DOI:
10.1039/D1RA04763A
(Paper)
RSC Adv., 2021,
11, 26336-26343
Tuning the microstructural and magnetic properties of CoFe2O4/SiO2 nanocomposites by Cu2+ doping
Received
20th June 2021
, Accepted 26th July 2021
First published on 2nd August 2021
Abstract
Co–Cu ferrite is a promising functional material in many practical applications, and its physical properties can be tailored by changing its composition. In this work, Co1−xCuxFe2O4 (0 ≤ x ≤ 0.3) nanoparticles (NPs) embedded in a SiO2 matrix were prepared by a sol–gel method. The effect of a small Cu2+ doping content on their microstructure and magnetic properties was studied using XRD, TEM, Mössbauer spectroscopy, and VSM. It was found that single cubic Co1−xCuxFe2O4 ferrite was formed in amorphous SiO2 matrix. The average crystallite size of Co1−xCuxFe2O4 increased from 18 to 36 nm as Cu2+ doping content x increased from 0 to 0.3. Mössbauer spectroscopy indicated that the occupancy of Cu2+ ions at the octahedral B sites led to a slight deformation of octahedral symmetry, and Cu2+doping resulted in cation migration between octahedral A and tetrahedral B sites. With Cu2+ content increasing, the saturation magnetization (Ms) first increased, then tended to decrease, while the coercivity (Hc) decreased continuously, which was associated with the cation migration. The results suggest that the Cu2+ doping content in Co1−xCuxFe2O4 NPs plays an important role in its magnetic properties.
1. Introduction
Cobalt ferrite (CoFe2O4) with moderate saturation magnetization, high coercivity and Curie temperature, as well as excellent chemical stability has gained increasing attention in technological applications, such as magnetic recording, catalysis, bio-targeted drug delivery, magnetic resonance imaging, and spintronics.1–7 In general, CoFe2O4 possesses a cubic inverse spinel structure with the Fd
m space group,in which Co2+ ions predominantly occupy octahedral B sites and Fe3+ ions are almost equally distributed between tetrahedral A and octahedral B sites. However, cation distribution between the A and B sites varies with the chemical composition and synthesis procedure. Designing the composition through the incorporation of divalent metal ions (such as Zn2+, Mn2+, Cu2+, and Ni2+) serves as a flexible strategy to tune the cation distribution of CoFe2O4 nanoparticles (NPs), which may be beneficial to further modify their physical properties or introduce novel functionalities.8–10
Recently Co–Cu ferrite, prepared through doping Cu2+ in CoFe2O4 NPs has been widely exploited for a variety of technological applications. Venkateshwarlu et al.8 reported that the increasing Seebeck coefficient was observed in CoFe2O4 after doping with Cu2+ ions. The enhanced effect of Cu2+ doping on photocatalytic degradation efficiency of CoFe2O4 was reported by Sundararajan et al.4 They also found that with Cu2+ content increasing, the saturation magnetization (Ms) decreased monotonously while the coercivity (Hc) first increased then decreased. Sanpo et al.11 demonstrated the substitution of Cu2+ ions into CoFe2O4 could improve the antibacterial property on against multidrug-resistant E. coli and Staphyloc occus aureus. These experimental results suggest that Cu2+ doping content in CoFe2O4 significantly influences their physical property. However, it is well known that copper ferrite (CuFe2O4) can exist in face-centered cubic and face-centered tetragonal phases due to obvious Jahn–Teller distortion of Cu2+ ions.13 Thus, when larger content of Cu2+ ions was doped in CoFe2O4 lattice, the crystal structure can transfer from cubic to tetragonal phase.12–14 Balavijayalakshmi et al. have reported that as the Cu2+ doping content x was >0.6, tetragonal CuFe2O4 can be observed in cubic Co1−xCuxFe2O4 NPs prepared by co-precipitation method.12 With small content of Cu2+ ions doping in CoFe2O4 NPs, the crystal microstructure and physical properties can be tailored and investigated without undesired phase transformation. To date, a limited extent of work has been found in the literature on the microstructural investigation of Co–Cu ferrites with small Cu2+ doping content.
Magnetic CoFe2O4 NPs prepared by chemical method are prone to agglomerate, which makes it quite difficult to exploit their unique physical properties for practical applications. Two strategies have been developed to stabilize and reduce nanoparticle agglomeration, obtaining single phase ferrite. One is coating CoFe2O4 NPs with a uniform and stable ultrathin layer to form core–shell NPs. Since the thickness of the coating layer (such as ultrathin phosphate layer15 and silicon carbide layer16) is only of a few nanometers, the magnetic properties of the CoFe2O4 core are not compromised after capping. The other is dispersing CoFe2O4 NPs in non-magnetic matrix to form nanocomposites, for example, dispersing CoFe2O4 in amorphous SiO2, i.e. CoFe2O4/SiO2 nanocomposites.17–20 For SiO2-based nanocomposites, SiO2 network can not only provide spatial nucleation sites for CoFe2O4 NPs, promote the formation of single-phase spinel, but also minimize the surface roughness and spin disorder, thereby enhance the magnetic properties of nanocomposites.21,22
In this work, we prepared Co1−xCuxFe2O4/SiO2 nanocomposites (0 ≤ x ≤ 0.3) using sol–gel method, in which SiO2 was used to obtain monophasic Co–Cu ferrites. With small amount Cu2+ ion doping, the crystal microstructure and physical properties were tailored without phase transformation. The goal of the present work is to study the effect of the small amount of Cu2+ doping on the microstructure, the hyperfine interaction, and magnetic properties of Co1−xCuxFe2O4 by using X-ray diffractometer (XRD), Mössbauer spectroscopy, and vibrating sample magnetometer (VSM) at room temperature. The result shows that the crystallite size of Co1−xCuxFe2O4 increases with Cu2+ content. The Cu2+ doping in CoFe2O4 induces a slight deformation of octahedral symmetry and change in cation distribution, which in turn modifies the values of Ms and Hc.
2. Experiments
2.1 Synthesis of Co1−xCuxFe2O4/SiO2 nanocomposites
The synthesis diagram for Co1−xCuxFe2O4/SiO2 nanocomposites (70% wt. ferrite/30% wt. SiO2) is presented in Fig. 1. Using cobalt nitrate hexahydrate (Co(NO3)2·6H2O), ferric nitrate nonahydrate (Fe(NO3)3·9H2O), copper(II) nitrate trihydrate (Cu(NO3)2·3H2O) as iron, cobalt, and copper sources, and tetraethyl orthosilicate (TEOS) as precursor of SiO2, a series of Co1−xCuxFe2O4/SiO2 nanocomposites (x = 0, 0.1, 0.2, and 0.3) were synthesized by sol–gel method. Firstly, the metal nitrates were weighted by the designed molar ratio and thoroughly dissolved in ethanol with magnetic stirring. Then, 1.5 mL ethylene glycol and 9.6 mL TEOS ethanol solution (volume ratio of 1
:
1) was injected into the solution, followed by adding 1 mL HNO3 and continuously stirring for 5 h. Secondly, the solution was evaporated on a 60 °C water bath to form black brown sol. After that, the sol was dried at 100 °C for at least 24 h to form xerogel. Finally, the obtained gel was calcined at 1000 °C for 2 h in air and cooled to room temperature. The final collected product was taken for further investigation.
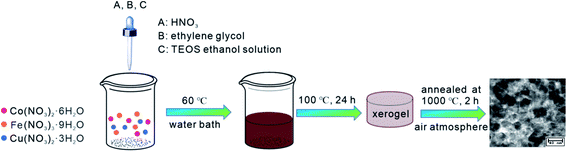 |
| Fig. 1 Schematic diagram of the synthesis method for Co1−xCuxFe2O4/SiO2 nanocomposites. | |
2.2 Characterization
The crystal structure, morphology, and magnetic properties of the as-prepared Co1−xCuxFe2O4/SiO2 were investigated by Rigaku D/max-2500 X-ray diffractometer (XRD, λ = 1.5406 Å), JEM-2100HR transmission electron microscope (TEM), and LakeShore7407 vibrating sample magnetometer (VSM, B = 1.5 T), respectively. The crystallite size of Co1−xCuxFe2O4 was estimated by using Scherrer’s formula. The room temperature Mössbauer spectra were collected on a FAST Comtec Mössbauer system in transmission mode, using a 57Co(Pd) source and a conventional constant acceleration mode. The Mössbauer spectra of the samples were fitted using Lorentzian lines via the least square method.
3. Results and discussion
3.1 Structure and morphology analysis
XRD patterns of the as-prepared Co1−xCuxFe2O4/SiO2 samples are shown in Fig. 2. The diffraction peaks from (111), (220), (311), (222), (321), (400), (422) and (511) are consistent with the standard spectrum of cubic spinel CoFe2O4 (JCPDS no. 22-1086), which demonstrates the formation of Co–Cu ferrite with no detectable impurity phases. No reflection from SiO2 can be detected in XRD patterns due to the low content of amorphous SiO2. With increasing Cu2+ content, the diffraction peak (311) shifts from 35.455° to 35.374° with a small Δθ (0.081°) accompanied by increasing peak intensity and the narrower peak width.
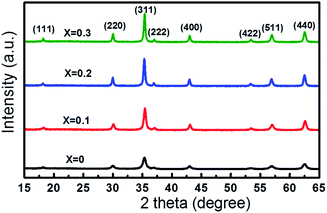 |
| Fig. 2 XRD patterns of the as-synthesized Co1−xCuxFe2O4/SiO2 with different Cu2+ content. | |
Fig. 3 presents the variation of lattice parameter and crystallite size of Co1−xCuxFe2O4 with Cu2+ doping content. The lattice parameter was determined from the X-ray data with MDI Jade 6.5 software using the high-purity silicon powders as a standard sample. It can be seen that the lattice parameter ao of 8.383 Å for the sample with x = 0 is in agreement with the reported value of pure CoFe2O4.23 As Cu2+ content increases from 0 to 0.3, the lattice parameter ao slightly increases from 8.383 to 8.389 Å. The increase in lattice parameter can be attributed to the difference in ionic radius of Co2+ (0.74 Å) and Cu2+ (0.76 Å).4,24 Furthermore, the average crystallite size, calculated with Scherrer equation is found to increase with increasing Cu2+ content (18, 26, 35 and 36 nm for Co1−xCuxFe2O4 with x = 0, 0.1, 0.2, and 0.3, respectively). This indicates that the Cu2+ doping in CoFe2O4 NPs favors the grain growth rate during sol–gel preparation process. Similar phenomenon in crystallite size has been also observed by Ashour et al. and Dippong et al.25,26
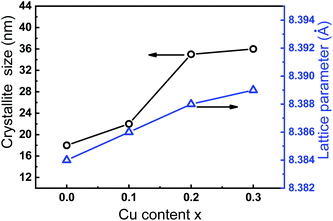 |
| Fig. 3 Plot of lattice parameter and crystallite size of Co1−xCuxFe2O4/SiO2 as a function of Cu2+ content. | |
TEM images of Co1−xCuxFe2O4/SiO2 samples with x = 0 (Fig. 4a) and x = 0.2 (Fig. 4b) are shown in Fig. 4. It can be seen that near-spherical Co–Cu ferrites are environed by amorphous SiO2 without obvious agglomerate. The average sizes are estimated to be 19 ± 5 nm (x = 0) and 39 ± 9 nm (x = 0.2), respectively, which are consistent with the results determined by XRD. Fig. 4c presents the selective area electron diffraction (SAED) pattern for the x = 0.2 sample. The diffraction rings are indexed as lattice plane (111), (220), (311), (400), (511), and (440) for spinel Co0.8Cu0.2Fe2O4, which is in agreement with the XRD result. The high resolution TEM (HRTEM) image of Co0.8Cu0.2Fe2O4 in Fig. 4d confirms that the sample is of good crystalline quality, and the clear space fringe with an interplanar spacing of 0.221 nm agrees with the (400) planes of CoFe2O4NPs.
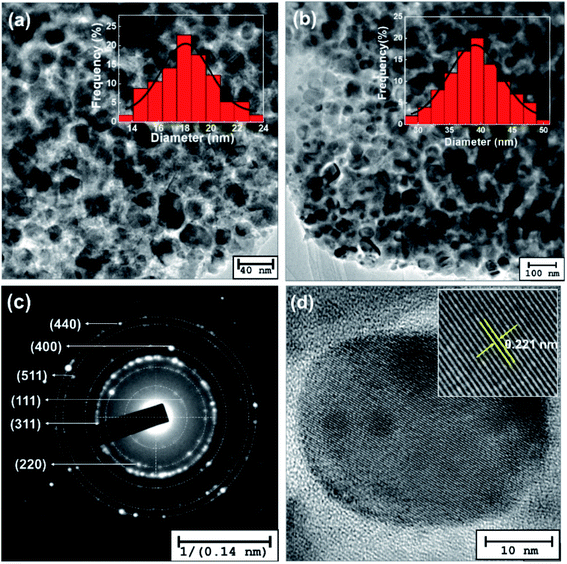 |
| Fig. 4 TEM images of the as-synthesized Co1−xCuFe2O4/SiO2 with (a) x = 0 and (b) x = 0.2. (c) SAED pattern and (d) HRTEM image for x = 0.2 sample. Insets in panel (a) and (b) show the average particle size distribution obtained by approximate 50 nanoparticles, respectively. | |
3.2 Mössbauer spectroscopy
Mössbauer technique serves as one of the most powerful tools for probing the atomic and electronic configuration of Fe atoms, thus, the hyperfine interaction of Co1−xCuFe2O4 was investigated through Mössbauer spectra. Fig. 5 shows the experimental Mössbauer spectra and fitting lines of Co1−xCuFe2O4/SiO2 with different Cu2+ doping contents, and Table 1 presents the correspondingly fitting parameters. These spectra are decomposed into two Zeeman sextets, demonstrating that Co1−xCuxFe2O4 NPs in the obtained samples are ferromagnetically ordered. The values of isomer shifts (IS) are in the range of 0.26–0.40 mm s−1, suggesting that Fe ions in the present Co1−xCuxFe2O4 NPs are in high spin Fe3+ charge state. Among two sextets, one with smaller IS and hyperfine field (Hin) arises from the tetrahedral Fe3+ ions, and the other with larger IS and Hin can be ascribed to the octahedral Fe3+ ions. It is well known that the value of IS is dependent on s-electron density of Fe3+ nucleus. Owing to the larger bond length of Fe3+–O2− at octahedral B sites, the orbital overlapping of Fe3+ and O2− is smaller, hence the IS at octahedral B sites is larger than that of tetrahedral A sites. With increasing Cu2+ doping content, the ISA value decreases while the ISB increases, suggesting that the Cu2+ doping behavior can affect the s-electron distribution of Fe3+ ions at tetrahedral A and octahedral B sites due to Jahn–Teller effect of Cu2+ ions.27
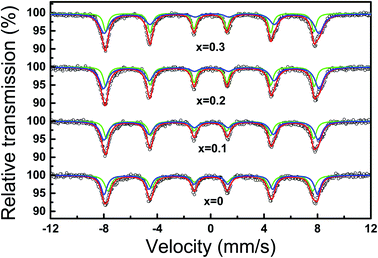 |
| Fig. 5 Mössbauer spectra of Co1−xCuxFe2O4/SiO2 samples. Symbols represent the experimental data and the continuous line corresponds to the fitting data. | |
Table 1 Mössbauer parameters of Co1−xCuxFe2O4/SiO2 samplesa
Sample |
Component |
IS (mm s−1) |
QS (mm s−1) |
Hin (T) |
FWHM (mm s−1) |
S (%) |
SA/SB |
IS = isomer shift; QS = quadruple split, Hin = hyperfine field, S = relative absorption area, FWHM = the half width at half maximum. |
x = 0 |
Sextet (A) |
0.300 ± 0.004 |
0.027 ± 0.008 |
47.6 ± 1.1 |
0.296 ± 0.012 |
46.7 |
0.876 |
Sextet (B) |
0.324 ± 0.003 |
0.007 ± 0.002 |
49.6 ± 0.9 |
0.279 ± 0.011 |
53.3 |
x = 0.1 |
Sextet (A) |
0.292 ± 0.003 |
0.022 ± 0.004 |
48.0 ± 0.8 |
0.248 ± 0.010 |
44.2 |
0.792 |
Sextet (B) |
0.346 ± 0.007 |
0.014 ± 0.003 |
49.8 ± 0.8 |
0.326 ± 0.021 |
55.8 |
x = 0.2 |
Sextet (A) |
0.280 ± 0.005 |
0.037 ± 0.001 |
48.4 ± 0.7 |
0.222 ± 0.007 |
43.8 |
0.779 |
Sextet (B) |
0.369 ± 0.010 |
0.023 ± 0.003 |
50.0 ± 0.8 |
0.383 ± 0.011 |
56.2 |
x = 0.3 |
Sextet (A) |
0.269 ± 0.006 |
0.039 ± 0.002 |
48.4 ± 0.6 |
0.217 ± 0.013 |
43.7 |
0.776 |
Sextet (B) |
0.399 ± 0.011 |
0.039 ± 0.003 |
49.8 ± 0.9 |
0.434 ± 0.011 |
56.3 |
Among Mössbauer parameters, quadrupole splitting (QS) is related to the crystal symmetry. As seen from Table 1, the value of QSB gradually increases with Cu2+ content, while the values of QSA do not exhibit a specific tendency. This phenomenon reveals that the local symmetry of octahedral B site Fe3+ ions is modified during Cu2+ doping process, suggesting that the Cu2+ ions preferentially occupied octahedral B sites in the as-prepared Co–Cu ferrites. Owing to Jahn–Teller effect of Cu2+ ions at octahedral B sites, they form dsp2 orbital hybridization and produce strain in Co1−xCuxFe2O4 crystals, inducing the octahedral symmetry to deform slightly without disrupting the lattice symmetry.28
As a consequence, hypothesizing that all Cu2+ ions locate at octahedral B sites, it is possible to give an estimate of cation distribution for Co1−xCuxFe2O4 NPs as (CuσFe1−σ)A[Co1−x−σCuxFe1+σ]B, where x is Cu2+ content and the value of σ can be determined by:
|
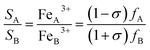 | (1) |
Here, assuming the recoilless fraction
fA and
fB to be same, the relative area ratio
SA/
SB thus directly corresponds to the ratio of the number of Fe
3+ ions at tetrahedral A and octahedral B sites.
27 Based on the Mössbauer fitting data, the ratio
SA/
SB for the
x = 0 sample is 0.876, thus the cation distribution can be written as (Co
0.066Fe
0.934)
A [Co
0.934Fe
1.066]
B, that is to say, 93.4% of Co
2+ ions resides at octahedral B sites. Sawatzky
et al.29 reported that the ratio of octahedral Co
2+ ions in CoFe
2O
4 depended on the heat treatment. They estimated that 96% and 79% of Co
2+ ions presented in the slowly cooled and quenched CoFe
2O
4 NPs, respectively. When Cu
2+ ions is doped in CoFe
2O
4, the ratio of
SA/
SB for
x = 0.1 sample becomes 0.792. The cation distribution is represented as (Co
0.116Fe
0.884)
A[Co
0.784Cu
0.1Fe
1.116]
B, demonstrating that Cu
2+ doping results in the relocation of small amount of Co
2+ from B to A sites concomitantly with some Fe
3+ ions migrated from A to B sites, although Cu
2+ ions locate at the octahedral B sites. Further increasing Cu
2+ content to 0.2 and 0.3, it is found that the concentration of Fe
3+ ion in A and B sites almost unchanged (
SA/
SB = 0.779 for
x = 0.2 and 0.776 for
x = 0.3 sample), revealing that Cu
2+ ions only replace octahedral Co
2+ ions, and make no effect on Fe
3+ distribution. The cation distribution can be written as (Co
0.124Fe
0.876)
A [Co
0.676Cu
0.2Fe
1.124]
B for the sample with
x = 0.2, and (Co
0.126Fe
0.874)
A [Co
0.574Cu
0.3Fe
1.126]
B for the sample with
x = 0.3.
From the 7th column of Table 1, we find that the half width at half maximum (FWHM) of A and B lines varies with Cu2+ content. In cubic spinel lattice, each A-site Fe3+ ion is surrounded by 12 nearest B-site ions neighbors and each B-site Fe3+ ion is surrounded by 6 nearest A-site ions neighbors, thus B-site Fe3+ is more sensitive to the change in the surrounding cation distribution than the A-site Fe3+ ions. According to the cation distribution, for the sample with x = 0, each Fe3+ ion in A and B sites is surrounded by approximately 6 nearest Fe3+ ions, therefore, the line width is comparable but relatively narrow. When Cu2+ ions are doped in CoFe2O4 lattice, some Fe3+ ions migrate from A to B sites, hence the A site Fe3+ ions get more nearest FeB3+ neighbors. This leads to a reduction in the total super-exchange strength of B-site Fe3+ ions while an increase in A-site Fe3+ ions.30 Consequently, broadened B line and narrowed A line are observed in Co–Cu ferrite. In addition, Table 1 also presents the same increasing trend of the hyperfine field (Hin) for tetrahedral A and octahedral B sites with increasing Cu2+ content. The weighted average values of Hin are 48.7, 49.0, 49.3, and 49.2 T for the Co1−xCuxFe2O4 with x = 0, 0.1, 0.2, and 0.3, respectively. The increase in Hin can be attributed to the increasing crystallite size, since the fluctuation of magnetization vectors close to easy direction of magnetization can give rise to a size dependent magnetic hyperfine field.31
3.3 Magnetic properties analysis
Fig. 6a shows the magnetic hysteresis loops of Co1−xCuxFe2O4/SiO2 samples measured at room temperature. Clearly, these loops show the typical characteristics of ferromagnetic materials. At the applied field intensity (15 kOe), saturation state cannot be reached yet, thus the saturation magnetization Ms was estimated by fitting the high-field part of the magnetization curves using the relation
here H is the field strength, a and b are constant determined by the fitting procedure.32 The fitted Ms and the measured coercivity Hc are plotted as functions of Cu2+ content x in Fig. 6b. The Ms for the pure CoFe2O4 is 24.7 emu g−1, which is close to the reported value for 10–15 nm CoFe2O4/SiO2 (30–50% SiO2) prepared sol–gel method.19 The low Ms for pure CoFe2O4 sample can be attributed to the existence of amorphous SiO2 matrix, which modifies the magnetic behavior through minimizing the particle interactions between ferrite particles.33,34 The value of Ms first increases to 34.3 emu g−1 when Cu2+ content is 0.1, and then reduces to 27.1 emu g−1 as Cu2+ content further increases to 0.3. Two factors are possibly responsible for the higher Ms values for Cu-doping CoFe2O4 comparing with pure CoFe2O4. For x = 0.1 sample, the Mössbauer analysis indicates that doping Cu2+ ions with magnetic moment 1 μB results in the migration of Fe3+ ions from tetrahedral A to octahedral B sites. This behavior leads to the magnetization of the octahedral B sites and hence the Ms increases.35 For the samples with x = 0.2 and 0.3, more Cu2+ ions occupied B-sites decreases the B-sublattice magnetization, thereby the enhanced Ms can be attributed to the increasing crystallite sizes with Cu2+ content. Noted that the Ms of 34.3 emu g−1 for the x = 0.1 sample is about 38.9% larger than pure CoFe2O4.
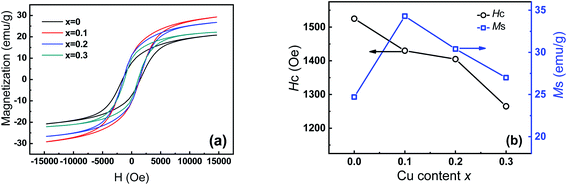 |
| Fig. 6 (a) Hysteresis loops of Co1−xCuxFe2O4/SiO2, (b) plot of Ms and Hc of samples as a function of Cu2+ content. | |
Considering the Neel' two sub-lattice collinear model of ferrimagnetism, the magnetic moment ηNeelB per unit formula in Bohr magneton can be estimated by ηNeelB = MB(x) − MA(x).36 Assuming the magnetic moment of Fe3+, Co2+ and Cu2+ to be 5, 3 and 1 μB, respectively, then using the obtained cation distribution from Mössbauer analysis, the magnetic moments ηNeelB are calculated and summarized in Table 2. Meanwhile, Table 2 also provides the magnetic moment ηobsB determined by the fitted Ms using the following formula:37 ηobsB = (Mw × Ms)/5585, where Mw is the molecular weight of the ferrite. As Table 2 indicates, the calculated values of ηobsB are smaller than that of ηNeelB, which suggests Neel's collinear model is not suitable for the obtained samples. Moreover, there is a significant canted spin arrangement in B-sites, which enhances the B–B interaction and in turn decreases the A–B interaction. According to the Yafet and Kittel’s three sublattice model, the spin-canting angle θYK (Yafet–Kittle angle) is calculated by:38
|
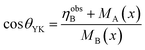 | (2) |
Table 2 Magnetic parameters of Co1−xCuxFe2O4/SiO2 at room temperature
Sample |
Ms (emu g−1) |
Mr (emu g−1) |
Hc (Oe) |
ηobsB (μB) |
ηNeelB (μB) |
θYK (degree) |
x = 0 |
24.7 |
7.6 |
1525 |
1.48 |
3.26 |
38.6 |
x = 0.1 |
34.3 |
11.1 |
1430 |
2.06 |
3.37 |
33.6 |
X = 0.2 |
30.4 |
10.8 |
1405 |
1.83 |
3.10 |
33.1 |
X = 0.3 |
27.1 |
9.35 |
1265 |
1.63 |
2.91 |
33.4 |
The results are given in Table 2. It should be noted that the values of θYK is 38.6° for x = 0.1 sample, comparable to the reported value for CoFe2O4/SiO2 with 30% silica in ref. 39. However, the θYK decreases to ∼33° for Cu-doping ferrites (Table 2), which indicates the presence of Cu2+ ions at B sites reduces the degree of spin canting. Using high field Mössbauer spectra, Peddis et al.39,40 confirmed that the spin canting mainly located in the octahedral B sites. Owing to the high anisotropy energy of Co2+ ions,41 the non-collinear canting spin mainly occurs in B-site Fe3+ magnetic moment.42,43 The similar θYK values observed in as-prepared Co1−xCuxFe2O4 with 18–36 nm sizes indicate that the spin canting is not a surface phenomenon but an effect throughout the volume of the particles, including surface spin and core spin.41
On the other hand, the coercivity Hc decreases continuously from 1525 to 1265 Oe as Cu2+ doping content increases from 0 to 0.3. The change in Hc with Cu2+ content may be related to crystallite size, cation distribution, and magneto crystalline anisotropy constant. It is well known that the Hc of magnetic particle with single domain should increase with crystallite size in principle. In the present case, the average crystallite sizes of Co1−xCuxFe2O4 NPs are lower than the single domain critical size (40 nm) of CoFe2O4 NPs. Therefore, the decrease in Hc should be attributed to the cation distribution and magneto-crystalline anisotropy constant. Since Co2+ ion at octahedral B site has larger anisotropy (+850 × 10−24 J per ion) than that at tetrahedral A site (−79×10−24 J per ion),44 the octahedral Co2+ ions can be responsible for the high magneto-crystalline anisotropy of CoFe2O4.45,46 The replacement of octahedral Co2+ by Cu2+ ion results in the reduction in the percentage of Co2+ in B sites, and thus decreases the anisotropy constant.
4. Conclusions
To summarize, we have studied the effect of Cu2+ doping content on the microstructural and magnetic properties of Co1−xCuxFe2O4/SiO2 (0 ≤ x ≤ 0.3) nanocomposites. Although all the obtained Co1−xCuxFe2O4 NPs have cubic spinel structure, the substitution of Cu2+ for Co2+ ions can bring change in the crystallite size, cation distribution, and magnetic properties. The crystallite size increases with Cu2+ doping content. The preferred occupancy of Cu2+ ions at octahedral B sites results in slight deformation of octahedral symmetry and Fe3+ ions migration from tetrahedral A to octahedral B sites. Moreover, the values of Ms and Hc are strongly dependent on Cu2+ doping content, which can be attributed to the cation migration between both sublattices (A and B). The relatively large spin-canting angle θYK reveals that the spin canting mainly occurs in the octahedral Fe3+ throughout the particles. The results suggest that the Cu2+ doping content in Co1−xCuxFe2O4 NPs can play an important role in tuning their physical properties, which may be of great significance in to exploit novel applications in high density information storage, electronic devices and biomedicine.
Conflicts of interest
There are no conflicts to declare.
Acknowledgements
This work was supported by the National Natural Science Foundation of China (Nos. 21371071), Foundation of Science and Technology of Jilin, China (Grant No. 201205075).
References
- Z. T. Zhang, A. J. Rondinone, J. X. Ma, J. Shen and S. Dai, Morphologically Templated Growth of Aligned Spinel CoFe2O4 Nanorods, Adv. Mater., 2005, 17(11), 1415–1419 CrossRef CAS.
- L. Demir, U. Perisanoglu and M. Sahin, Investigating XRF parameters and valance electronic structure of the Co, Ni, and Cu spinel ferrite, Ceram. Int., 2019, 45, 7748–7753 CrossRef CAS.
- M. Zhang,F, Z. ,Y. Yang, T. An, W. Qu, H. Li, J. Zhang and N. Li, Catalytic activity of ferrates (NiFe2O4, ZnFe2O4 and CoFe2O4) on the thermal decomposition of ammonium perchlorate, Propell., Explos., Pyrot., 2020, 45(3), 463–471 CrossRef.
- M. Sundararajan and L. J. Kennedy, Photocatalytic removal of rhodamine B under irradiation of visible light using Co1−xCuxFe2O4 (0≤x≤0.5) nanoparticles, J. Environ. Chem. Eng., 2017, 5(4), 4075–4092 CrossRef CAS.
- B. Li, H. T. Fan, X. Xing, Y. Yang, C. C. Wang and D. F. Qiu, Triple functions nanocomposites of porous silica-CoFe2O4-MWCNTs as carrier for pH-sensitive anti-cancer drugs controlled delivery, Dalton Trans., 2017, 46, 14831–14838 RSC.
- J. Yang, Y. Chen, Y. H. Li and X. B. Yin, Magnetic resonance imaging-guided multi-drug chemotherapy and photothermal synergistic therapy with ph and nir-stimulation release, ACS Appl. Mater. Interfaces, 2017, 9(27), 22278–22288 CrossRef CAS PubMed.
- T. Walther, U. Straube, R. Koferstein and S. G. Ebbinghaus, Hysteretic magnetoelectric behavior of CoFe2O4-BaTiO3 composites prepared by reductive sintering and reoxidation, J. Mater. Chem. C, 2016, 4, 4792–4799 RSC.
- C. Venkateshwarlu and D. Ravinder, Thermoelectric power studies of Cu-Co ferrites, J. Alloy Compd., 2006, 426, 4–6 CrossRef CAS.
- T. Tatarchuk, M. Bououdina, W. Macyk, O. Shyichuk, N. Paliychuk, I. Yaremiy, B. Al-Najar and M. Pacia, Structural, optical, and magnetic properties of Zn-doped CoFe2O4 nanoparticles, Nanoscale Res. Lett., 2017, 12(1–11), 141 CrossRef PubMed.
- G. Xi and Y. Xi, Effects on magnetic properties of different metal ions substitution cobalt ferrites synthesis by sol–gel auto-combustion route using used batteries, Mater. Lett., 2016, 164, 444–448 CrossRef.
- N. Sanpo, C. C. Berndt, C. Wen and J. Wang, Transition metal-substituted cobalt ferrite nanoparticles for biomedical applications, Acta Biomater., 2013, 9(3), 5830–5837 CrossRef CAS PubMed.
- J. Balavijayalakshmi, N. Suriyanarayanan and R. Jayapraksah, Influence of copper on the magnetic properties of cobalt ferrite nanoparticles, Mater. Lett., 2012, 81, 52–54 CrossRef CAS.
- R. A. Mecurrie, Ferromagnetic material structure and properties, Academic Press, London, 1994 Search PubMed.
- M. Kucera and P. Brom, Magneto-optical properties of nano-crystalline cubic and tetragonal copper ferrite thin films, J. Appl. Phys., 2018, 117, 17B738 CrossRef.
- T. Muthukumaran and J. Philip, Synthesis of water dispersible phosphate capped CoFe2O4 nanoparticles and its applications in efficient organic dye removal, Colloid. Surface. A, 2021, 610, 125755 CrossRef CAS.
- T. Muthukumaran and J. Philip, A facile approach to synthesis of cobalt ferrite nanoparticles with a uniform ultrathin layer of silicon carbide for organic dye removal, J. Mol. Liq., 2020, 317(1–14), 114110 CrossRef CAS.
- M. Gharagozlou, B. Ramezanzadeh and Z. Baradaran, Synthesize and characterization of a novel anticorrosive cobalt ferrite nanoparticles dispersed in silica matrix (CoFe2O4-SiO2) to improve the corrosion protection performance of epoxy coating, Appl. Surf. Sci., 2016, 377, 86–98 CrossRef CAS.
- Z. S. Piskuła, P. Skokowski, T. Tolinski, M. Zielinski, P. Kirszensztejn and W. Nowicki, Structure, magnetic and catalytic properties of SiO2-MFe2O4 (M =Mn, Co, Ni, Cu) nanocomposites and their syntheses by a modified sol–gel method, Mater. Chem. Phys., 2019, 235, 121731 CrossRef.
- T. Dippong, O. Cadar, E. A. Levei, I. Bibicu, L. Diamandescu, C. Leostean, M. Lazar, G. Borodi and L. B. Tudoran, Structure and magnetic properties of CoFe2O4/SiO2 nanocomposites obtained by sol–gel and post annealing pathways, Ceram. Int., 2017, 43, 2113–2122 CrossRef CAS.
- J. Hua, Y. Liu, L. Wang, M. Feng, J. Zhao and H. Li, Mössbauer studies on Mn substituted CoFe2O4/SiO2 nanocomposites synthesized by sol–gel method, J. Magn. Magn. Mater., 2016, 402, 166–171 CrossRef CAS.
- K. Nadeem, T. Traussnig, I. Letofsky-Papst, H. Krenn and U. Brossmann, Sol–gel synthesis and characterization of single-phase Ni ferrite nanoparticles dispersed in SiO2 matrix, J. Alloy. Compd., 2010, 493, 385–390 CrossRef CAS.
- S. Rohilla, S. Kumar, P. Aghamkar, S. Sunder and A. Agarwal, Investigations on structural and magnetic properties of cobalt ferrite/silica nanocomposites prepared by the coprecipitation method, J. Magn. Magn. Mater., 2011, 323, 897–902 CrossRef CAS.
- Y. Tang, X. Wang, Q. Zhang, Y. Li and H. Wang, Solvothermal synthesis of Co1−xNixFe2O4 and its application in ammonia vapors detection Progress in natural science, Mater. Inter., 2012, 22, 53–58 Search PubMed.
- C. C. Naik, S. K. Gaonkar, I. Furtado and A. V. Salker, Effect of Cu2+ substitution on structural, magnetic and dielectric properties of cobalt ferrite with its enhanced antimicrobial property, J. Mater. Sci-Mater. El., 2018, 29, 14746–14761 CrossRef CAS.
- A. H. Ashour, A. I. El-Batal, M. I. A. Abde Maksoud, G. S. El-Sayyad, S. Labib, E. Abdeltwab and M. M. El-Okr, Antimicrobial activity of metal-substituted cobalt ferrite nanoparticles synthesized by sol–gel technique, Particuology, 2018, 40, 141–151 CrossRef CAS.
- T. Dipponga, I. G. Deacb, O. Cadarc, E. A. Leveic and I. Peteand, Impact of Cu2+ substitution by Co2+ on the structural and magnetic properties of CuFe2O4 synthesized by sol–gel route, Mater. Charact., 2020, 163, 110248 CrossRef.
- B. K. Chatterjee, K. Bhattacharjee, A. Dey, C. K. Ghosha and K. K. Chattopadhyay, Influence of spherical assembly of copper ferrite nanoparticles on magnetic properties: orientation of magnetic easy axis, Dalton Trans., 2014, 43, 7930–7944 RSC.
- A. B. Naik, S. R. Sawant, S. A. Patil and J. I. Powar, On the variation of a.c. susceptibility with temperature for some Cu-Li ferrites,Bull, Mater. Sci., 1988, 11(4), 315–318 CAS.
- G. A. Sawatzky, F. V. D. Woude and A. H. Morrish, Cation distributions in octahedral and tetrahedral sites of the ferromagnetic spinel CoFe2O4, J. Appl. Phys., 1968, 39, 1204–1205 CrossRef CAS.
- G. A. Sawatzkyt, F. D. W. Van and A. H. Morrish, Mössbauer study of several ferrimagnetic spinels, Phys. Rev., 1969, 187(2), 747–757 CrossRef.
- K. M. Batoo, D. Salah, G. Kumar, A. Kumar, M. Singh, M. A. El-sadek, F. A. Mir, A. Imran and D. A. Jameel, Hyperfine interaction and tuning of magnetic anisotropy of Cu doped CoFe2O4 ferrite nanoparticles, J. Magn. Magn. Mater., 2016, 411, 91–97 CrossRef CAS.
- A. H. Morrish, The physical principles of magnetism, Wiley, New York, 1965 Search PubMed.
- V. Blanco-Gutiérrez, M. Virumbrales, R. Saez-Puche and M. J. Torralvo-Fernández, Superparamagnetic Behavior of MFe2O4 Nanoparticles and MFe2O4/SiO2 Composites (M: Co, Ni), J. Phys. Chem. C, 2013, 117(40), 20927–20935 CrossRef.
- M. Virumbrales, R. Saez-Puche, M. J. Torralvo and V. Blanco-Gutierrez, Mesoporous
silica matrix as a tool for minimizing dipolar interactions in NiFe2O4 and ZnFe2O4 nanoparticles, Nanomaterials, 2017, 7, 151 CrossRef PubMed.
- M. Hashim, Alimuddin, S. Kumar, B. H. Koo, S. E. Shirsath, E. M. Mohammed, J. Shah, R. K. Kotnala, H. K. Choi, H. Chung and R. Kumar, Structural, electric and magnetic properties of Co-Cu ferrite nanoparticles, J. Alloy. Compd., 2012, 518, 11–18 CrossRef CAS.
- L. Néel, Magnetic properties of ferrites: ferrimagnetism and antiferromagnetism, Ann. Phys. Paris., 1948, 137–198 CrossRef.
- B. G. Toksha, S. E. Shirsath, M. L. Mane, S. M. Patange, S. S. Jadhav and K. M. Jadhav, Autocombustion high-temperature synthesis, structural, and magnetic properties of CoCrxFe2−xO4 (0 ≤ x ≤ 1.0), J. Phys. Chem. C, 2011, 115, 20905–20912 CrossRef CAS.
- Y. Yafet and C. Kittel, Antiferromagnetic arrangements in ferrites, Phys. Rev., 1952, 87, 290 CrossRef CAS.
- D. Peddis, M. V. Mansilla, S. Morup, C. Cannas, A. Musinu, G. Piccaluga, F. Orazio, F. Lucari and D. Fiorani, Spin-canting and magnetic anisotropy in ultrasmall CoFe2O4 nanoparticles, J. Phys. Chem. B, 2008, 112, 8507 CrossRef CAS PubMed.
- D. Peddis, C. Cannas, G. Piccaluga, E. Agostinelli and D. Fiorani, Spin-glass-like freezing and enhanced magnetization in ultra-small CoFe2O4 nanoparticles, Nanotechnology, 2010, 21(1–10), 125705 CrossRef CAS PubMed.
- C. Cannas, A. Musinu, G. Piccaluga, D. Fiorani, D. Peddis, H. K. Rasmussen and S. Mørup, Magnetic properties of cobalt ferrite-silica nanocomposites prepared by a sol–gel autocombustion technique, J. Chem. Phys., 2006, 125(535), 164714 CrossRef CAS PubMed.
- C. Bellitto, E. M. Bauer and G. Righini, On the crystalstructures and magnetism of some hybrid organic–inorganic metal organophosphonates, Inorg. Chim. Acta, 2008, 361, 3785–3799 CrossRef CAS.
- D. Peddis, N. Yaacoub, M. Ferretti, A. Martinelli, G. Piccaluga, A. Musinu, C. Cannas, G. Navarra, J. M. Greneche and D. Fiorani, Cationic distribution and spin canting in CoFe2O4 nanoparticles, J. Phys.: Condens. Matter, 2011, 23, 426004 CrossRef CAS PubMed.
- H. Y. Zhang, B. X. Gu, H. R. Zhai, Y. Z. Miao, S. Y. Zhang and H. B. Huang, Anisotropy and Faraday effect in Co spinel ferrite films, J. Appl. Phys., 1994, 75, 7099–7101 CrossRef CAS.
- J. F. Hochepied, Ph. Sainctavit and M. P. Pileni, X-ray absorption spectra and X-ray magnetic circular dichroism studies at Fe and Co L2,3 edges of mixed cobalt-zinc ferrite nanoparticles: cationic repartition, magnetic structure and hysteresis cycles, J. Magn. Magn. Mater., 2001, 231, 315–322 CrossRef CAS.
- A. A. Ati, Z. Othaman and A. Samavati, Influence of cobalt on structural and magnetic properties of nickel ferrite nanoparticles, J. Mol. Struct., 2013, 1052, 177–182 CrossRef CAS.
|
This journal is © The Royal Society of Chemistry 2021 |