. Aoki and H. Ajiro, Polym. Chem., 2021, 12, 1533 RSC.
- J. F. Lutz, O. Akdemir and A. Hoth, J. Am. Chem. Soc., 2006, 128, 13046 CrossRef CAS PubMed.
- Y. Okada and F. Tanaka, Macromolecules, 2005, 38, 4465 CrossRef CAS.
- E. Bruce, H. I. Okur, S. Stegmaier, C. I. Drexler, B. A. Rogers, N. F. van der Vegt and P. S. Cremer, J. Am. Chem. Soc., 2020, 142, 19094 CrossRef CAS PubMed.
- Y. Xia, N. A. Burke and H. D. Stöver, Macromolecules, 2006, 39, 2275 CrossRef CAS.
- S. Sun and P. Wu, Macromolecules, 2013, 46, 236 CrossRef CAS.
- G. B. Chua, P. J. Roth, H. T. Duong, T. P. Davis and A. B. Lowe, Macromolecules, 2012, 45, 1362 CrossRef CAS.
- H. Ajiro, Y. Takahashi and M. Akashi, Macromolecules, 2012, 45, 2668 CrossRef CAS.
- T. Maeda, M. Takenouchi, K. Yamamoto and T. Aoyagi, Polym. J., 2009, 41, 181 CrossRef CAS.
- J. F. Lutz, K. Weichenhan, Ö. Akdemir and A. Hoth, Macromolecules, 2007, 40, 2503 CrossRef CAS.
- S. Bolisetty, C. Schneider, F. Polzer, M. Ballauff, W. Li, A. Zhang and A. D. Schlüter, Macromolecules, 2009, 42, 7122 CrossRef CAS.
- Q. T. Pham, W. B. Russel, J. C. Thibeault and W. Lau, Macromolecules, 1999, 32, 2996 CrossRef CAS.
- S. Honda, T. Yamamoto and Y. Tezuka, J. Am. Chem. Soc., 2010, 132, 10251 CrossRef CAS.
- S. Honda, T. Yamamoto and Y. Tezuka, Nat. Commun., 2013, 4, 1 Search PubMed.
|
This journal is © The Royal Society of Chemistry 2021 |
Click here to see how this site uses Cookies. View our privacy policy here.