DOI:
10.1039/D1RA05416C
(Paper)
RSC Adv., 2021,
11, 29537-29542
Iron-containing palygorskite clay as Fenton reagent for the catalytic degradation of phenol in water
Received
14th July 2021
, Accepted 16th August 2021
First published on 2nd September 2021
Abstract
Heterogeneous Fenton systems have great application prospects in the catalytic degradation of organic wastewater; however, they are still not widely used in operation due to the difficulty of preparing catalysts in low yields and the high manufacturing cost. Herein, we report that a pristine iron-containing palygorskite clay can be used as a Fenton catalyst reagent without any retreatment. The composition, morphology, and structure of palygorskite clay, as well as the distribution and content of Fe element in palygorskite, were characterized via several physicochemical techniques. The degradation reaction of phenol in water was carried out as a probe reaction for the palygorskite Fenton reagent. The effects of the palygorskite content, pH value, and hydrogen peroxide concentration on the degradation efficiency of phenol were studied. Under optimum operating conditions, the chemical oxygen demand (COD) degradation efficiency of phenol reaches 94% with a reaction temperature of 20 °C and a reaction time of 15 min.
1. Introduction
Water pollution is a critical issue affecting and constraining people's life and society's development.1,2 The increased phenol pollution has become a major concern in environmental protection because they are highly toxic, carcinogenic and difficult to degrade.3,4 Traditional research methods for phenol removal include biological treatment,5 extraction,6 and wet oxidation.7 It was impossible to go into operation as all of these methods have limitations of high cost, low efficiency, secondary pollution and other factors. In recent years, advanced oxidation processes (AOPs) have been widely studied by scholars,4 which generally includes Fenton oxidation,8,9 ozone oxidation,10 sonolysis oxidation,11 super critical oxidation,12 and photochemical oxidation.13
Compared with other methods of AOPs, the Fenton oxidation is easy to operate, and the process is relatively environmentally friendly. The mechanism of the Fenton oxidation is described as following:14,15
|
Fe2+ + H2O2 → Fe3+ + OH− + ˙OH
| (1) |
|
Fe3+ + H2O2 → Fe2+ + HO2˙ + H+
| (2) |
|
Fe2+ + HO2˙ → Fe3+ + HO2−
| (3) |
|
Fe3+ + HO2˙ → Fe2+ + O2 + H+
| (4) |
|
H2O2 + ˙OH → HO2˙ + H2O
| (5) |
As mentioned above, in the presence of hydrogen peroxide (H2O2), (OH) was generated by the reaction between Fe2+ and H2O2, which can degrade the macromolecules into micromolecules or mineralize the organic molecules into inorganic matters such as CO2 and H2O. The clear disadvantage of the traditional homogeneous Fenton system was that it needed a higher concentration of Fe2+ in the solution. Some heterogeneous Fenton systems have been studied for improving the Fenton oxidation property. For example, Guo et al. synthesized a sulfur-doped α-Fe2O3 (α-Fe2O3/S) from ferrous sulfate and Na2S2O3 through a mixed hydrothermal calcination treatment for the degradation of acid orange 7 and phenol.16 Xu et al. prepared a magnetic nano-scale Fe3O4/CeO2 composite via the impregnation method as a heterogeneous catalyst for the degradation of 4-chlorophenol.17 In addition, some scholars found that the introduction of electricity, light and ultrasound can improve the Fenton oxidation efficiency, which led to the research on electron-Fenton,18,19 photo-Fenton,20,21 sono-Fenton,22,23 sono-photo-Fenton,24 sono-electron-Fenton,25 and photo-electron-Fenton.26 Although numerous methods were used for Fenton reagents to degrade organic wastewater, they are still difficult to be used in actual application because the methods are limited by the difficulty of preparing catalysts in low yields and high cost of catalyst preparation. Therefore, it is necessary to develop a simple heterogeneous Fenton system for the degradation of organics in water.
Palygorskite clay, also known as attapulgite, is a kind of hydrous magnesium-rich silicate clay mineral with a chain-layered structure.27–30 Its theoretical chemical formula is Mg5Si8O20(HO)2(OH2)4·4H2O. Palygorskite is a fibrous porous crystal. The crystal structure unit layer is arranged by 8 Si–O tetrahedrons in a 2
:
1 type layer, where Si4+ can be replaced by Fe3+ or Al3+, and Mg2+ by Fe2+, Fe3+ or Al3+. The basic crystal unit forms a bundle with a chain structure, and the crystal is acicular, fibrous, or fibrous aggregate.29 Palygorskite clay has been widely used in the fields of chemical industry, light industry, textile, building materials, environmental protection and pharmaceutical due to its specific fiber structure and excellent adsorption and decolorization properties.30 In this study, we reported that a pristine iron-containing palygorskite clay can be used as a Fenton heterogeneous reagent without any retreatment. The composition, morphology, and structure of palygorskite clay, as well as the distribution and content of Fe element within it were studied via several physicochemical techniques. The effects of palygorskite content, pH value, and hydrogen peroxide concentration on the phenol degradation efficiency were also studied and the optimum operating conditions concluded were catalyst dosage of 0.5 g L−1, initial pH = 3 and hydrogen peroxide dosage of 30 mmol L−1. This study provides a catalyst material for improving the heterogeneous Fenton oxidation property.
2. Materials and methods
2.1 Materials
All the chemical reagents (phenol, hydrogen peroxide, sulfuric acid, sodium hydroxide, and hydrochloric acid) were of A.R. grade and used without further purification. The raw palygorskite clay, provided by Gansu Cuihua Technology Co., Ltd China, was passed through an 80-mesh sieve before use.
2.2 Characterization
X-ray diffraction (XRD) pattern was collected on a Shimadzu XRD-6000 X-ray powder diffractometer with Cu Kα radiation (λ = 1.5406 Å), and the scan rate was 10° min−1. Scanning electron microscopy (SEM) was carried out on Zeiss Supra 55. High-resolution transmission electron microscopy (HRTEM) uses Tecnai G2 produced by FEI. The determination of COD was based on a 5B-3C COD rapid analyzer produced by Lianhua Technology Co., Ltd China. The content of Fe3+ in the solution after the reaction was determined via ion chromatography (ICS-90A). The composition of palygorskite clay was tested on an X-ray fluorescence spectrometer (XRF-1800).
2.3 Catalytic experiment
Unless otherwise specified, all experiments were performed using 100 mL phenol (100 mg L−1) with an appropriate pH value adjusted using 0.5 mol L−1 H2SO4 and 1.0 mol L−1 NaOH. During the experiment, 0.5 g L−1 palygorskite was added to the phenol solution and magnetically stirred for 30 min (500 rpm) to establish an adsorption/desorption equilibrium at 25 °C. Subsequently, different volumes of H2O2 (30%) were added at the required temperature. Finally, 5 mL of the mixed solution was taken out after a reaction time of 15 min and centrifuged at high speed (10
000 rpm, 3 min) to measure the COD and phenol concentration. The catalytic degradation efficiency is described as: [1 − (Cc/C0)] × 100, where C0 and Cc are the initial phenol COD value and the phenol COD value after the reaction, respectively. Effects of the palygorskite content, pH value, and hydrogen peroxide concentration on phenol degradation efficiency were also studied.
3. Results and discussion
3.1 Characterization of palygorskite clay
Fig. 1 shows the XRD pattern of raw palygorskite clay provided by Gansu Cuihua Technology Co., Ltd, China. There are numerous peaks stacked together. The highest peak was located at 2θ 26.6°, which is characteristic of the quartz (101) crystal plane. In order to analyze the specific composition of palygorskite clay, we compared the impurity crystals with the JCPDS card. We can see that besides the palygorskite (JCPDS no. 21-0958), the raw attapulgite powder includes dolomite (JCPDS no. 36-0426), clinochlore (JCPDS no. 29-0701), muscovite-3T (JCPDS no. 07-0042), feldspar (JCPDS no. 70-1862), quartz (JCPDS no. 46-1045), calciye (JCPDS no. 47-1743), gypsum (JCPDS no. 21-0816),31 and Fe2O3 (JCPDS no. 40-1139).
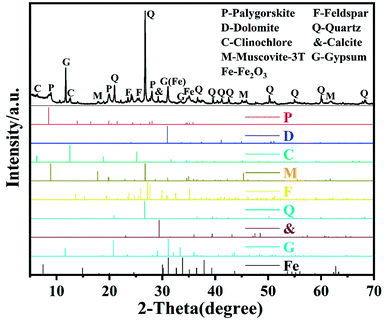 |
| Fig. 1 XRD pattern of raw palygorskite clay. | |
SEM image of palygorskite with and without ultrasonic treatment of ethanol is shown in Fig. 2. It can be seen that various crystals interacted closely and superimposed on each other without ethanol ultrasound (Fig. 2a). However, the rod-shaped palygorskite crystals could not be distinguished from the other crystals. After palygorskite was ultrasonically dispersed in ethanol, we can clearly see the rod-shaped palygorskite (Fig. 2b). Palygorskite has a length of about 0.4–3 μm. In addition, we can see numerous flake impurity crystals after ultrasonic treatment of ethanol.
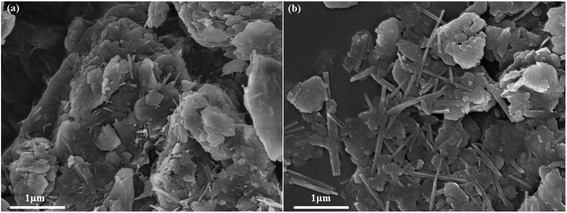 |
| Fig. 2 SEM images of palygorskite without (a) and with ethanol ultrasound (b). | |
HRTEM was used to study the fine structure of the palygorskite sample (Fig. 3). The plane spacing between adjacent stripes on the edge of the palygorskite was measured to be 0.249 nm in HRTEM (Fig. 3a), exactly matching with the (110) (JCPDS no. 33-0664) crystal plane of α-Fe2O3.32 Linear scanning maps of Mg, Al, Si and Fe were obtained along the line shown in the HRTEM of the palygorskite sample (Fig. 3b and c), which shows that α-Fe2O3 is located at the edge of the palygorskite clay. An energy dispersive spectrometer was used to test the content of the elements of palygorskite clay (Fig. 3d). It was confirmed that palygorskite clay contained Mg, Al, Si and Fe, and their contents were determined to be 8.17%, 23.38%, 43.32% and 24.11%, respectively. In addition, the XRF test shows the content of Fe2O3 to be 9.9%. We suspected that Fe exists in the form of Fe2O3 on the surface of palygorskite clay.
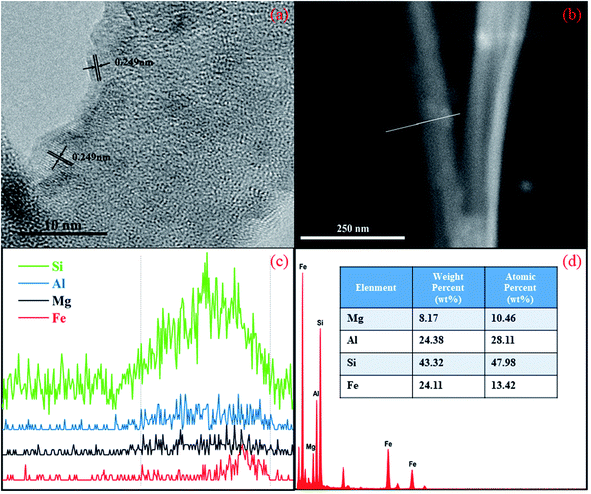 |
| Fig. 3 (a) Enlarged HRTEM image of palygorskite clay. (b) HRTEM image of palygorskite clay. (c) Linear scanning maps of Mg, Al, Si and Fe along the line shown in the HRTEM of palygorskite clay. (d) EDS of palygorskite clay. | |
3.2 Catalytic activity of palygorskite clay
3.2.1 Effect of palygorskite clay content. The effect of the amount of palygorskite clay on the phenol degradation efficiency was investigated in the range of 0.01–2 g L−1 of palygorskite (Fig. 4). As the content of palygorskite increases from 0.01 to 0.5 g L−1, the degradation efficiency of COD increases from 62% to 94%. With further increase in the quality of the palygorskite clay further increased, the COD degradation rate showed a decreasing trend, reaching a minimum of 60% at 2 g L−1. According to the mechanism of the Fenton system as described above,14,15 the palygorskite clay content was smaller, and the ˙OH radicals generated by the action between H2O2 and Fe2+ were less, leading to low phenol degradation efficiency. If we added excessive palygorskite clay, a large amount of ˙OH groups may rapidly be generated in the system. The excessive ˙OH may accumulate and react with each other to offset the utilization of ˙OH and reduce the degradation rate, thereby reducing the phenol degradation efficiency. We selected 0.5 g L−1 as the appropriate dose for subsequent experiments. Furthermore, the concentration of Fe3+ in the solution was measured after the reaction was carried out to evaluate the practicability of palygorskite in the degradation of organic wastewater. We can see that the Fe3+ concentration is lower than 5 ppm in the dosage range of 0.01–2 g L−1 of palygorskite.
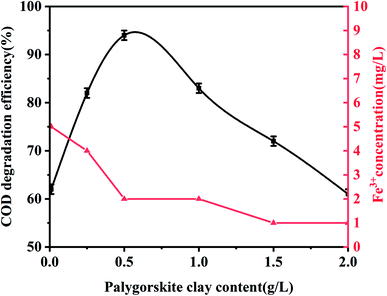 |
| Fig. 4 Effect of palygorskite content on phenol degradation efficiency (other reaction conditions: H2O2 concentration 30 mmol L−1, initial pH value = 3, reaction temperature 20 °C, and reaction time 15 min). The ordinate on the right represents the dissolution rate of Fe3+ in an aqueous solution. The results of the COD degradation efficiency presented in the graph are the average value calculated from three parallel experiments. | |
In order to evaluate the effect of the Fe content of palygorskite on the phenol degradation efficiency, raw palygorskite was acidified using 6 mol L−1 hydrochloric acid (with a liquid-to-solid ratio of 10
:
1). The XRF test shows the content of Fe2O3 was 3.5% for the acidified palygorskite. With the addition of 0.5 g L−1 palygorskite, 30 mmol L−1 H2O2 and with an initial pH value of 3, the phenol degradation efficiency was 34% for the above-acidified sample. The content of Fe2O3 in the palygorskite clay was an important factor for degradation efficiency.
3.2.2 Effect of H2O2 concentration. The effect of H2O2 concentration on the degradation rate of phenol is shown in Fig. 5. When the concentration of H2O2 increased from 10 to 30 mmol L−1, the phenol degradation efficiency improved. However, when the H2O2 increased to 130 mmol L−1, the phenol degradation efficiency slowly decreased. When the concentration of H2O2 is low, increasing H2O2 will increase ˙OH, resulting in an increase in the phenol degradation efficiency. Overdose of H2O2 will lead to the rapid oxidation of Fe2+ to Fe3+, thus consuming H2O2 and inhibiting the generation of ˙OH. 30 mmol L−1 of H2O2 will be used for the phenol degradation experiment. In addition, the Fe3+ content increases with the increase in the H2O2 concentration, which may due to the reaction between H2O2 and Fe2+ to form Fe3+.
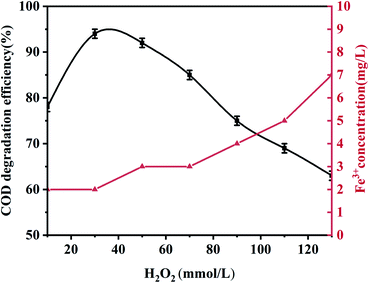 |
| Fig. 5 Effect of H2O2 concentration on phenol degradation efficiency (other reaction conditions: palygorskite content 0.5 g L−1, initial pH value of 3, reaction temperature 20 °C, and reaction time 15 min). The ordinate on the right represents the dissolution rate of Fe3+ in an aqueous solution. The results of the COD degradation efficiency presented in the graph are the average value calculated from three parallel experiments. | |
3.2.3 Effect of pH value. A relatively wide range of pH 1–9 was selected for testing the effect of pH on the Fenton reaction (Fig. 6). It was found that the phenol degradation efficiency gradually increased with the increase in pH from 1 to 3, reaching the maximum value of 94% when the pH was 3. As pH exceeds 3, the degradation efficiency gradually decreased. According to literature, the optimum pH of heterogeneous Fenton was 2.8.33,34 If the pH value is lower than the optimum value, H+ in the system would be higher, which will break the conversion between different valence states of iron. If the pH value is higher, there would be too little H+ in the system, which will inhibit the formation of ˙OH and influence degradation efficiency. Therefore, pH = 3 is selected as the optimum pH. In the experiment, the phenol degradation efficiency could be kept above 70% at a relatively broad pH of 1–9. In addition, we can see that Fe3+ decreases with the increase in pH and disappears in the solution after pH = 7. Based on the above experimental results, we found that the optimum operating conditions are the following: catalyst dosage of 0.5 g L−1, initial pH value of 3, and hydrogen peroxide dosage of 30 mmol L−1.
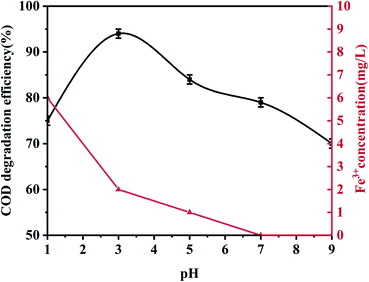 |
| Fig. 6 Effect of initial pH value on phenol degradation efficiency (other reaction conditions: palygorskite content 0.5 g L−1, H2O2 concentration 30 mmol L−1, reaction temperature 20 °C, and reaction time 15 min). The ordinate on the right represents the dissolution rate of Fe3+ in an aqueous solution. The results of the COD degradation efficiency presented in the graph are the average value calculated from three parallel experiments. | |
4. Conclusion
In summary, we reported that a pristine iron-containing palygorskite clay can be used as a heterogeneous Fenton reagent without any retreatment. Besides, palygorskite pristine powder sample includes impurity crystals such as gypsum, quartz, fluorphlogopite, and dolomite. We can clearly see the rod-shaped palygorskite after the powder sample was ultrasonically dispersed in ethanol. HRTEM and linear scanning map indicate that Fe exists in the form of Fe2O3 on the surface of the palygorskite clay. The degradation of phenol in water was carried out as a probe reaction for the palygorskite Fenton reagent. The impact of several roles, such as palygorskite content, H2O2 concentration, and pH value, on the phenol degradation efficiency has been studied. The optimum operating conditions utilized were palygorskite content of 0.5 g L−1, initial pH = 3, and hydrogen peroxide dosage of 30 mmol L−1. Under the optimum operating conditions, the COD degradation efficiency of phenol reached 94% within a reaction time of 15 min. The degradation ability of the palygorskite clay may be attributed to Fe2O3 on the surface of palygorskite.
Conflicts of interest
All authors declare that they have no conflict of interest.
Acknowledgements
This work was supported by the National Natural Science Foundation of China (No. 21376019, 21676013) and Beijing Engineering Center for Hierarchical Catalysts.
References
- L. Schweitzer and J. Noblet, Chapter 3.6 – Water Contamination and Pollution, Green Chem., 2018, 261 Search PubMed.
- Q. Wang and Z. Yang, Industrial water pollution, water environment treatment, and health risks in China, Environ. Pollut., 2016, 218, 358–365 CrossRef CAS PubMed.
- A. Haddadi and M. Shavandi, Biodegradation of phenol in hypersaline conditions by Halomonas sp. strain PH2-2 isolated from saline soil, Int. Biodeter. Biodegr., 2013, 85, 29–34 CrossRef CAS.
- A. Babuponnusami and K. Muthukumar, Advanced oxidation of phenol: a comparison between n Fenton, electro-Fenton, sono-electro-Fenton and photo-electro-Fenton processes, Chem. Eng. J., 2012, 183, 1–9 CrossRef CAS.
- H. H. P. Fang, D. W. Liang and T. Zhang, Anaerobic treatment of phenol in wastewater under thermophilic condition, Water Res., 2006, 40, 427–434 CrossRef CAS PubMed.
- W. Kujawski, A. Warszawski, W. Ratajczak, T. Porebski, W. Capafa and I. Ostrowska, Removal of phenol from wastewater by different separation techniques, Desalination, 2004, 163, 287–296 CrossRef CAS.
- A. Santos, P. Yustos, S. Gomis, G. Ruiz and F. Garcia-Ochoa, Reaction network and kinetic modeling of wet oxidation of phenol catalyzed by activated carbon, Chem. Eng. Sci., 2006, 61, 2457–2567 CrossRef CAS.
- J. J. Pignatello, E. Oliveros and A. Mackay, Advanced Oxidation Processes for Organic Contaminant Destruction Based on the Fenton Reaction and Related Chemistry, Crit. Rev. Env. Sci. Tec., 2006, 36, 1–84 CrossRef CAS.
- H. L. Zheng, Y. X. Pan and X. Y. Xiang, Oxidation of acidic dye Eosin Y by the solar photo-Fenton processes, J. Hazard. Mater., 2007, 141, 457–464 CrossRef CAS PubMed.
- M. Sunder and D. C. Hempel, Oxidation of tri- and perchloroethene in aqueous solution with ozone and hydrogen peroxide in a tube reactor, Water Res., 1997, 31(1), 40 CrossRef.
- Y. Segura, R. Molina, F. Martínez and J. A. Melero, Integrated heterogeneous sono–photo Fenton processes for the degradation of phenolic aqueous solutions, Ultrason. Sonochem., 2009, 16(3), 417–424 CrossRef CAS PubMed.
- I. V. Perez, Supercritical Water Oxidation of Phenol and 2,4-Dinitrophenol, J. Supercritical. Fluids., 2004, 30(1), 71–87 CrossRef.
- A. M. Amat, A. F. Arques, F. Lopez and M. A. Miranda, Solar photo-catalysis to remove paper mill wastewater pollutants, Sol. Energy., 2005, 79(4), 393–401 CrossRef CAS.
- E. G. Garrido-Ramirez, B. K. Theng and M. L. Mora, Clays and oxide minerals as catalysts and nanocatalysts in Fenton-like reactions – a review, Appl. Clay Sci., 2010, 47(3–4), 182–192 CrossRef CAS.
- X. G. Shi, A. Tian, J. H. You, H. Yang, Y. Z. Wang and X. X. Xue, Degradation of organic dyes by a new heterogeneous Fenton reagent – Fe2GeS4 nanoparticle, J. Hazard. Mater., 2018, 353, 182–189 CrossRef CAS PubMed.
- L. Q. Guo, F. Chen, X. Q. Fan, W. D. Cai and J. L. Zhang, S-doped α-Fe2O3 as a highly active heterogeneous Fenton-like catalyst towards the degradation of acid orange 7 and phenol, Appl. Catal., B, 2010, 96(1–2), 162–168 CrossRef CAS.
- L. J. Xu and J. L. Wang, Magnetic Nanoscaled Fe3O4/CeO2 Composite as an Efficient Fenton-Like Heterogeneous Catalyst for Degradation of 4-Chlorophenol, Environ. Sci. Technol., 2012, 46(18), 10145–10153 CrossRef CAS PubMed.
- C. Flox, S. Ammar, C. Arias, E. Brillas, A. Vargas-Zavala and R. Abdelhedi, Electro-Fenton and photoelectro-Fenton degradation of indigo carmine in acidic aqueous medium, Appl. Catal., B, 2006, 67(1–2), 93 CrossRef CAS.
- Y.-H. Huang, C.-C. Chen, G.-H. Huang and S. S. Chou, Comparison of a novel electro-Fenton method with Fenton's reagent in treating a highly contaminated wastewater, Water Sci. Technol., 2001, 43(2), 17–24 CrossRef CAS PubMed.
- S. Malato, J. Blanco, A. Vidal and C. Richter, Photocatalysis with solar energy at a pilot-plant scale: an overview, Appl. Catal., B, 2002, 37(1), 1–15 CrossRef CAS.
- F. Martínez, G. Calleja, J. A. Melero and R. Molina, Heterogeneous photo-Fenton degradation of phenolic aqueous solutions over iron-containing SBA-15 catalyst, Appl. Catal., B, 2005, 60(3–4), 181–190 CrossRef.
- H. B. Ammar, Sono-Fenton process for metronidazole degradation in aqueous solution: effect of acoustic cavitation and peroxydisulfate anion, Ultrason. Sonochem., 2016, 33, 164–169 CrossRef CAS PubMed.
- R. Molina, F. Martínez, J. A. Melero, D. H. Bremner and A. G. Chakinala, Mineralization of phenol by a heterogeneous ultrasound/Fe-SBA-15/H2O2 process: multivariate study by factorial design of experiments, Appl. Catal., B, 2006, 66(3–4), 198–207 CrossRef CAS.
- Y. Segura, R. Molina, F. Martínez and J. A. Melero, Integrated heterogeneous sono–photo Fenton processes for the degradation of phenolic aqueous solutions, Ultrason. Sonochem., 2009, 16(3), 417–424 CrossRef CAS PubMed.
- M. E. Abdelsalam and P. R. Birkin, A study investigating the sonoelectrochemical degradation of an organic compound employing Fenton's reagent, Phys. Chem. Chem. Phys., 2002, 4(21), 5340–5345 RSC.
- C. Flox, J. A. Garrido, R. M. Rodríguez, P. Cabot, F. Centellas, C. Arias and E. Brillas, Mineralization of herbicide mecoprop by photoelectro-Fenton with UVA and solar light, Catal. Today, 2007, 129(1–2), 29–36 CrossRef CAS.
- Z. J. Zheng, J. G. Cheng, Y. H. Xia, M. Chen, J. Y. Zhou and K. Xu, Study on the Purification of Palygorskite clays, Chin. Ceram. Soc., 2013, 32(12), 2471–2475 CAS.
- Z. Wang, W. Wei, Z. Z. Lin, Y. Kong, F. Wang and K. C. Yang, Research Progress on the Application of Palygorskite in Water Treatment, J. Anhui Agric. Sci., 2012, 40(16), 9048–9050 CAS.
- Z. X. Yu, Z. Y. Lu, X. H. Zhao, C. X. Li, J. M. Pan and Y. S. Yan, Palygorskite Modification and Its Application in Environmental Water Treatment, Bull. Chin. Ceram. Soc., 2010, 29(6), 1367–1372 CAS.
- P. Zhao, Y. Yao, F. Lin and C. X. Zhang, Application and Modification of Attapulgite, Chem. Pro. Technol., 2006, 5, 47–49 Search PubMed.
- J. Ren, L. L. Liu, L. Tao and C. W. Fu, Mineral Composition Analysis of Palygorskitefrom Gansu Area, Bull. Chin. Ceram. Soc., 2013, 32(11), 2362–2365 CAS.
- M. H. Chen, J. L. Liu, D. L. Chao, J. Wang, J. H. Yin, J. Y. Lin, J. F. Hong and S. Z. Xiang, Porous α-Fe2O3 nanorods supported on carbon nanotubes-graphene foam as superior anode for lithium ion batteries, Nano Energy, 2014, 9, 364–372 CrossRef CAS.
- N. N. Wang, T. Zheng, G. S. Zhang and P. Wang, A review on Fenton-like processes for organic wastewater treatment, J. Environ. Chem. Eng., 2016, 4(1), 762–787 CrossRef CAS.
- A. Babuponnusami and K. Muthukumar, A review on Fenton and improvements to the Fenton process for wastewater treatment, J. Environ. Chem. Eng., 2014, 2(1), 557–572 CrossRef CAS.
Footnote |
† These authors contributed equally to this work. |
|
This journal is © The Royal Society of Chemistry 2021 |