DOI:
10.1039/D1RA05764B
(Paper)
RSC Adv., 2021,
11, 33276-33287
Effects of external electric field on the sensing property of volatile organic compounds over Janus MoSSe monolayer: a first-principles investigation†
Received
29th July 2021
, Accepted 27th September 2021
First published on 11th October 2021
Abstract
Janus 2D transition metal dichalcogenide (TMD) is a new generation 2D material with a unique asymmetric structure. This asymmetric structure (or out-off plane symmetric geometry) of Janus 2D TMD has been reported to yield tunable electronic properties through strain and electric field, which can also be applied in gas sensing. In this work, we performed DFT calculations to investigate the gas sensing property of cyclohexane and acetone on MoS2 and Janus MoSSe monolayers under external electric fields. Our results show that cyclohexane possesses slightly larger adsorption energy on pristine MoS2 and Janus MoSSe monolayers than acetone without external electric fields. After applying the external electric fields, the adsorption energy for cyclohexane on MoS2 shows no enhancement. However, the adsorption energy of acetone shows the most substantial enhancement on the Janus MoSSe monolayer. We found that the dipole moment orientations of adsorbates and the monolayer can strongly interact with the external electric fields. Hence, the combination of polar adsorbate and polar material, i.e., acetone and Janus MoSSe, demonstrates the most vital sensitivity under the applied bias. On the other hand, the non-polar adsorbate and non-polar material combination show a negligible effect on external bias. These findings can be applied to the design of gas sensors in the future through polar materials.
1. Introduction
Since the discovery of graphene, two-dimensional (2D) materials have gradually attracted significant attention due to their many unique properties, which are distinct from the 3D bulk materials.1–9 Transition metal dichalcogenides (TMD) are one of the most widely studied 2D materials due to their specific electrical, optical, and other physical properties.10–14 Recently, Janus 2D TMD materials have gradually attracted considerable interest and have two different atom layers on two sides to generate an out-off plane symmetry of its geometry. MoSSe, a single-layer Janus 2D TMD, has been successfully fabricated experimentally through the modified CVD method,15,16 although Janus MoSSe does not exist in nature. Theoretically, the MoSSe monolayer has been demonstrated to be stable by calculating the phonon dispersion and through molecular dynamics simulations.17 Compared with the traditional 2D TMD monolayers, Janus 2D TMD monolayers have many advantages based on their unique electronic properties, such as tunable band-gap, Rashba effect, and piezoelectric effect. In addition, it has been recently reported that Janus 2D TMD monolayers can be applied in many fields, including catalysts,18 transistors,19 optoelectronic devices,20 and sensors.21 Recently, there have been many studies regarding the gas sensing properties using the Janus 2D TMD monolayers. For example, using first-principle calculations, Janus MoSSe monolayers have been studied in the sensing NO2, NH3, SOF6 decompositions species, adulterant compounds, and volatile organic compounds (VOCs).22–28 In these reports, the sensing properties depend on electronic interactions and adsorption strength between the target gaseous molecules and the sensor materials. The enormous adsorption energy will lead to stronger sensitivity. Thus, it is crucial to enhance the sensitivity by increasing the adsorption energy of the gas molecules on Janus 2D TMD materials. In the literature, some studies have used chemical doping, strain deformation, and external electric fields to adjust the sensitivity of gas molecules.29–32 Among these, strain deformation is commonly adopted, but external electric fields have been used in few studies for tuning the sensitivity of gas molecules on Janus 2D TMD monolayers.
It is well-known that the reactivity of catalytic reactions and the sensitivity of gas sensors can be modified by applying an external electric field.33–37 In the literature, methane conversion reactions have been reported to show better catalytic activity over various catalysts under external electric fields than without applying external electric fields.36,38,39 In addition, it has also been reported that 2D materials possess an excellent capability in capturing various gas molecules under external electric fields. For example, Sathishkumar et al. reported that the CO2 adsorption energy could be significantly enhanced by applying an external electric field on both N-doped penta-graphene and penta-C2N nanosheets.40,41 Moreover, Ma et al. calculated that the Sc2CO2 monolayer could regulate the adsorption strength of SO2 by applying tensile strains or controlling external electronic fields.42 Furthermore, for TMD materials, it has been reported that the external electric fields can significantly modulate charge transfer between the NO2/NH3 molecules and MoS2.43 However, the investigations on gas adsorption on Janus 2D TMD materials under external electronic fields are few, and the mechanism of external electronic fields towards gas adsorption on the Janus 2D TMD materials is also unknown. Thus, in this work, we investigated the adsorption and sensitivity of gas molecules on Janus 2D TMD materials under external electric fields.
Our previous studies have investigated that acetone possesses a good sensitivity over the Janus MoSSe monolayer.26 Besides, it has been reported that external electric fields show high reactivity to polar gas molecules during their adsorption. Since acetone is a well-known polar gas molecule, we will study the adsorption of acetone on both Janus MoSSe and pristine MoS2 monolayers under external electric fields. Moreover, we will also investigate the adsorption of another non-polar VOC, cyclohexane, on both Janus MoSSe and pristine MoS2 monolayers under external electric fields for comparison.
2. Computational details
2.1 DFT calculations
All calculations were carried out using the Vienna Ab initio Simulation Package (VASP)44–47 with the projector augmented wave (PAW) method.48,49 The exchange–correlation functional adopted was the Perdew–Burke–Ernzerhof (PBE)50 involved in the generalized gradient approximation (GGA) method. The Kohn–Sham orbitals were expanded in a plane-wave basis set with a kinetic energy cutoff of 520 eV. All calculations of 2D transition metal dichalcogenide monolayers were carried out using the 2H phase of pristine MoS2 and Janus MoSSe. The (4 × 4) supercell is considered for all TMDs monolayers with 15 Å vacuum along the c-axis to avoid the interlayer interaction, as shown in Fig. 1. As dipole interactions are necessary, the dipole corrections perpendicular to all monolayers were carried out in the calculations. The Brillion zone were sampled using (4 × 4 × 1) Gamma-centered Monkhorst–Pack k-points mesh51 for VOCs adsorption on different TMDs. The convergence threshold was 1 × 10−5 eV for the total electronic energy in the self-consistent loop. The atomic positions were relaxed using the quasi-Newton algorithm until the x, y, and z-components of unconstrained atomic force were smaller than 1 × 10−2 eV Å−1. The dispersion energy correction was considered using the DFT-D3 method by Grimme et al.52,53 The density-derived electrostatic and chemical (DDEC6) approach was examined for the electron transfer between the molecule and surface to analyze the electrical properties.54–56 Besides, the following equation defines the adsorption energies (Eads) of VOCs on the TMDs monolayers: |
Eads = EVOC/TMD − EVOC − ETMD
| (1) |
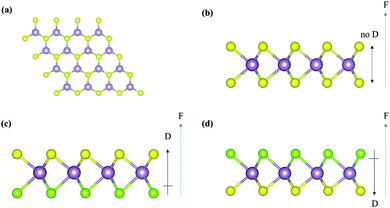 |
| Fig. 1 (a) Top view of the optimized TMDs monolayers with the 2H phase, (b) side view of the MoS2 monolayer under external electric field, (c) side view of the S-layer of MoSSe monolayer under external electric field, (d) side view of the Se-layer of MoSSe monolayer under external electric field. The D and F represent the intrinsic dipole moment of the monolayer as well as the external electric field, respectively, where the arrows indicate their directions. The purple, yellow, and green spheres represent Mo, S, and Se, respectively. | |
EVOC/TMD is the total energy of VOCs adsorbed on pristine MoS2 or Janus MoSSe. EVOC is the energy of free adsorbate VOC molecules, and ETMD is the energy of pristine MoS2 or Janus MoSSe monolayers.
2.2 External electric field calculations
The approach proposed by Neugebauer and Scheffler for adding the external electric field was used in this work. It generated an artificial dipole sheet at the middle of the vacuum space along the z-direction in the supercell to simulate a uniform electric field.57 Besides, a large enough vacuum space is needed to avoid the overlap between the charge density of the slab and the artificial dipole sheet.58 For an electron, it can tunnel to the vacuum when the distance between the surface and the artificial dipole sheet exceeds Φ/F (Φ and F are the work function and electric field, respectively). Since the calculated work functions of pristine MoS2 and Janus MoSSe monolayers were 5.45 eV and 5.54 eV, respectively, the maximum width should be ∼9 Å between the monolayers and the artificial dipole sheet under the maximum electric field (F = 0.6 V Å−1). Furthermore, it is roughly expressed as
for the charge density decaying, in which Φ is the work function (in eV), and z is the distance from the slab (in Å). Based on the calculated work function mentioned above, the charge density drops by order of magnitude per 0.90 Å. Thus, only negligible charge density resides around the dipole sheet introduced at ∼9 Å. Moreover, if the vacuum thickness is too wide, the external electric field can pull out the electrons from the Fermi level of the surface to the vacuum to cause the field emissions.58,59 In this work, because we set a ∼18 Å vacuum space, the width between the monolayers and the dipole sheet is ∼9 Å, which is enough to avoid the field emission issue. The above methods have been implemented in the VASP software, which can be realized by setting the keyword “EFIELD” in the INCAR during the calculation. Moreover, the dipole corrections to the slab and potential (IDIPOL and LDIPOL) should also be included in the calculation when applying external electric fields.
3. Results and discussion
3.1 Adsorption of acetone and cyclohexane on MoS2 and Janus MoSSe monolayers without external electric fields
Fig. 2 shows three different adsorption structures of acetone on Janus MoSSe and MoS2 monolayers without an external electric field. The adsorption structures of acetone can be divided into (1) acetone vertically adsorbing on the surface using its O end, named Acev1, (2) acetone vertically adsorbing on the surface using its C–H end, named Acev2, and (3) acetone adsorbing on the surface parallelly, named Aceh. The adsorption energies of Acev1, Acev2, and Aceh on pristine MoS2 monolayer are −0.24 eV, −0.29 eV, and −0.36 eV, respectively. In addition, since the Janus MoSSe monolayer possesses an asymmetric structure on both sides, the acetone molecule can adsorb on either the S-layer or Se-layer using the adsorption configuration mentioned above. The adsorption energies of Acev1, Acev2, and Aceh on the S-layer of the MoSSe monolayer are −0.22 eV, −0.29 eV, and −0.32 eV, respectively, while the adsorption energies of Acev1, Acev2, and Aceh on the Se-layer of the MoSSe monolayer are −0.23 eV, −0.25 eV, and −0.35 eV, respectively. These results reveal that the parallel adsorption of acetone molecule is the most favorable adsorption structure on the TMD monolayers. We also calculated the adsorption of a non-polar VOC molecule, cyclohexane, on both pristine MoS2 and Janus MoSSe monolayers in the absence of the external electric field. The most favorable adsorption structure of cyclohexane on the TMDs is also the parallel adsorption configuration using the van der Waals interaction, as shown in Fig. 3. The calculated adsorption energies of cyclohexane on pristine MoS2, S-layer of MoSSe, and Se-layer of MoSSe monolayers are −0.39 eV, −0.36 eV, and −0.37 eV, respectively. The adsorption energy of cyclohexane on the 2D TMDs is slightly larger than that of acetone (Table 1).
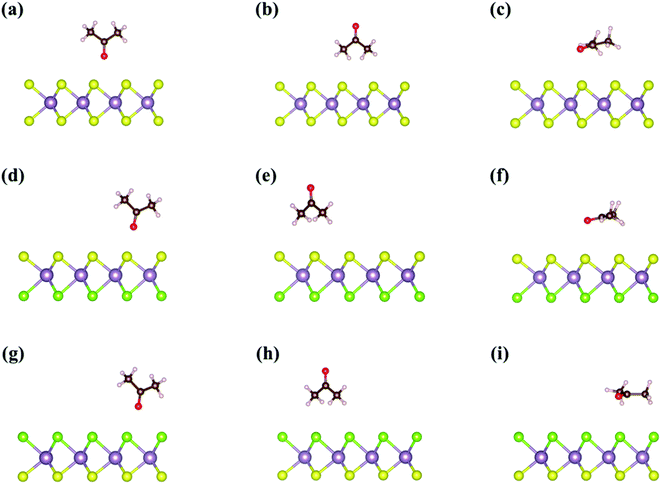 |
| Fig. 2 Optimized adsorption structures of acetone on the TMDs monolayers without the external electric fields: (a) Acev1, (b) Acev2, and (c) Aceh, on MoS2; (d) Acev1, (e) Acev2, and (f) Aceh, on S-layer of the Janus MoSSe; (g) Acev1, (h) Acev2 and (i) Aceh, on Se-layer of the Janus MoSSe. Purple, yellow, and green spheres represent Mo, S, and Se atoms, respectively, while brown, red, and light pink balls are C, O, and H atoms, respectively. | |
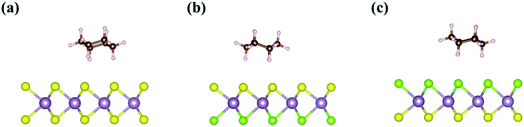 |
| Fig. 3 Optimized adsorption structures of cyclohexane on the (a) MoS2, (b) S-layer of the Janus MoSSe, and (c) Se-layer of the Janus MoSSe without the external electric fields. Purple, yellow, and green spheres represent Mo, S, and Se atoms, respectively, while brown, red, and light pink balls are C, O, and H atoms, respectively. | |
Table 1 The calculated adsorption energy, Eads for different adsorption structures of acetone and cyclohexane on pristine MoS2 and Janus MoSSe monolayers
TMD |
Species |
Adsorption type |
Eads (eV) |
MoS2 |
|
Acev1 |
−0.24 |
Acetone |
Acev2 |
−0.29 |
|
Aceh |
−0.36 |
Cyclohexane |
Parallel |
−0.39 |
MoSSe-S |
|
Acev1 |
−0.22 |
Acetone |
Acev2 |
−0.29 |
|
Aceh |
−0.32 |
Cyclohexane |
Parallel |
−0.36 |
MoSSe-Se |
|
Acev1 |
−0.23 |
Acetone |
Acev2 |
−0.25 |
|
Aceh |
−0.35 |
Cyclohexane |
Parallel |
−0.37 |
3.2 Adsorption of acetone on MoS2 monolayer with external electric fields
Subsequently, we investigated the adsorption of acetone on a pristine MoS2 monolayer in the presence of external electric fields, as shown in Fig. 4. The magnitude of external electric fields applied was between −0.6 V Å−1 to +0.6 V Å−1. As mentioned above, three possible adsorption structures are also listed out for acetone adsorption on pristine MoS2. As shown in Table 2, in the presence of the external electric field of +0.6 V Å−1, we found that the adsorption energy of Acev1, Acev2, and Aceh on the pristine MoS2 monolayer becomes 0.28 eV, −0.49 eV, and −0.17 eV, respectively. It shows that the adsorption energy of Acev2 increases while that of Acev1 and Aceh decreases in comparison with that in the absence of the external electric field. Besides, in the presence of external electric fields of +0.4 and +0.2 V Å−1, we observe similar trends, in which the adsorption energy of Acev2 still increases and those for Acev1 and Aceh decrease, as listed in Table 2. The result indicates that the adsorption energy of Acev2 is enhanced by the favorable external electric field, whereas that of Acev1 and Aceh are weakened, especially for Acev1, under the positive bias.
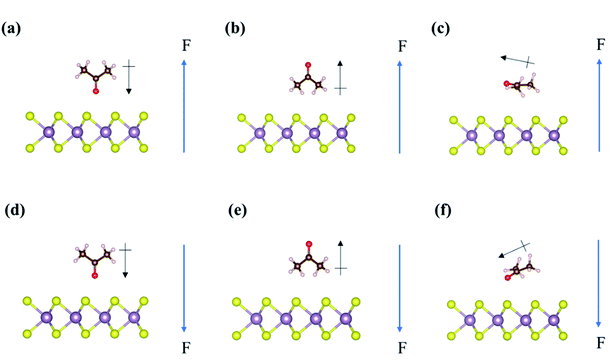 |
| Fig. 4 Optimized adsorption structures of acetone on MoS2 in the presence of the external electric field: (a) Acev1, (b) Acev2, and (c) Aceh under the positive external electric field; (d) Acev1, (e) Acev2, and (f) Aceh under the negative external electric field. The arrows are the directions of the dipole moment and the external electric field. Purple and yellow spheres represent Mo and S atoms, respectively, while brown, red, and light pink balls are C, O, and H atoms, respectively. | |
Table 2 Summary of electric field effects on the calculated adsorption energy, Eads, for different adsorption structures of acetone on pristine MoS2 and Janus MoSSe monolayers
E-field strength (V Å−1) |
MoS2 |
MoSSe-S |
MoSSe-Se |
Acev1 |
Acev2 |
Aceh |
Acev1 |
Acev2 |
Aceh |
Acev1 |
Acev2 |
Aceh |
0.6 |
0.28 |
−0.49 |
−0.17 |
−0.05 |
−0.85 |
−0.50 |
0.57 |
0.21 |
−0.11 |
0.4 |
0.02 |
−0.52 |
−0.31 |
−0.20 |
−0.77 |
−0.55 |
0.26 |
−0.10 |
−0.26 |
0.2 |
−0.11 |
−0.40 |
−0.34 |
−0.21 |
−0.53 |
−0.42 |
0.02 |
−0.20 |
−0.26 |
0 |
−0.24 |
−0.29 |
−0.36 |
−0.22 |
−0.29 |
−0.32 |
−0.23 |
−0.25 |
−0.35 |
−0.2 |
−0.39 |
−0.17 |
−0.38 |
−0.23 |
−0.05 |
−0.24 |
−0.48 |
−0.26 |
−0.50 |
−0.4 |
−0.52 |
−0.06 |
−0.44 |
−0.25 |
0.17 |
−0.19 |
−0.74 |
−0.27 |
−0.66 |
−0.6 |
−0.50 |
0.18 |
−0.36 |
−0.11 |
0.51 |
0.03 |
−0.84 |
−0.13 |
−0.65 |
On the other hand, when applying negative external electric fields of −0.60 V Å−1, −0.40 V Å−1, and −0.20 V Å−1, the adsorption energy of Acev1 on the pristine MoS2 monolayer becomes −0.50 eV, −0.52 eV, and −0.38 eV, respectively. However, the adsorption energy of Acev2 on pristine MoS2 monolayer becomes 0.18 eV, −0.06 eV, and −0.17 eV in the presence of external electric fields of −0.6 V Å−1, −0.4 V Å−1, and −0.2 V Å−1, respectively. Interestingly, the adsorption energy of Acev1 on pristine MoS2 monolayer is enhanced by the negative external electric field, which shows the opposite trend to the results of applying positive external electric fields.
3.3 Adsorption of acetone on Janus MoSSe monolayer with external electric fields
For the adsorption of acetone on the Janus MoSSe monolayer in the presence of external electric fields, we found that the S-layer and Se-layer of Janus MoSSe show extreme differences. Fig. 5 displays all the adsorption structures of acetone on the Janus MoSSe monolayer under positive or negative external electric fields. First, on the S-layer of Janus MoSSe monolayer (Fig. 5(a)–(f)), the adsorption energy of Acev1 is −0.21 eV, −0.20 eV, and −0.05 eV under +0.2 V Å−1 to +0.6 V Å−1, while it is −0.23 eV, −0.25 eV, and −0.11 eV under −0.2 V Å−1 to −0.6 V Å−1, respectively. This result reveals that the adsorption energy of Acev1 almost remains at around −0.23 eV and then decreases after the external electric fields increases to +0.6 V Å−1 or −0.6 V Å−1. On the contrary, the adsorption energy of Acev2 on the S-layer of Janus MoSSe monolayer increases to −0.53 eV, −0.77 eV, and −0.85 eV under the external electric fields of +0.2 V Å−1, +0.4 V Å−1, and +0.6 V Å−1, respectively. However, the adsorption energy of Acev2 dramatically decreases to −0.05, 0.17, and 0.51 eV from −0.2 V Å−1 to −0.6 V Å−1, respectively. Besides, the adsorption energy of Aceh possesses similar changing trends to Acev2 in that the positive bias can enhance its adsorption energy. However, the negative bias will inhibit the adsorption energy of Aceh on the S-layer of the Janus MoSSe monolayer.
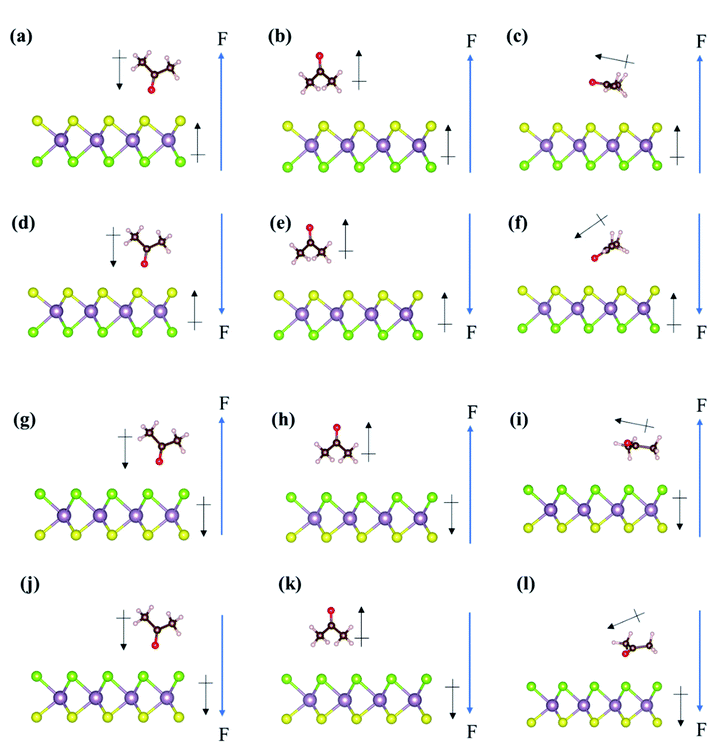 |
| Fig. 5 (a) Acev1, (b) Acev2, and (c) Aceh under a positive external electric field; (d) Acev1, (e) Acev2, and (f) Aceh under a negative external electric field, in the optimized adsorption structures of acetone on the S-layer of Janus MoSSe. (g) Acev1, (h) Acev2, and (i) Aceh under the positive external electric field; (j) Acev1, (k) Acev2, and (l) Aceh under the negative external electric field, in the optimized adsorption structures of acetone on the Se-layer of Janus MoSSe. The arrows are the directions of the dipole moment and the external electric field. Purple, yellow, and green spheres represent Mo, S, and Se atoms, respectively, while brown, red, and light pink balls are C, O, and H atoms, respectively. | |
Moreover, the adsorption structures of Aceh will be orientated by the positive bias and then tilt around 30° (Fig. 5(c)). In comparison, the negative bias can result in a tilting angle of about −30° in the adsorption structure of Aceh on the S-layer of Janus MoSSe monolayer (Fig. 5(f)). Our results demonstrate that either positive or negative external electric fields show immense magnitude change to Acev2 and Aceh on the S-layer of Janus MoSSe monolayer, particularly for Acev2. However, the effect of external electric fields on Acev1 is limited. Hence, the adsorption energy of acetone in the presence of the external electric field on the S-layer of the Janus MoSSe monolayer displays a different trend to that on the pristine MoS2 monolayer.
On the Se-layer of the Janus MoSSe monolayer, the adsorption energy of acetone shows an opposite trend to the counterpart of acetone on the Janus MoSSe monolayer's S-layer in Fig. 5(g)–(l). The adsorption energy of Acev1 on the Se-layer of the Janus MoSSe monolayer dramatically changes in a range from 0.57 eV to −0.84 eV under +0.6 V Å−1 to −0.6V Å−1, which is similar to the changing magnitude of Acev2 on the S-layer of the Janus MoSSe monolayer. Likewise, as listed in Table 2, the changing magnitude of the adsorption energy for Acev2 on the Se-layer of the Janus MoSSe monolayer is like the trend of Acev1 on the S-layer of the Janus MoSSe monolayer. Furthermore, the positive external electric field shows an inhibition to the adsorption energy for Aceh. In contrast, the negative external electric field demonstrates the enhancement of the adsorption energy of Aceh on the Se-layer of the Janus MoSSe monolayer. This result is opposite to the adsorption of Aceh on the S-layer of the Janus MoSSe monolayer. Consequently, we found that external electric fields substantially affect the adsorption energy of Acev1 and Aceh on the Se-layer of Janus MoSSe monolayer, particularly for Acev1. In summary, the result indicates that the Janus MoSSe monolayer shows highly selective sensitivity concerning the acetone molecule in the presence of external electric fields.
3.4 Adsorption of cyclohexane on Janus MoSSe and MoS2 monolayers with external electric fields
The adsorption of cyclohexane on the Janus MoSSe monolayer represents the interaction between a non-polar molecule and a polar material, as shown in Fig. 6. In the presence of external electric fields, the adsorption energy of cyclohexane on the S-layer of the Janus MoSSe monolayer becomes −0.72 eV, −0.60 eV, −0.48 eV, −0.24 eV, −0.12 eV, and −0.01 eV, under the external electric field values from +0.6 V Å−1 to −0.6 V Å−1, as listed in Table 3. In addition, the adsorption energy of cyclohexane on Se-layer of the Janus MoSSe monolayer illustrates an inverse result. The adsorption energy varies from −0.01 eV to −0.72 eV when the external electric field is from +0.6 V Å−1 to −0.6 V Å−1. Furthermore, the adsorption energy of cyclohexane on pristine MoS2 monolayer almost remains constant at around −0.39 eV under either positive or negative external electric fields. Compared with cyclohexane adsorption on pristine MoS2 monolayer, the Janus MoSSe monolayer shows better sensitivity than the MoS2 monolayer under external electric fields (MoS2 monolayer shows no change in the adsorption energy under external electric fields). Moreover, we observe that the changing trends in the adsorption energies of cyclohexane are the same at both sides of the Janus MoSSe monolayer, and the only difference is the direction of external electric fields.
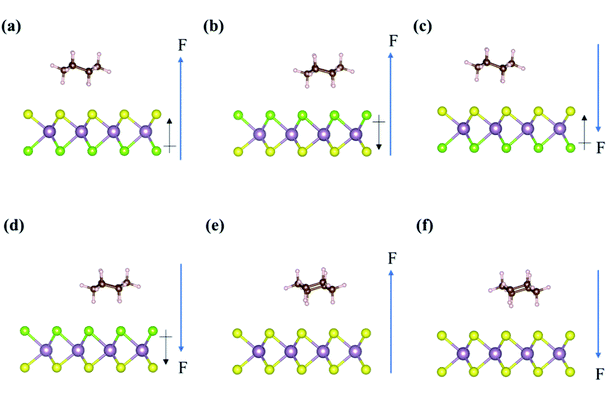 |
| Fig. 6 Optimized adsorption structures of cyclohexane on the (a) S-layer of Janus MoSSe with the positive external electric field, (b) Se-layer of Janus MoSSe with the positive external electric field, (c) S-layer of Janus MoSSe with the negative external electric field, (d) Se-layer of Janus MoSSe with the negative external electric field, (e) MoS2 with the positive external electric field, and (f) MoS2 with a negative external electric field. The arrows are the directions of the dipole moment and the external electric field. Purple, yellow, and green spheres represent Mo, S, and Se atoms, respectively, while brown, red, and light pink balls are C, O, and H atoms, respectively. | |
Table 3 Summary of electric field effects on the calculated adsorption energy, Eads, for different adsorption structures of cyclohexane on pristine MoS2 and Janus MoSSe monolayers
E-field strength (V Å−1) |
Cyclohexane |
MoS2 |
MoSSe-S |
MoSSe-Se |
0.6 |
−0.38 |
−0.72 |
0.00 |
0.4 |
−0.39 |
−0.60 |
−0.10 |
0.2 |
−0.39 |
−0.48 |
−0.22 |
0 |
−0.39 |
−0.36 |
−0.37 |
−0.2 |
−0.39 |
−0.24 |
−0.46 |
−0.4 |
−0.40 |
−0.12 |
−0.58 |
−0.6 |
−0.40 |
−0.01 |
−0.72 |
3.5 Dipole moment analysis
Our results find that both acetone and cyclohexane show good sensitivity on the Janus MoSSe monolayer in the presence of external electric fields. However, only the acetone molecule is affected by external electric fields on the pristine MoS2 monolayer. Moreover, the changing magnitude of the adsorption energy of acetone on the Janus MoSSe monolayer is much stronger than that on the MoS2 monolayer. As mentioned in the previous studies,34,36 the external electric fields can couple with the internal electric fields between the gas molecule and surface and the intrinsic dipole moments of the gas molecule. In this study, since both acetone and Janus MoSSe monolayer possess intrinsic dipole moments, the external electric fields could interact with both of them. Hence, we observed that the directions of intrinsic dipole moments of acetone and MoSSe monolayer strongly correlate with the directions of external electric fields. In VASP software, the directions of positive and negative external electric fields were set as upward (↑) and downward (↓), respectively. Therefore, when the directions of the external electric field and dipole moments are in the same direction, the adsorption energy of acetone has the most prominent enhancement. This situation occurs in the adsorption of Acev2 on the S-layer (see the arrows in Fig. 5(b)) and Acev1 on the Se-layer (see the arrows in Fig. 5(j)) of the MoSSe monolayer under positive and negative external electric fields, respectively. Second, when one of the dipole moments in either acetone or Janus MoSSe is opposite to the external electric fields, the adsorption energy of acetone will decrease, as shown by the arrows in Fig. 5(a), (d), (h), and (k).
Furthermore, when the direction of the external electric field is opposite to both acetone and MoSSe monolayer, the adsorption energy of acetone will dramatically and remarkably decrease, even the positive adsorption energy value, for instance, the Acev2 on the S-layer of the Janus MoSSe monolayer under negative external electric field (Fig. 5(e)), or the Acev1 on the Se-layer of the Janus MoSSe monolayer under positive external electric field (Fig. 5(g)). All the detailed orientations of adsorbates, surfaces, and external electric fields are recorded in Tables S1 and S2.†
Similarly, in the adsorption of cyclohexane on the Janus MoSSe monolayer under an external electric field, the external electric field can only react with the intrinsic dipole moment of the MoSSe monolayer since cyclohexane has no dipole moment. Hence, the change in the adsorption energy of cyclohexane on the Janus MoSSe monolayer is only altered by the direction between the intrinsic dipole moment of the MoSSe monolayer and the external electric field. The positive external electric field can enhance the adsorption energy of cyclohexane on the S-layer of the MoSSe monolayer. In contrast, the negative external electric field can enhance the adsorption energy of cyclohexane on the Se-layer of MoSSe monolayer and vice versa, as listed in Table S3.†
A similar phenomenon is also observed in the adsorption of acetone and cyclohexane on the MoS2 monolayer, as shown by the arrows in Fig. 4 and 6. For the adsorption of acetone on MoS2, since only acetone has the intrinsic dipole moment, it can enhance the adsorption energy of acetone when the direction of its intrinsic dipole moment and the external electric field are the same and vice versa, as listed in Tables S1 and S2.† For the adsorption of cyclohexane on the MoS2 monolayer, because both cyclohexane and MoS2 monolayer have no intrinsic dipole moment, the adsorption energy can not be altered by the external electric field, as shown in Table S3.†
3.6 Sensing of NO molecule
In the literature, it has been reported that both MoSSe and MoS2 monolayers possess good sensitivity towards smaller and simpler toxic species, such as NO molecules.22,43,60 Hence, we have calculated the NO sensing properties on both MoSSe and MoS2 monolayers in the presence of external electric fields. NO molecule possesses a small dipole moment value of 0.16 D, while the dipole moment value of the MoSSe monolayer is 2.99 D. Thus, the external electric field can majorly react with the intrinsic dipole moment of the MoSSe monolayer when NO adsorbs on the MoSSe monolayer under the external electric field. The adsorption energies of NO on the MoSSe monolayer majorly depend on the orientations between the MoSSe monolayer and the external electric field. When the orientations between the MoSSe monolayer and the external electric field are the same, the adsorption energies of NO on the MoSSe monolayer can be enhanced and vice versa, as shown in Fig. S1 and Table S4.† Moreover, the adsorption energies of NO on the MoS2 monolayer change only slightly with and without the external electric fields. It results from the small dipole moment value of the NO molecule since the MoS2 monolayer is a non-polar material. However, although the MoSSe monolayer also has better sensitivity towards NO sensing under external electric fields, the adsorption energies of the NO molecule are still smaller than that of acetone and cyclohexane on the MoSSe monolayer.
3.7 Electronic property analysis
As mentioned earlier, the adsorption structures of both Acev1 and Acev2 can be strongly regulated by different orientations of external electric fields on the Janus MoSSe monolayer. The adsorption energy of Acev1 on the Se-layer of MoSSe can be enhanced by the negative bias, while that of Acev2 on the S-layer of MoSSe can be enhanced by the positive bias. Therefore, we calculate the net atomic charges by DDEC6 of acetone on the Janus MoSSe monolayer to further understand the interaction between the external electric fields and the electron transfer of the gas/surface, as listed in Table 4. We considered the DDEC6 charges for Acev1 on the Se-layer of MoSSe and Acev2 on the S-layer of MoSSe. Without the external electric field, the calculated DDEC6 charge of Acev1 on the Se-layer of MoSSe is 0.074|e|, indicating that the electron transfer is from acetone to the Se-layer MoSSe. This electron transfer also represents that the direction of the internal electric field between Acev1 and Se-layer of MoSSe is downward. When applying the negative external electric field in the same direction, the internal electric field between Acev1 and the Se-layer of MoSSe can be enhanced by the external electric field. Hence, the electron transfer between Acev1 on the Se-layer of MoSSe becomes 0.090|e|, 0.105|e|, and 0.122|e| under the external electric fields of −0.2 V Å−1, −0.4 V Å−1, and −0.6 V Å−1, respectively. This result reveals that Acev1 can donate more electrons to the Se-layer of MoSSe via enhancing the negative external electric field.
Table 4 Calculated DDEC atomic charges for the selected adsorption structures of acetone molecule after adsorption on Janus MoSSe monolayer. The positive and negative values indicate losing and gaining electrons of the acetone molecule
E-field strength (V Å−1) |
TMD |
Adsorption type |
DDEC (|e|) |
0.6 |
MoSSe-S |
Acev2 |
−0.007 |
0.4 |
MoSSe-S |
Acev2 |
0.008 |
0.2 |
MoSSe-S |
Acev2 |
0.017 |
0 |
MoSSe-S |
Acev2 |
0.033 |
0 |
MoSSe-Se |
Acev1 |
0.074 |
−0.2 |
MoSSe-Se |
Acev1 |
0.090 |
−0.4 |
MoSSe-Se |
Acev1 |
0.105 |
−0.6 |
MoSSe-Se |
Acev1 |
0.122 |
On the other hand, for the adsorption of Acev2 and the S-layer of MoSSe, the calculated DDEC6 charge of Acev2 is 0.033|e| in the absence of the external electric field. It also indicates that the electron transfer is from acetone to the S-layer of MoSSe. However, a positive external electric field can induce the electron transfer from the S-layer of MoSSe to acetone. Thus, as shown in Table 4, the calculated DDEC6 charges of Acev2 become 0.017|e|, 0.008|e|, and −0.007|e| under the external electric fields of +0.2 V Å−1, +0.4 V Å−1, and +0.6 V Å−1, respectively. We found that the electron transfer between the S-layer of MoSSe and acetone changes from positive net charges to negative net charges, indicating that acetone transforms from being an electron donor to an electron acceptor with increasing external electric fields. These results demonstrate that the internal electric field between acetone and the MoSSe monolayer will also be strongly affected by external electric fields.
Besides, in our results, the DDEC6 charges of S and Se in MoS2 and Janus MoSSe monolayers have different distributions under different external electric fields. For instance, the DDEC6 charges of S and Se in Janus MoSSe monolayer without electric field are −0.239|e| and −0.180|e|, respectively. Under the electric field of +0.6 V Å−1, the DDEC6 charges of S and Se in Janus MoSSe monolayer become −0.274|e| and −0.141|e|, respectively. One can see that the net charge difference between S and Se increased from 0.059|e| to 0.133|e|. This result indicates that the intrinsic dipole moment of Janus MoSSe can be enlarged by applying the external electric field.
3.8 Effective dipole moment and effective polarizability analysis
The relationship between the adsorption energy and dipole moment as well as polarizability in the presence of a uniform electric field could be summarized by a Taylor series as follows,59,61–63
Eads and E0ads represent the approximated adsorption energies in the presence and the absence of an electric field, respectively. F is the magnitude of the external electric field. ΔμF=0 and ΔαF=0 are the effective dipole moment difference and effective polarizability difference between the adsorbates and surfaces at the zero-electric field, respectively. Fig. 7 shows the plots of adsorption energies for acetone and cyclohexane on both pristine MoS2 and Janus MoSSe monolayers as a function of external electric fields. Table 5 lists the fitting results from Fig. 7, which includes the effective dipole moment and effective polarizability for the adsorption of gas molecules on both pristine MoS2 and Janus MoSSe monolayers.
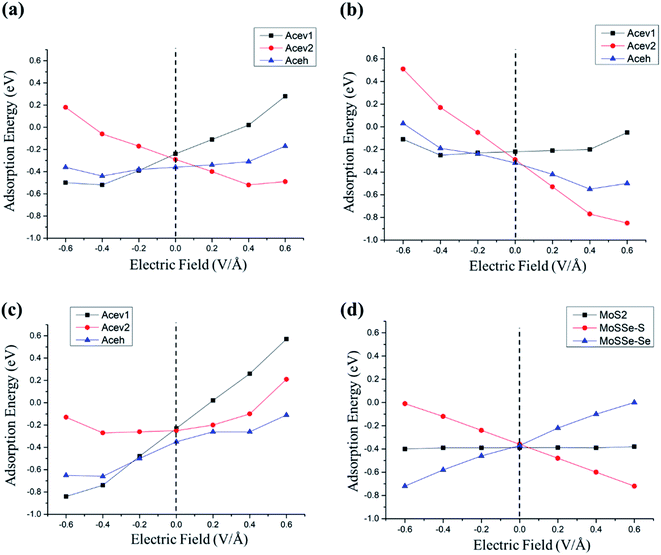 |
| Fig. 7 Effects of an applied external electric field for (a) acetone on MoS2, (b) acetone on the S-layer of Janus MoSSe, (c) acetone on the Se-layer of Janus MoSSe, and (d) cyclohexane on both MoS2 and Janus MoSSe monolayers. | |
Table 5 Fitting results of variations in the adsorption energies as a function of the electric field (V Å−1) for acetone and cyclohexane adsorption on pristine MoS2 and Janus MoSSe monolayers. ΔμF=0 and ΔαF=0 are the corresponding calculated electric dipole moment (in e Å) and electric polarizability (in e Å2 V−1), respectively
TMD |
Species |
Adsorption type |
Fitting results |
ΔμF=0 |
ΔαF=0 |
MoS2 |
|
Acev1 |
Eads = −0.27 + 0.66F + 0.40F2 |
−0.66 |
−0.80 |
Acetone |
Acev2 |
Eads = −0.31 − 0.56F + 0.39F2 |
0.56 |
−0.78 |
|
Aceh |
Eads = −0.38 + 0.15F + 0.28F2 |
−0.15 |
−0.56 |
Cyclohexane |
Parallel |
Eads = −0.39 + 0.01F − 0.002F2 |
−0.01 |
0.004 |
MoSSe-S |
|
Acev1 |
Eads = −0.25 + 0.05F + 0.42F2 |
−0.05 |
−0.84 |
Acetone |
Acev2 |
Eads = −0.32 − 1.15F + 0.36F2 |
1.15 |
−0.72 |
|
Aceh |
Eads = −0.36 − 0.44F + 0.27F2 |
0.44 |
−0.54 |
Cyclohexane |
Parallel |
Eads = −0.36 − 0.59F − 0.02F2 |
0.59 |
0.04 |
MoSSe-Se |
|
Acev1 |
Eads = −0.25 + 1.20F + 0.28F2 |
−1.20 |
−0.56 |
Acetone |
Acev2 |
Eads = −0.28 + 0.25F + 0.83F2 |
−0.25 |
−1.66 |
|
Aceh |
Eads = −0.39 + 0.48F − 0.04F2 |
−0.48 |
0.08 |
Cyclohexane |
Parallel |
Eads = −0.35 + 0.60F − 0.02F2 |
−0.60 |
0.04 |
We found that the effective polarizability of acetone on pristine MoS2 varies from −0.56 to −0.80. In comparison, the effective polarizability of acetone on both sides of MoSSe displays a wide range from 0.08 to −1.66. This result implies that the effective polarizability change is more sensitive to acetone on MoSSe than on the MoS2 monolayer. In addition, the absolute maximum effective dipole moment of acetone on pristine MoS2 is 0.66. In contrast, the corresponding value for acetone on Janus MoSSe is 1.20, almost two times that of acetone on MoS2. Thus, the adsorption energy of a polar molecule on a polar material is more sensitive than that of a polar molecule on a non-polar material in the presence of an external electric field due to effective dipole moment and effective polarizability.
On the other hand, the effective polarizability of cyclohexane on both pristine MoS2 and Janus MoSSe monolayer is negligible, which is in a range from 0.004 to 0.08. Moreover, the effective dipole moment of cyclohexane on pristine MoS2 is also negligible, which is −0.01. However, the effective dipole moment of cyclohexane on Janus MoSSe monolayer possesses a value of −0.60. These results indicate that the external electric field can affect the effective dipole moment between cyclohexane and the Janus MoSSe monolayer. However, it does not affect the effective polarizability between cyclohexane and the Janus MoSSe monolayer. As a result, it is demonstrated that the adsorption energy of a non-polar molecule on a polar material could be strongly altered by an external electric field than that of a non-polar molecule on a non-polar material based on effective dipole moments.
3.9 Desorption temperatures analysis
To understand the desorption temperatures towards re-use of the sensor, we performed micro-kinetics simulations and calculated the desorption temperatures of adsorbates under different external electric fields. The MKMCXX software was used to calculate the microkinetic simulations.64 Fig. S2(a)† illustrates the temperature evolution of acetone coverage on Janus MoSSe monolayer. Without applying the external electric fields, the desorption temperature of acetone on the MoSSe monolayer is from 150 K to 220 K. When increasing the magnitudes of the external electric fields, the desorption temperatures of acetone on the MoSSe monolayer also increase due to the enhancement of its adsorption energy. At 0.6 V Å−1, the desorption temperature of acetone on the MoSSe monolayer is from 380 K to 500 K. Fig. S2(b) to (d)† show the calculated temperature-programmed desorption diagrams of acetone on MoS2, cyclohexane on MoSSe, and cyclohexane on MoS2 under different external electric fields, respectively. The results reveal that the desorption temperatures of acetone and cyclohexane on the MoSSe monolayer are located at a specific temperature under specific external electric fields, including high and low temperatures. On the other hand, the desorption temperatures of acetone and cyclohexane on the MoS2 monolayer are all concentrated in the range of around 200 to 350 K. Thus, the calculated desorption temperatures demonstrate that the MoSSe monolayer can detect gas molecules at different specific external electric fields. It also reflects that the MoSSe monolayer possesses more sensitivity towards the detection of gas molecules under external electric fields. Besides, the mechanism for the gas molecule's desorption depends on the magnitudes of external electric fields as well as the desorption temperatures. A larger external electric field can lead to a higher desorption temperature and vice versa. Thus, tuning the external electric field can control the sensitivity of the sensor, and it can enable the re-use of the sensor.
4. Conclusions
We have employed DFT calculations to study the adsorption of acetone and cyclohexane on pristine MoS2 and Janus MoSSe monolayers in the presence of external electric fields in this work. According to our results, cyclohexane shows slightly larger adsorption energy on both pristine MoS2 and Janus MoSSe monolayers than that of acetone in the absence of external electric fields. In addition, under external electric fields, we found that the adsorption energy of acetone can be enhanced from −0.31 eV to −0.85 eV while that of cyclohexane can be enhanced from −0.36 eV to −0.72 eV on Janus MoSSe monolayer. Moreover, the adsorption energy of acetone on pristine MoS2 monolayer also shows an enhancement from −0.35 eV to −0.57 eV in the presence of external electric fields. However, we observed that the adsorption energy of cyclohexane has almost no change on pristine MoS2 monolayer in the presence of external electric fields.
To elucidate, we analyzed the dipole moments and the electron transfer between the adsorbates and the monolayers. Because the Janus MoSSe and acetone are polar species while pristine MoS2 and cyclohexane are non-polar species, the intrinsic dipole moments of Janus MoSSe and acetone can react with the external electric fields. We found that when the orientations of dipole moments of Janus MoSSe, acetone, and external electric fields are all the same, this situation will maximize the adsorption energy of the acetone molecule. Besides, when there is only one polar species in the system, i.e., acetone on MoS2 or cyclohexane on Janus MoSSe, the enhancement of the adsorption energy for these gas molecules is smaller than the counterpart of acetone on Janus MoSSe. Finally, since cyclohexane and MoS2 are without intrinsic dipole moments, the adsorption of cyclohexane on MoS2 is not affected by the external electric fields. The calculated DDEC atomic charges also reveal that the internal electric field between acetone and Janus MoSSe will be enhanced or inhibited by external electric fields in different directions. By calculating the effective dipole moment and effective polarizability, we observe that the effective dipole moment plays an important role in the adsorption of either acetone or cyclohexane on Janus MoSSe monolayer under external electric fields. Consequently, our results demonstrate that polar materials possess better electric sensitivity than non-polar materials in the presence of external electric fields. This finding can be applied in the design of gas sensors and can be used in gas storage applications in the future through polar materials.
Conflicts of interest
There are no conflicts to declare.
Acknowledgements
The authors gratefully acknowledge the financial support for this work from the grant 109-2113-M-035-003-MY3 of the Ministry of Science and Technology (MOST), Taiwan. The National Center of High-Performance Computing (NCHC) contributed to this project by allowing access to their computer facilities and donating computer time.
References
- K. S. Novoselov, A. K. Geim, S. V. Morozov, D. Jiang, Y. Zhang, S. V. Dubonos, I. V. Grigorieva and A. A. Firsov, Science, 2004, 306, 666–669 CrossRef CAS PubMed.
- Z. Chen, J. Wang, D. Pan, Y. Wang, R. Noetzel, H. Li, P. Xie, W. Pei, A. Umar, L. Jiang, N. Li, N. F. Rooij and G. Zhou, ACS Nano, 2018, 12, 2521–2530 CrossRef CAS PubMed.
- G. Fiori, F. Bonaccorso, G. Iannaccone, T. Palacios, D. Neumaier, A. Seabaugh, S. K. Banerjee and L. Colombo, Nat. Nanotechnol., 2014, 9, 768–779 CrossRef CAS PubMed.
- Y. H. Kim, S. J. Kim, Y. J. Kim, Y. S. Shim, S. Y. Kim, B. H. Hong and H. W. Jang, ACS Nano, 2015, 9, 10453–10460 CrossRef CAS PubMed.
- Y. H. Kim, J. S. Park, Y.-R. Choi, S. Y. Park, S. Y. Lee, W. Sohn, Y.-S. Shim, J.-H. Lee, C. R. Park, Y. S. Choi, B. H. Hong, J. H. Lee, W. H. Lee, D. Lee and H. W. Jang, J. Mater. Chem. A, 2017, 5, 19116–19125 RSC.
- R. Mas-Balleste, C. Gomez-Navarro, J. Gomez-Herrero and F. Zamora, Nanoscale, 2011, 3, 20–30 RSC.
- K. S. Novoselov, A. Mishchenko, A. Carvalho and A. H. Castro Neto, Science, 2016, 353, aac9439 CrossRef CAS PubMed.
- Z. Sun, T. Liao, Y. Dou, S. M. Hwang, M. S. Park, L. Jiang, J. H. Kim and S. X. Dou, Nat. Commun., 2014, 5, 3813 CrossRef CAS PubMed.
- F. Torrisi and J. N. Coleman, Nat. Nanotechnol., 2014, 9, 738–739 CrossRef CAS PubMed.
- J. K. Huang, J. Pu, C. L. Hsu, M. H. Chiu, Z. Y. Juang, Y. H. Chang, W. H. Chang, Y. Iwasa, T. Takenobu and L. J. Li, ACS Nano, 2014, 8, 923–930 CrossRef CAS PubMed.
- S. Manzeli, D. Ovchinnikov, D. Pasquier, O. V. Yazyev and A. Kis, Nat. Rev. Mater., 2017, 2, 17033 CrossRef CAS.
- C. Tan, Z. Lai and H. Zhang, Adv. Mater., 2017, 29, 1701392 CrossRef PubMed.
- J. Wang, Y. Wei, H. Li, X. Huang and H. Zhang, Sci. China Chem., 2018, 61, 1227–1242 CrossRef CAS.
- X. Zhang, Z. Lai, Q. Ma and H. Zhang, Chem. Soc. Rev., 2018, 47, 3301–3338 RSC.
- A. Y. Lu, H. Zhu, J. Xiao, C. P. Chuu, Y. Han, M. H. Chiu, C. C. Cheng, C. W. Yang, K. H. Wei, Y. Yang, Y. Wang, D. Sokaras, D. Nordlund, P. Yang, D. A. Muller, M. Y. Chou, X. Zhang and L. J. Li, Nat. Nanotechnol., 2017, 12, 744–749 CrossRef CAS PubMed.
- J. Zhang, S. Jia, I. Kholmanov, L. Dong, D. Er, W. Chen, H. Guo, Z. Jin, V. B. Shenoy, L. Shi and J. Lou, ACS Nano, 2017, 11, 8192–8198 CrossRef CAS PubMed.
- R. McWeeny, Rev. Mod. Phys., 1960, 32, 335–369 CrossRef.
- W. Shi, Z. Wang and Y. Q. Fu, J. Phys. D: Appl. Phys., 2017, 50, 405303 CrossRef.
- B. Radisavljevic, A. Radenovic, J. Brivio, V. Giacometti and A. Kis, Nat. Nanotechnol., 2011, 6, 147–150 CrossRef CAS PubMed.
- E. Gourmelon, O. Lignier, H. Hadouda, G. Couturier, J. C. Bernède, J. Tedd, J. Pouzet and J. Salardenne, Sol. Energy Mater. Sol. Cells, 1997, 46, 115–121 CrossRef CAS.
- A. Shokri and N. Salami, Sensor. Actuator. B Chem., 2016, 236, 378–385 CrossRef CAS.
- R. Chaurasiya and A. Dixit, Appl. Surf. Sci., 2019, 490, 204–219 CrossRef CAS.
- R. Chaurasiya and A. Dixit, Phys. Chem. Chem. Phys., 2020, 22, 13903–13922 RSC.
- C. Jin, X. Tang, X. Tan, S. C. Smith, Y. Dai and L. Kou, J. Mater. Chem. A, 2019, 7, 1099–1106 RSC.
- X.-Y. Yang, T. Hussain, J. P. A. Wärnå, Z. Xu and R. Ahuja, Comput. Mater. Sci., 2021, 186 Search PubMed.
- C. H. Yeh, ACS Omega, 2020, 5, 31398–31406 CrossRef CAS PubMed.
- B. Zhu, K. Zheng, X. Chen, J. Qiu, H. Guo, F. Zhang, L. Lang, J. Yu and J. Bao, Phys. Chem. Chem. Phys., 2021, 23, 1675–1683 RSC.
- P. Panigrahi, D. Jini, H. Bae, H. Lee, R. Ahuja and T. Hussain, Appl. Surf. Sci., 2021, 542 Search PubMed.
- D. Chen, X. Lei, Y. Wang, S. Zhong, G. Liu, B. Xu and C. Ouyang, Appl. Surf. Sci., 2019, 497 Search PubMed.
- F. Li, W. Wei, H. Wang, B. Huang, Y. Dai and T. Jacob, J. Phys. Chem. Lett., 2019, 10, 559–565 CrossRef PubMed.
- J. Zeng, G. Liu, Y. Han, W. Luo, M. Wu, B. Xu and C. Ouyang, ACS Omega, 2021, 6, 14639–14647 CrossRef CAS PubMed.
- R. Zhao, T. Wang, M. Zhao, C. Xia, Y. An, S. Wei and X. Dai, Appl. Surf. Sci., 2019, 491, 128–137 CrossRef CAS.
- A. C. Aragones, N. L. Haworth, N. Darwish, S. Ciampi, N. J. Bloomfield, G. G. Wallace, I. Diez-Perez and M. L. Coote, Nature, 2016, 531, 88–91 CrossRef CAS PubMed.
- K.-Y. Lin, S. Nachimuthu, M. T. Nguyen, H. Mizuta and J.-C. Jiang, J. Phys. Chem. C, 2019, 123, 30373–30381 CrossRef CAS.
- S. Nachimuthu, L. He, H.-J. Cheng, R. D. Tiono and J.-C. Jiang, Sustain. Energy Fuels, 2021, 5, 2159–2168 RSC.
- C.-H. Yeh, T. M. L. Pham, S. Nachimuthu and J.-C. Jiang, ACS Catal., 2019, 9, 8230–8242 CrossRef CAS.
- L. Yue, N. Wang, S. Zhou, X. Sun, M. Schlangen and H. Schwarz, Angew. Chem., Int. Ed., 2018, 57, 14635–14639 CrossRef CAS PubMed.
- F. Che, J. T. Gray, S. Ha, N. Kruse, S. L. Scott and J.-S. McEwen, ACS Catal., 2018, 8, 5153–5174 CrossRef CAS.
- T. Yabe, K. Yamada, T. Oguri, T. Higo, S. Ogo and Y. Sekine, ACS Catal., 2018, 8, 11470–11477 CrossRef CAS.
- N. Sathishkumar, S.-Y. Wu and H.-T. Chen, Chem. Eng. J., 2020, 391 Search PubMed.
- N. Sathishkumar, S.-Y. Wu and H.-T. Chen, Chem. Eng. J., 2021, 407 Search PubMed.
- S. Ma, D. Yuan, Z. Jiao, T. Wang and X. Dai, J. Phys. Chem. C, 2017, 121, 24077–24084 CrossRef CAS.
- Q. Yue, Z. Shao, S. Chang and J. Li, Nanoscale Res. Lett., 2013, 8, 425 CrossRef PubMed.
- G. Kresse and J. Hafner, Phys. Rev. B: Condens. Matter Mater. Phys., 1993, 47, 558–561 CrossRef CAS PubMed.
- G. Kresse and J. Hafner, Phys. Rev. B: Condens. Matter Mater. Phys., 1994, 49, 14251–14269 CrossRef CAS PubMed.
- G. Kresse and J. Furthmuller, Phys. Rev. B: Condens. Matter Mater. Phys., 1996, 54, 11169–11186 CrossRef CAS PubMed.
- G. Kresse and J. Furthmüller, Comput. Mater. Sci., 1996, 6, 15–50 CrossRef CAS.
- P. E. Blochl, Phys. Rev. B: Condens. Matter Mater. Phys., 1994, 50, 17953–17979 CrossRef PubMed.
- G. Kresse and D. Joubert, Phys. Rev. B: Condens. Matter Mater. Phys., 1999, 59, 1758–1775 CrossRef CAS.
- J. P. Perdew, K. Burke and M. Ernzerhof, Phys. Rev. Lett., 1996, 77, 3865–3868 CrossRef CAS PubMed.
- H. J. Monkhorst and J. D. Pack, Phys. Rev. B: Solid State, 1976, 13, 5188–5192 CrossRef.
- S. Grimme, J. Antony, S. Ehrlich and H. Krieg, J. Chem. Phys., 2010, 132, 154104 CrossRef PubMed.
- S. Grimme, S. Ehrlich and L. Goerigk, J. Comput. Chem., 2011, 32, 1456–1465 CrossRef CAS PubMed.
- N. G. Limas and T. A. Manz, RSC Adv., 2016, 6, 45727–45747 RSC.
- T. A. Manz, RSC Adv., 2017, 7, 45552–45581 RSC.
- T. A. Manz and N. G. Limas, RSC Adv., 2016, 6, 47771–47801 RSC.
- J. Neugebauer and M. Scheffler, Phys. Rev. B: Condens. Matter Mater. Phys., 1992, 46, 16067–16080 CrossRef CAS PubMed.
- P. J. Feibelman, Phys. Rev. B: Condens. Matter Mater. Phys., 2001, 64, 125403 CrossRef.
- F. Che, J. T. Gray, S. Ha and J.-S. McEwen, J. Catal., 2015, 332, 187–200 CrossRef CAS.
- A. Taufik, Y. Asakura, T. Hasegawa and S. Yin, ACS Appl. Nano Mater., 2021, 4, 6861–6871 CrossRef CAS.
- K. B. Oldham, J. Electroanal. Chem., 2008, 613, 131–138 CrossRef CAS.
- J. S. Filhol and M. L. Doublet, J. Phys. Chem. C, 2014, 118, 19023–19031 CrossRef CAS.
- E. Masumian, S. M. Hashemianzadeh and A. Nowroozi, Phys. Lett. A, 2014, 378, 2549–2552 CrossRef CAS.
- I. A. Filot, R. A. van Santen and E. J. Hensen, Angew. Chem., Int. Ed., 2014, 53, 12746–12750 CrossRef CAS PubMed.
Footnote |
† Electronic supplementary information (ESI) available. See DOI: 10.1039/d1ra05764b |
|
This journal is © The Royal Society of Chemistry 2021 |