DOI:
10.1039/D1RA05396E
(Paper)
RSC Adv., 2021,
11, 28375-28380
Mesoporous MnFe2O4 magnetic nanoparticles as a peroxidase mimic for the colorimetric detection of urine glucose†
Received
14th July 2021
, Accepted 4th August 2021
First published on 23rd August 2021
Abstract
Mesoporous MnFe2O4 magnetic nanoparticles (mMnFe2O4 MNPs) were prepared with a one-step synthesis method and characterized to possess intrinsic peroxidase-like activity, and had obvious advantages over other peroxidase nanozymes in terms of high catalytic affinity, high stability, mono-dispersion, easy preparation, and quick separation. The mMnFe2O4 MNPs were used as a colorimetric sensor for indirect sensing of urine glucose based on the sensing principle that H2O2 can be produced from glucose oxidation catalyzed by glucose oxidase (GOx), and under the catalysis of the mMnFe2O4 MNPs nanozyme, H2O2 can oxidize 3,3′,5,5′-tetramethylbenzidine (TMB) to produce a blue color in a few minutes. This sensor is simple, cheap, sensitive, and specific to glucose detection with a detection limit of 0.7 μM, suggesting its potential for on-site glucose detection.
1. Introduction
Glucose, an important energy source in the human body, participates in many physiological and pathological functions. Irregular glucose metabolism in urine is an early warning of health problems,1 such as diabetes, a metabolic disease characterized by high glucose in blood and urine, resulting in serious damage to the human body,2 suggesting the importance of early and accurate detection of urine glucose for health monitoring. For this purpose, various biosensors have been invented for glucose detection, including electrochemical3–7 and fluorescence8–10 techniques. However, most of these biosensors are complicated in construction and sophisticated in equipment, indicating the necessity to develop a simple, low cost and easy-to-detect method for on-site monitoring of the glucose level.
Another indirect method for evaluating glucose level is to detect H2O2 generated from glucose oxidase catalyzed by glucose oxidase (GOx).11 Horseradish peroxidase (HRP), a natural enzyme catalyzing the oxidation of 3,3′,5,5′-tetramethylbenzidine (TMB) or other substrates by H2O2, is the enzyme most commonly used as a diagnostic kit for H2O2 sensing.12 However, the activity of HRP is not stable in a harsh environment (such as in extreme pH medium, organic solvents, and high temperature), and preparing pure HRP is time-consuming and expensive,13 thereby limiting the practical application of HRP as a natural enzyme. To solve these problems, an increasing number of scientists have turned their attention to the construction of efficient enzyme mimics. Since Yan et al. first reported Fe3O4 magnetic nanoparticles (NPs) as peroxidase mimics (termed as “nanozyme”),14 many other nanozymes have been developed and applied for indirect glucose detection,15–18 such as ZnO NPs,19 V2O5 nanowires,20 Au NPs,21 Pt NPs,22 and Pd NPs.23 However, the enzyme-like activity of most nanozymes can be easily affected by additional coating or modification. Therefore, it is still necessary to develop new nanozymes with high performance.
In the past few years, spinel ferrites (MFe2O4, M = Zn, Co, Ni, Cd, Mn) have attracted increasing attention due to their wide application in the fields of magnetic materials, energy, environmental protection, biomedicine, and catalytic application.24–27 Their chemical and physical properties can be effectively regulated by tuning the shape, size, and morphology.28 Thus, ferrite nanomaterials with different morphologies have been successfully prepared, such as homogeneous hollow microspheres of spinel ferrites,29 porous “timber-like” ZnFe2O4 structures30 and MnFe2O4 nanomaterials with nano-octahedron, nano-sheet or nano-wire morphologies.31 Interestingly, the MnFe2O4 nanomaterials showed high morphology-dependent oxidase-like activity, with a considerably higher oxidase-like activity for the MnFe2O4 nanomaterials with a nano-octahedron morphology than those with a nano-sheet or nano-wire morphology. Therefore, the tunable morphology-dependent enzyme-like property of the spinel ferrites has attracted the attention of an increasing number of researchers.
Here, we report the one-step green-synthesis of mesoporous MnFe2O4 magnetic nanoparticles (mMnFe2O4 MNPs) with high intrinsic peroxidase activity, uniform distribution, high stability, and rapid separation. As shown in Scheme 1, H2O2 can be generated from glucose oxidation catalyzed by GOx, and under the catalysis of the mMnFe2O4 MNPs nanozyme, TMB can be oxidized by H2O2 to produce a blue color observable with the naked eye in a few minutes. Based on this principle, we developed a colorimetric sensor for glucose detection, which showed great potential for point-of-care monitoring of urine glucose due to its easy preparation, low cost, and quick detection.
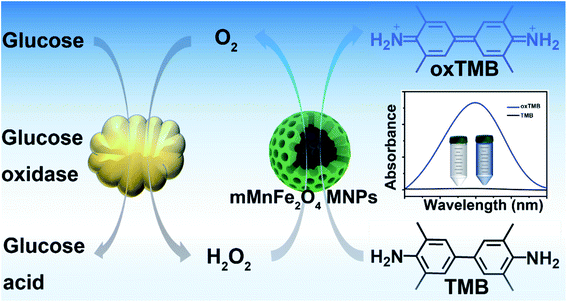 |
| Scheme 1 Schematic illustration of glucose detection with glucose oxidase (GOx) and mMnFe2O4 MNPs-catalyzed system. | |
2. Experimental section
2.1 Materials
Sodium acetate (NaAc), ferric chloride (FeCl3·6H2O), manganous dichloride (MnCl2·4H2O), ethylene glycol (EG), hydrogen peroxide (H2O2), 3,3′,5,5′-tetramethylbenzidine (TMB), fructose, lactose, and maltose were purchased from Sinopharm Chemical Reagent Co. Ltd (Shanghai, China), glucose from Sigma-Aldrich, glucose oxidase (GOx, 10 KU) from Aladdin. All other chemicals were of analytical grade and used without further purification. Deionized water was used throughout the experiment.
2.2 Apparatus and characterization
A JEOL JEM-2010FEF transmission electron microscope (TEM) and a JSM-7900F scanning electron microscope (SEM) were used for measuring the morphology and size of mMnFe2O4 MNPs. The X-ray diffraction (XRD) pattern of mMnFe2O4 MNPs was obtained with a Shimadzu XRD-7000 diffractometer with Cu Kα radiation (λ = 0.154056). The zeta potential and size of mMnFe2O4 MNPs were measured with a Malvern Nano-ZS90 apparatus. Energy dispersive X-ray spectroscopy (EDS) was performed on an Oxford X-max 80 T. X-ray photoelectron spectra (XPS) were acquired using an ESCALAB 250Xi electron spectrometer. The spectra were measured using Al Kα radiation (12.5 kV, 400 W) in a chamber with a base pressure of 8 × 10−8 Pa. UV-Vis spectra were collected using a Shimadzu UV-2450 spectrophotometer.
2.3 Synthesis of mMnFe2O4 MNPs
The mMnFe2O4 MNPs were synthesized with a solvothermal method as previously reported with small modifications.32 Briefly, NaAc (2.7 g) was first completely dissolved in EG (30 mL) under ultrasonication for 10 min, followed by adding MnCl2·4H2O and FeCl3·6H2O at a molar ratio of 1
:
2. Next, the mixture was transferred into a Teflon autoclave and sealed to heat overnight at 180 °C. After cooling to room temperature naturally, the mMnFe2O4 MNPs were obtained with magnet, followed by three washes with water and ethanol and drying at 30 °C. Finally, the dried mMnFe2O4 MNPs were stored at room temperature for further use.
2.4 Peroxidase activity, reaction-rate factors and steady-state kinetics
The peroxidase activity of mMnFe2O4 MNPs was studied using TMB as the substrate. Briefly, mMnFe2O4 MNPs (10 μg mL−1) were quickly mixed with 2.0 mL solution of TMB (400 μM) and H2O2 (20 mM) in citric buffer (25 mM, pH 4.0), the absorbance at 652 nm was recorded. Similarly, the factors affecting the rate of catalytic reaction, such as pH, temperature, reaction time, salt concentration, H2O2 concentration, and steady-state kinetics, were investigated using TMB as the substrate. Finally, the initial rates were calculated from the plot of absorbance versus time.
2.5 Glucose sensing
For glucose sensing, 20 μL of GOx (10 mg mL−1, pH = 7) was first mixed separately with 180 μL of glucose solution at different concentration (0.0, 0.5, 2.5, 5.0, 10.0, 16.0, 50.0, 100.0, 500.0, 1000.0 μM) (pH = 7), and incubated at 37 °C for 30 min. Then, the mixture was supplemented with 1770 μL of citric buffer (25 mM, pH 4.0), 10 μL of mMnFe2O4 MNPs (2 mg mL−1) and 20 μL TMB solution (40 mM). After incubation at 37 °C for 30 min, the absorbance of the resulting solution was measured at 652 nm. Meanwhile, the control experiments were performed using maltose, lactose, and fructose to replace glucose in a similar way.
3. Results and discussion
3.1 Characterization of mMnFe2O4 MNPs
Fig. 1 shows the SEM and TEM images of mMnFe2O4 MNPs. In the SEM and TEM images, mMnFe2O4 MNPs were seen to present uniform nanocrystals with a size of nearly 180 nm and a well-defined meso-structure. Fig. S1,† shows the dynamic light scattering (DLS) size distribution of mMnFe2O4 MNPs. In Fig. S2,† the EDS result showed the existence of Mn, Fe and O elements. The nanoparticles were further characterized by X-ray photoelectron spectroscopy (XPS) (Fig. S3†), and the XPS results are consistent with the previous reports.31 The above results confirmed that successful synthesis of mMnFe2O4 nanoparticles.
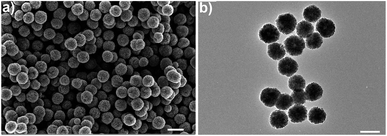 |
| Fig. 1 (a) SEM image (scale bar 300 nm), (b) TEM image (scale bar 200 nm). For specificity test, the system was incubated with interference ions (Cd2+, Pb2+, Zn2+, Cu2+, Fe3+, K+, Na+, As3+, Cr3+, Mn2+, Ag+, Ca2+, Ba2+, Mg2+; Cl−, Br−, NO3−, SO42−, CO32−, F−, OH−) at a high concentration of 500 μM, and the other procedures were the same as described above. For urine sample tests, urine samples were collected and filtered through a filter with pore size of 0.22 μm, and different concentration of glucose were added as described above. Finally, the absorbance of the samples was measured at 652 nm using a spectrophotometer. | |
The structure of mMnFe2O4 nanoparticles was investigated by FT-IR (Fig. S4†) and XRD (Fig. S5†). All the diffraction peaks are consistent with the FCC structure of mMnFe2O4 with high crystallinity (JCPDS card no. 74-2403).33 The porous structure of the nanocrystals was investigated by N2 adsorption–desorption analysis (Fig. S6a†). The mesopore size was shown to be about 7.6 nm from the HK pore size distribution curve (Fig. S6b†), and the surface area was calculated to be 53.4 m2 g−1. The magnetic property of mMnFe2O4 MNPs was characterized by field dependent magnetization (Fig. S7†), and mMnFe2O4 MNPs were shown to possess superparamagnetic property with 70 emu g−1 of maximum saturation magnetization (Ms) value, which is almost comparable to the Ms value of Fe2O3 nanoparticles.34
3.2 Peroxidase-like activity of mMnFe2O4 MNPs
After treatment with mMnFe2O4 MNPs and H2O2, the colorless TMB solution rapidly turned blue, with the blue color from the oxidized TMB product (oxTMB). In, Fig. 2a, the UV-vis absorbance of oxTMB at 652 nm was seen to be obviously enhanced in the mixture of mMnFe2O4 MNPs + TMB + H2O2. However, neither H2O2 nor mMnFe2O4 MNPs alone can efficiently oxidize TMB to produce a blue color. Therefore, TMB can be oxidized by H2O2 under the catalysis of mMnFe2O4 MNPs, which is also the case for horseradish peroxidase (HRP). These results indicated that mMnFe2O4 MNPs may possess an intrinsic peroxidase-like activity, which was further characterized by using other peroxidase substrates, such as 2,2-azino-bis (3-ethylbenzothiazoline-6-sulfonic acid) (ABTS) and o-phenylenediamine (OPD), to replace TMB (Fig. 2b), and mMnFe2O4 MNPs were shown to possess a broad peroxidase-like activity. Moreover, in Fig. S8,† the peroxidase-like activity was observed to be caused by mMnFe2O4 MNPs rather than ions leached from mMnFe2O4 MNPs in acidic solution.
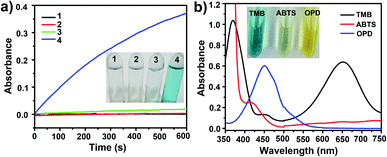 |
| Fig. 2 (a) Feasibility study of H2O2 sensing based on the catalytic ability of mMnFe2O4 MNPs characterized by UV-vis spectroscopy at 652 nm. (1) TMB; (2) mMnFe2O4 MNPs + TMB; (3) TMB + H2O2; (4) mMnFe2O4 MNPs + TMB + H2O2. (b) UV-vis spectra of mMnFe2O4 MNPs to produce different colors under different substrates (TMB, ABTS and OPD). Inset: the corresponding photographs. | |
The kinetic mechanism of the peroxidase-like activity of mMnFe2O4 MNPs was investigated by analyzing apparent steady-state kinetic parameters at varying TMB concentrations (50–800 μM). The typical Michaelis–Menten curves were obtained by measuring the absorbance of TMB at 652 nm for 300 s (Fig. 3a). Michaelis–Menten constant (Km) and maximum initial velocity (Vmax) were calculated through Lineweaver–Burk plots (Fig. 3b and c). It was reported the smaller the Km value, the stronger the affinity between enzyme and substrate.35 The apparent Km value for the mMnFe2O4 MNPs with TMB as the substrate is 0.07 mM−1, which is significantly lower than that of the other catalysts (Table S1†), suggesting higher affinity of mMnFe2O4 MNPs for TMB. This could be due to the mesoporous structure of mMnFe2O4 MNPs, which can enhance the up-take of TMB by mMnFe2O4 MNPs and strengthen the catalytic activity. In Fig. 3d, mMnFe2O4 MNPs were shown to decompose H2O2 to produce ·OH radical, which is consistent with previous report that the catalytic mechanism of nanozymes is to bind and react with H2O2 and then release hydroxyl radical (OH) to react with TMB.36
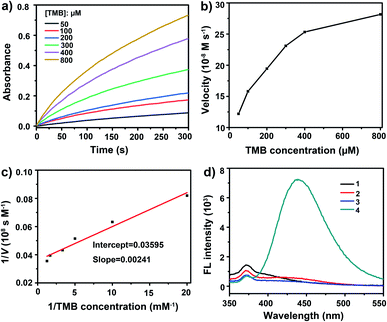 |
| Fig. 3 Steady-state kinetic of peroxidase-like activity of mMnFe2O4 MNPs. (a) Time-dependent absorbance changes at 652 nm of TMB catalyzed by mMnFe2O4 MNPs in the presence of TMB at different concentrations; (b) the velocity of reaction changes in the presence of TMB at different concentrations; (c) Lineweaver–Burk plot corresponding to (b). (d) Detecting hydroxyl radical (·OH) with fluorescence spectra of TA in the different mediums. (1) TA; (2) TA + mMnFe2O4 MNPs; (3) TA + H2O2; (4) TA + mMnFe2O4 MNPs + H2O2. | |
3.3 Optimization of experimental conditions
Like natural enzyme HRP, the peroxidase-like activity of mMnFe2O4 MNPs is dependent on reaction conditions. The catalytic activity of mMnFe2O4 MNPs is much higher in acidic solutions (Fig. S9a†). In Fig. S10,† the SEM result showed that the morphology of mMnFe2O4 MNPs remained almost intact at pH 4.0 for 2 h. Considering the stability and catalytic efficiency of mMnFe2O4 MNPs, pH 4.0 was defined as the optimal pH value experiment. Meanwhile, the incubation temperature of mMnFe2O4 MNPs with TMB and H2O2 was also investigated and the results are shown in Fig. S9b,† with 37 °C as the optimal temperature. Additionally, the optimal concentration of H2O2 was investigated in the range of 0 to 60 mM, and the absorbance at 652 nm obviously increased and reached a plateau at 20 mM (Fig. S9c†), so 20 mM was chosen as the optimal H2O2 concentration for further experiments. Similarly, 0.4 mM of TMB (Fig. S9d†) and 10 μg mL−1 of mMnFe2O4 MNPs (Fig. S9e†) were found to the optimal parameters for further experiments. Furthermore, the incubation time of mMnFe2O4 MNPs with TMB and H2O2 was also optimized to achieve the best catalytic activity of the nanozymes (Fig. S9f†), and 15 min was shown as the optimal incubation time. In this study, the optimized experimental conditions consisted of pH 4.0, 37 °C, 20 mM of H2O2, 0.4 mM of TMB, 10 μg mL−1 of mMnFe2O4 MNPs, and 15 min of incubation time.
Besides, the enzymatic-like reaction activity of mMnFe2O4 MNPs was shown not to be affected by adding NaCl (0–0.25 M) to the reaction media (Fig. S11†), confirming the high stability of our nanozymes in NaCl media.
3.4 Application to glucose detection
Since the catalytic activity of mMnFe2O4 MNPs depends on H2O2, H2O2 is the main product of glucose oxidation catalyzed by glucose oxidase (GOx). When combined with GOx, the mMnFe2O4 MNPs could be used for glucose detection instead of traditional HRP. Fig. 4a shows the glucose concentration-response curves to the absorbance of oxTMB. The linear range for glucose was from 0.5 to 16.0 μM with a detection limit of 0.7 μM (S/N ≥ 3) (Fig. 4b), which was lower than the detection limit of several previously reported nanozyme-based methods (Table S2†).
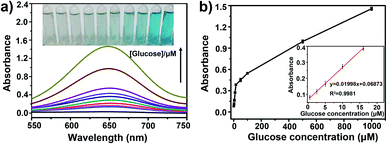 |
| Fig. 4 Biosensing performance of the colorimetric sensor with different glucose concentrations. (a) UV-vis spectra of TMB + mMnFe2O4 MNPs in the presence of varying concentrations of glucose (from bottom to top: 0.0, 0.5, 2.5, 5.0, 10.0, 16.0, 50.0, 100.0, 500.0, 1000.0 μM). Inset: the corresponding photographs, from left to right. (b) Calibration curve of absorbance at 652 nm vs. glucose concentration. Inset: linear relationship of absorbance at 652 nm vs. glucose concentration. | |
3.5 Specificity test
Specificity is important for the application of mMnFe2O4 MNPs probes. The specificity tests were performed in the presence of common ions (14 cations: Cd2+, Pb2+, Zn2+, Cu2+, Fe3+, K+, Na+, As3+, Cr3+, Mn2+, Ag+, Ca2+, Ba2+, Mg2+; 7 anions: Cl−, Br−, NO3−, SO42−, CO32−, F−, OH−), which did not interfere with H2O2 determination (Fig. 5a and b), confirming that the mMnFe2O4 MNPs probe is specific to H2O2. The specificity of the mMnFe2O4 MNPs nanozyme toward glucose detection was investigated by performing the control experiments using 10 mM of fructose, lactose, and maltose to replace glucose (1 mM). In Fig. 5c and d, the absorbance at 652 nm for glucose was seen to be obviously higher than that of glucose analogues, confirming that the mMnFe2O4 MNPs nanozyme is highly specific to glucose, probably due to the high affinity of glucose oxidase for glucose.
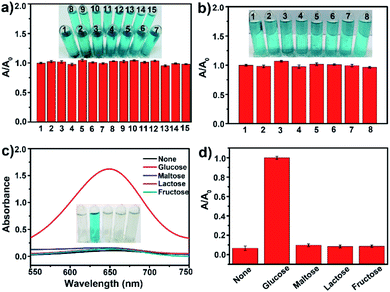 |
| Fig. 5 Specificity tests. (a) The absorbance ratio at 652 nm for interference cations (from 1 to 15: none, Cd2+, Pb2+, Zn2+, Cu2+, Fe3+, K+, Na+, As3+, Cr3+, Mn2+, Ag+, Ca2+, Ba2+, Mg2+). Inset: the corresponding photographs. (b) The absorbance ratio at 652 nm for interference anions (from 1 to 8: none, Cl−, Br−, NO3−, SO42−, CO32−, F−, OH−). Inset: the corresponding photographs; determination of the specificity of glucose detection. (c) UV-vis spectra of TMB + mMnFe2O4 MNPs + GOx system in the absence (none) and presence of glucose (1 mM), maltose (10 mM), lactose (10 mM), and fructose (10 mM). Inset: the variation of colors with different solutions. (d) The absorbance ratio at 652 nm for (c). The error bars represent the standard deviation of three measurements. | |
3.6 Real sample test
The potential of the mMnFe2O4 MNPs nanozyme for glucose detection was further evaluated by detecting glucose in urine samples from healthy people and a diabetic volunteer. The UV-vis spectra and the colorimetric differentiation are shown in Fig. S12.† According to the calibration curve, the concentration of glucose was 8.2 mM (147.6 mg dL−1) in the diabetic urine, but could hardly be detected in healthy human's urine. The obtained values were further analyzed by spiking different concentrations of glucose into the healthy human's urine and recovering them (Table S3†). Based on the recovery rate and the relative standard deviation (RSD), there was no significant difference between the spiked value and the detected value, indicating the potential of this colorimetric probe for real sample testing.
4. Conclusion
In paper, we reported the synthesis and characterization of the mMnFe2O4 MNPs with intrinsic peroxidase-like activity as well as their application in colorimetric detection of urine glucose. As a novel peroxidase nanozyme, the mMnFe2O4 MNPs nanozyme shows several advantages over HRP and other peroxidase nanozymes, such as mesoporous nanostructure, high stability, good dispersibility, excellent separability, and high catalytic efficiency. Based on the reaction mechanism of Scheme 1, we developed a simple, low-cost, highly sensitive, and selective colorimetric method for glucose detection, which shows the practicability of glucose detection in the urine of healthy and diabetic people, suggesting its potential as a simple and useful probe for bioanalysis. The overall results indicate that the mMnFe2O4 MNPs nanozyme may have broad application prospect as a powerful artificial peroxidase.
Ethical statement
For the human urine experiments, informed consent was obtained from all human subjects. All experiments were performed in compliance with relevant laws or guidelines. All experiments followed institutional guidelines, and were approved by the institutional committee.
Conflicts of interest
There are no conflicts of interest to declare.
Acknowledgements
The authors gratefully acknowledge the financial supports from National Natural Science Foundation of China (21904046, 31772785, 21205043) and the Fundamental Research Funds for the Central Universities (2662018QD042).
References
- J. Lu, R. F. Bu, Z. L. Sun, Q. S. Lu, H. Jin, Y. Wang, S. H. Wang, L. Li, Z. L. Xie and B. Q. Yang, Diabetes Res. Clin. Pract., 2011, 93, 179–186 CrossRef CAS PubMed.
- A. Untereiner and L. Y. Wu, Antioxid. Redox Signaling, 2017, 28, 1463–1482 CrossRef PubMed.
- T. Kangkamano, A. Numnuam, W. Limbut, P. Kanatharana and P. Thavarungkul, Sens. Actuators, B, 2017, 246, 854–863 CrossRef CAS.
- H. Imran, K. Vaishali, S. A. Francy, P. N. Manikandan and V. Dharuman, Bioelectrochemistry, 2021, 137, 107645–107655 CrossRef CAS PubMed.
- Q. Han, H. Wang, D. Wu and Q. Wei, Biosens. Bioelectron., 2021, 173, 112803–112808 CrossRef CAS PubMed.
- M. Palmer, M. Masikini, L. W. Jiang, J. J. Wang, F. Cummings, J. Chamier, O. Inyang and M. Chowdhury, J. Alloys Compd., 2021, 853, 156900–156910 CrossRef CAS.
- X. Wei, J. Guo, H. Lian, X. Sun and B. Liu, Sens. Actuators, B, 2021, 329, 129205–129212 CrossRef CAS.
- M.-M. Farahani, F. Ghorbani and N. Mosleh, Spectrochim. Acta, Part A, 2021, 245, 118892–118897 CrossRef.
- Y. L. Ngo, P. L. Nguyen, J. Jana, W. M. Choi, J. S. Chung and S. H. Hur, Anal. Chim. Acta, 2021, 1147, 187–198 CrossRef CAS.
- K. Kim, H. Kim, E. J. Jo, H. Jang, J. Park, G. Y. Jung and M. G. Kim, Biosens. Bioelectron., 2021, 175, 112855–112860 CrossRef CAS PubMed.
- A. Tereshchenko, M. Bechelany, R. Viter, V. Khranovskyy, V. Smyntyna, N. Starodub and R. Yakimova, Sens. Actuators, B, 2016, 229, 664–677 CrossRef CAS.
- D. Sodzel, V. Khranovskyy, V. Beni, A. P. F. Turner, R. Viter, M. O. Eriksson, P. O. Holtz, J. M. Janot, M. Bechelany, S. Balme, V. Smyntyna, E. Kolesneva, L. Dubovskaya, I. Volotovski, A. Ubelis and R. Yakimova, Microchim. Acta, 2015, 182, 1819–1826 CrossRef CAS.
- J. M. Perez, Nat. Nanotechnol., 2007, 2, 535–536 CrossRef CAS PubMed.
- L. Z. Gao, J. Zhuang, L. Nie, J. B. Zhang, Y. Zhang, N. Gu, T. H. Wang, J. Feng, D. L. Yang, S. Perrett and X. Y. Yan, Nat. Nanotechnol., 2007, 2, 577–583 CrossRef CAS PubMed.
- J. Wu, X. Wang, Q. Wang, Z. Lou, S. Li, Y. Zhu, L. Qin and H. Wei, Chem. Soc. Rev., 2019, 48, 1004–1076 RSC.
- S. Y. Jin, C. Wu, Z. Z. Ye and Y. B. Ying, Sens. Actuators, B, 2018, 283, 18–34 CrossRef.
- Y. Y. Huang, J. S. Ren and X. G. Qu, Chem. Rev., 2019, 119, 4357–4412 CrossRef CAS PubMed.
- Y. H. Lin, J. S. Ren and X. G. Qu, Acc. Chem. Res., 2014, 47, 1097–1105 CrossRef CAS PubMed.
- A. Asati, S. Santra, C. Kaittanis, S. Nath, J. M. Perez and J. Manuel, Angew Chem. Int. Ed., 2010, 121, 2344–2348 CrossRef.
- Y. B. Feng, L. Hong, A. L. Liu, W. D. Chen, G. W. Li, W. Chen and X. H. Xia, Int. J. Environ. Sci. Technol., 2015, 12, 653–660 CrossRef CAS.
- J. X. Wang, Y. Zhuo, Y. Zhou, H. J. Wang, R. Yuan and Y. Q. Chai, ACS Appl. Mater. Interfaces, 2016, 8, 12968–12975 CrossRef CAS PubMed.
- R. André, F. Natálio, M. Humanes, J. Leppin, K. Heinze, R. Wever, H. C. Schröder, W. E. G. Müller and W. Tremel, Adv. Funct. Mater., 2011, 21, 501–509 CrossRef.
- Y. H. Lin, J. S. Ren and X. G. Qu, Adv. Mater., 2014, 26, 4200–4217 CrossRef CAS PubMed.
- J. Kim, H. R. Cho, H. Jeon, D. Kim, C. Song, N. Lee, S. H. Choi and T. Hyeon, J. Am. Chem. Soc., 2017, 139, 10992–10995 CrossRef CAS.
- S. Ida, K. Yamada, T. Matsunaga, H. Hagiwara, Y. Matsumoto and T. Ishihara, J. Am. Chem. Soc., 2010, 132, 17343–17345 CrossRef CAS PubMed.
- S. H. Xuan, F. Wang, Y. X. Wang, J. C. Yu and K. C. F. Leung, J. Mater. Chem., 2010, 20, 5086–5094 RSC.
- C. Burda, X. B. Chen, R. Narayanan and M. A. El-Sayed, Chem. Rev., 2005, 105, 1025–1102 CrossRef CAS PubMed.
- N. Z. Bao, L. M. Shen, Y. H. A. Wang, J. X. Ma, D. Mazumdar and A. Gupta, J. Am. Chem. Soc., 2009, 131, 12900–12901 CrossRef CAS.
- Z. M. Li, X. Y. Lai, H. Wang, D. Mao, X. J. Xing and D. Wang, J. Phys. Chem. C, 2009, 113, 2792–2797 CrossRef CAS.
- M. Wang, Z. H. Ai and L. Z. Zhang, J. Phys. Chem. C, 2008, 112, 13163–13170 CrossRef CAS.
- A. A. Vernekar, T. Das, S. Ghosh and G. Mugesh, Chem.–Asian J., 2016, 11, 72–76 CrossRef CAS PubMed.
- X. Y. Long, J. Y. Li, D. Sheng and H. Z. Lian, Talanta, 2017, 166, 36–45 CrossRef CAS PubMed.
- M. A. Peluso, L. A. Gambaro, E. Pronsato, D. Gazzoli, H. J. Thomas and J. E. Sambeth, Catal. Today, 2008, 133, 487–492 CrossRef.
- S. Laurent, D. Forge, M. Port, A. Roch, C. Robic, L. V. Elst and R. N. Muller, Chem. Rev., 2008, 108, 2064–2110 CrossRef CAS PubMed.
- Y. Liu, D. L. Purich, C. C. Wu, Y. Wu, T. Chen, C. Cui, L. Q. Zhang, S. Cansiz, W. J. Hou, Y. Y. Wang, S. Y. Yang and W. H. Tan, J. Am. Chem. Soc., 2015, 137, 14952–14958 CrossRef CAS PubMed.
- L. Su, J. Feng, X. Zhou, C. L. Ren, H. H. Li and X. G. Chen, Anal. Chem., 2012, 84, 5753–5758 CrossRef CAS PubMed.
Footnotes |
† Electronic supplementary information (ESI) available. See DOI: 10.1039/d1ra05396e |
‡ These authors contributed equally to this work. |
|
This journal is © The Royal Society of Chemistry 2021 |
Click here to see how this site uses Cookies. View our privacy policy here.