DOI:
10.1039/D1RA04371D
(Paper)
RSC Adv., 2021,
11, 21813-21823
Quick extracellular biosynthesis of low-cadmium ZnxCd1−xS quantum dots with full-visible-region tuneable high fluorescence and its application potential assessment in cell imaging
Received
6th June 2021
, Accepted 7th June 2021
First published on 21st June 2021
Abstract
The biosynthesis of metal nanoparticles/QDs has been universally recognized as environmentally sound and energy-saving, generating less pollution and having good biocompatibility, which is most needed in biological and medical fields. In the arena of chemical routes, however, biosynthesis has long been criticized for its low productivity, time-consuming process, and poor control over size, shape and crystallinity, keeping the much-needed technology away from practical application. In this work, a rapid and extracellular biosynthesis of multi-colour ternary ZnxCd1−xS QDs by a mixed sulfate-reducing bacteria (SRB)-derived supernatant was carried out for the first time to solve the problems plaguing this field of biosynthesis. The results showed that about 3.5 g L−1 of ZnxCd1−xS QDs with size of 3.50–4.64 nm were achieved within 30 minutes. The PL emission wavelength of ZnxCd1−xS QDs increased from 450 to 590 nm to yield multicolor QDs by altering the molar ratio of Cd2+ to Zn2+. The SRB-biogenic ZnxCd1−xS QDs have high stability in gastric acid and at high temperature, as well as excellent biocompatibility and biosafety, successfully entering growing HeLa cells and labelling them without detectable harm to cells. The SRB-secreted peculiar extracellular proteins (EPs) play a decisive function in the time-saving, high-yield biosynthesis of PL-tuned multicolor QDs, which cover an abnormally high concentration of acidic amino acids to provide tremendous negatively charged sites for the absorption of Cd2+/Zn2+ for rapid nucleation and biosynthesis. The strongly electrostatic connection between the QDs and the EPs and the increasing amount of EPs attached to the QDs in response to the increase of Cd2+ concentration account for their high stability and excellent biocompatibility.
1 Introduction
Quantum dots (QDs) are inorganic semiconductor nanocrystals with a size range of 2–10 nm. They are composed of elements such as Cd, Pb, and Hg from groups II–VI, III–IV and IV–VI of the periodic table.1 Because the particle size of QDs is smaller than the bulk materials' Bohr radius, the energy levels pose discrete electronic energy states compared to the energy level continuum in bulk materials.2 Owing to the quantum confinement effects and size-dependent photoemission characteristics, QDs are known for being bright, color-tunable inorganic fluorophores with narrow symmetric emission bands and high photostability.3 Due to the unique photooptical and photovoltaic properties, QDs are broadly used in solar cells, photovoltaic devices, light-emitting diode (LED) fabrication, photodetectors and computing; particularly, they are well suited for application in the fields of biology and medicine for imaging, sensing and tracking particles or cells, including biolabeling and biosensing, as substitutes for organic fluorophores.2–4
At present, wet chemical methods such as micro-emulsion, co-precipitation, sol gel, solvothermal, ultrasonic irradiation and microwave heating are the mainstream routes to commercially fabricate a variety of nanoparticles and QDs.3,5 The chemical strategies provide excellent control over the shape and size of the nanoparticles and QDs, which is vitally important, as these parameters dominate their nanoscale properties.6 Although the solution-based wet routes have typically governed the nanosphere, increasing concerns have arisen with regard to the negative impact on the environment owing to the use of toxic chemicals/solvents, explosive precursors, harsh reaction conditions such as extreme temperature, pressure and pH, as well as the discharge of harmful byproducts.5,7–9 Therefore, there is an urgent need to develop green and environmentally friendly methods to synthesize nanoparticles and QDs as a viable alternative to the chemical synthesis routes.3,5 Moreover, the chemically manufactured nanoparticles and QDs are usually inadequate for biological and medical systems due to poor biocompatibility.10,11
Biological synthesis follows the green chemistry principle, which is more acceptable in terms of environment friendliness, energy savings, the use of ambient temperatures and pressures, the use of renewable materials as electron donors, and the production of biocompatible matter.3,10 A wide range of metal nanoparticles and QDs have therefore been synthesized by diverse microorganisms and biomolecules in the past 20 years.5,12 However, microbial biosynthesis of metal nanoparticles and QDs suffers from some disadvantages, which severely block its commercial application. The main shortcoming is poor control of size, morphology, structure, property, and crystallinity, along with difficult separation of the products from microbes. Another major drawback is the slow production rate compared to chemical synthesis routes, making the biological production process more time consuming.3,13 Nevertheless, in recent years, these problems have gradually been solved through developing extracellular biosynthesis processes, employing sulfate-reducing bacteria (SRB), changing incubation time and altering metal concentration to ease the separation process, increase the production efficiency, adjust the fluorescence spectra and control the size of nanoparticles, respectively.14–17
Among various semiconductor metal nanomaterials, the biosynthesis of metal sulfides has long been the most appealing research topic because of the abundant sulfur on Earth as well as the unique optical and electronic properties and wide applications of metal sulfides.3,10,18,19 In particular, the bio-manufacture of ZnS and CdS QDs has drawn considerable attention for applications in the fields of biology and medicine for imaging, sensing, tracking and drug delivery, owing to their high optical efficiency, high fluorescence intensity and low cost.3,10,12 ZnS is a wide-bandgap photoluminescent material with low toxicity and high photocorrosion resistance, but it has poor absorption over the visible light range,20 while CdS has a narrow bandgap with strong absorption in the visible light range, but it is highly toxic and has poor photocorrosion resistance.21,22 For zincblende ZnS and CdS, their lattice constants under ambient temperature are, respectively, 5.406 Å and 5.835 Å. Such a low lattice mismatch indicates that introducing Zn into the CdS lattice to form ZnxCd1−xS alloyed QDs may be a practical way to engineer emitters with both high bandgap emission efficiency and reduced toxicity,23–25 thus covering the shortage of single ZnS and CdS.26
As a ternary semiconductor, ZnxCd1−xS alloyed QDs possess a continuous, adjustable bandgap, high carrier mobility and high quantum yield,27 thus displaying wider potential applications than the discrete-bandgap binary materials ZnS and CdS QDs in phosphorescent differential sensing,28 biological markers,29 glucose sensing30 and immunosorbent assay.31 Especially, ZnxCd1−xS alloyed QDs open up a great possibility for in vitro and in vivo imaging, labeling and sensing in medicine and life science due to their tunable fluorescence characteristics.32 For more competent ZnxCd1−xS QDs in the fields of biology and medicine, the mainstream chemical synthesis methods have been concentrating on both tunable fluorescence and toxicity reduction.33–35 Tunable fluorescence is attained by increasing the cadmium content to realize fluorescence red-shift and form multicolor QDs, while toxicity reduction is achieved through the formation of a passivation layer on the surface of ZnxCd1−xS QDs using highly biocompatible molecules.36 However, the elevated Cd content inevitably leads to increased toxicity of the ZnxCd1−xS QDs,35 while the formation of a passivation layer using expensive biocompatible molecules increases production cost.34 Low-cost synthesis of ZnxCd1−xS alloyed QDs with low Cd content and red shift to full-visible-region tunable high fluorescence is of importance for applications in medicine and life sciences.
A great number of studies have focused on the biosynthesis of metal sulfides ZnS and CdS QDs as binary semiconductors, but no biosynthesis of ternary semiconductor ZnxCd1−xS alloyed QDs has been available, to date. In previous work, the highest ZnS QD extracellular biosynthesis rate of 35.0–45.0 g per L per month was obtained by mixed sulfate-reducing bacteria (SRB), which secreted peculiar extracellular proteins (EPs) containing extraordinary amino acids to strongly mediate the biosynthesis of binary metal sulfide QDs.15 In the current work, the mixed SRB was utilized for extracellular biosynthesis of low-toxicity and adjustable-fluorescence ternary semiconductor ZnxCd1-xS alloyed QDs for the first time. The ZnxCd1-xS QDs, with low Cd content, from 0.2 to 0.02 in molar ratio of Cd/Zn, and a tunable fluorescence from 450 nm to 590 nm, were biosynthesized at a high yield, and the high biocompatibility, high stability and low toxicity of the resulting ZnxCd1−xS QDs were assessed completely.
2 Results and discussion
2.1 Biosynthesis of ZnxCd1−xS QDs with full-visible-region tunable PL
2.1.1 Phase analysis of the resulting products by XRD and EDX. The XRD images displayed three major characteristic diffraction peaks (111), (220) and (311) in the as-prepared products from Zn2+/Cd2+ = 0
:
1 and Zn2+/Cd2+ = 1
:
0, which are well indexed to the cubic sphalerite structure of CdS (JCPDS Card No. 65-2887) and ZnS (JCPDS Card No. 65-5476), respectively (Fig. 1). This showed the SRB-derived supernatant is capable of biosynthesizing both CdS and ZnS as controls. The resulting products from different molar ratios of Cd2+ to Zn2+ of 1
:
50 to 1
:
5 also showed three major characteristic diffraction peaks, respectively symbolizing lattice planes (111), (220) and (311), indicating a quick synthesis of ternary ZnxCd1−xS by the SRB-derived supernatant. Compared to the hollow ZnS and CdS, the XRD peak position of the (111) plane of Zn1−xCdxS was slightly shifted to lower diffraction angles (2θ) from 29.2 to 28.6, 28.5, 28.3, 28.1, 27.8, 27.4, 26.8 and 26.6, respectively, as the concentrations of Cd gradually increased (Fig. 1). No impurity peaks occurred in the XRD patterns of Zn1−xCdxS, displaying the high purity of the biosynthesized Zn1−xCdxS crystals. The XRD characteristics of ZnxCd1−xS obtained in this study are completely consistent with those obtained in previous literature.24,35 The EDX images further revealed that the actual molar ratios of Zn2+ to Cd2+ in the biosynthesized Zn1−xCdxS were very close to that in the mixed solutions of Zn2+ and Cd2+, and that total molar concentrations of Zn2+ and Cd2+ were almost equal to that of the sulfur moiety (S2−), accounting for the high purity of the crystal materials (Fig. 2). Elements C, O and P were detected because of the existence of EPs, which adhered to the as-prepared products.15 As a result, the structural similarity between ZnS and CdS and relatively small discrepancy in their bond lengths facilitate the biosynthesis of ZnxCd1−xS.37
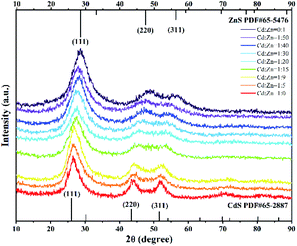 |
| Fig. 1 XRD patterns of the resulting products biosynthesized by the SRB-derived supernatant at different molar ratios of Cd2+ and Zn2+ ranging from 1 : 50 to 1 : 5. The ratio of Cd2+/Zn2+ at 1 : 0 and Cd2+/Zn2+ at 0 : 1 served as controls for CdS and ZnS, respectively. The two sets of lines on the X-axis (JCPDS No. 65-5476 and JCPDS No. 65-2887) are the standard spectra for ZnS and CdS. | |
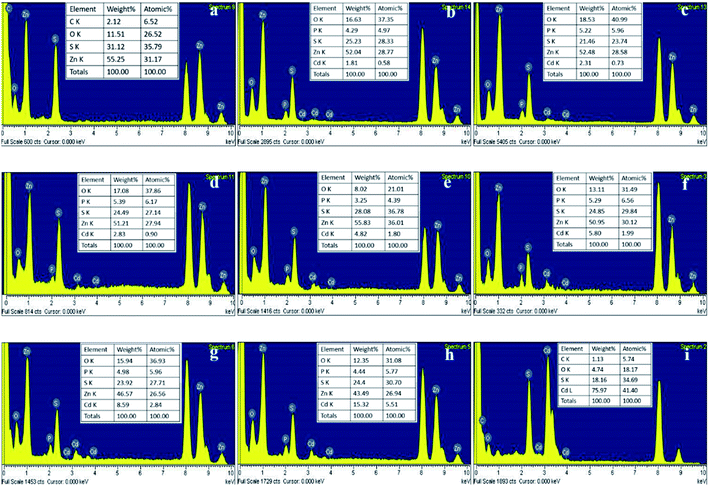 |
| Fig. 2 EDS patterns of the as-prepared ZnxCd1−xS with different molar ratios of Cd/Zn. The molar ratios of Cd/Zn = 0 : 1 (a), 1 : 50 (b), 1 : 40 (c), 1 : 30 (d), 1 : 20 (e), 1 : 15 (f), 1 : 9 (g), 1 : 5 (h), 1 : 0 (i). The appearance of copper peaks is due to the grid for TEM analysis. | |
2.1.2 Shape and size of the as-prepared ZnxCd1−xS by TEM and HRTEM. The biosynthesized ternary ZnxCd1−xS QDs were analyzed using TEM/HRTEM to accurately understand their structure, morphology and size. The TEM photographs reveal that all the as-prepared ZnxCd1−xS products with different molar ratios of Zn2+ to Cd2+ were monodisperse, well distributed, fairly uniform spheres (Fig. 3a–i). The average crystallite size (ACS) of the as-prepared ZnxCd1−xS is 3.50–4.64 nm (Fig. 3a1–3i1), suggesting that the SRB-derived supernatant rapidly fabricated ZnxCd1−xS QDs, independent of the molar ratio of Cd2+ to Zn2+. The HRTEM images depict clear lattice fringes of the nanocrystalline ZnxCd1-xS QDs' cubic structure (Fig. 3a2–3i2), exhibiting that the ZnxCd1-xS QDs have good crystallinity. The measured interplanar distance dhkl values of alloyed ZnxCd1-xS QDs (0 < x < 1) are smaller than the corresponding standard values d(111) = 3.36 Å of CdS (JCPDS: 65-2887) and larger than d(111) = 3.12 Å of ZnS (JCPDS: 65-5476) cubic structures; this was due to the substitution of Cd2+, with a greater ionic radii of 0.95 Å, by the smaller Zn2+, with an ionic radii of 0.74 Å, in the lattice sites of CdS nanocrystals.35 Based on the same reason, the lattice parameter a from HRTEM pictures also became less with the decrease of molar ratio of Cd2+ to Zn2+ in the ZnxCd1−xS QDs, which is consistent with those deduced from XRD images (Table 1).
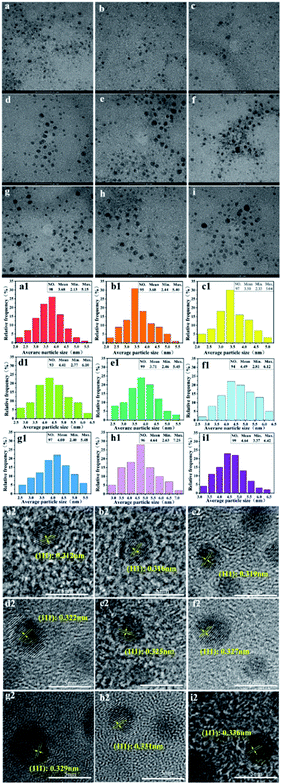 |
| Fig. 3 TEM, HRTEM and size distribution of the SRB-derived ternary ZnxCd1−xS alloyed QDs at different molar ratios of Cd/Zn. TEM of Cd/Zn = 0 : 1 (a), 1 : 50 (b), 1 : 40 (c), 1 : 30 (d), 1 : 20 (e), 1 : 15 (f), 1 : 9 (g), 1 : 5 (h), 1 : 0 (i); the particle size distribution of Cd/Zn = 0 : 1 (a1), 1 : 50 (b1), 1 : 40 (c1), 1 : 30 (d1), 1 : 20 (e1), 1 : 15 (f1), 1 : 9 (g1), 1 : 5 (h1), 1 : 0 (i1); HRTEM images of Cd/Zn = 0 : 1 (a2), 1 : 50 (b2), 1 : 40 (c2), 1 : 30 (d2), 1 : 20 (e2), 1 : 15 (f2), 1 : 9 (g2), 1 : 5 (h2), 1 : 0 (i2). | |
Table 1 Lattice parameters of cubic ZnxCd1−xS alloyed nanoparticles
Cd/Zn |
Interplanar distance d(111) (Å) |
Lattice constant (a) |
XRD |
HRTEM |
XRD |
HRTEM |
0 : 1 |
3.06 |
3.12 |
5.30 |
5.40 |
1 : 50 |
3.12 |
3.16 |
5.40 |
5.47 |
1 : 40 |
3.13 |
3.19 |
5.42 |
5.53 |
1 : 30 |
3.15 |
3.22 |
5.46 |
5.58 |
1 : 20 |
3.17 |
3.25 |
5.49 |
5.63 |
1 : 15 |
3.21 |
3.27 |
5.56 |
5.66 |
1 : 9 |
3.25 |
3.29 |
5.63 |
5.70 |
1 : 5 |
3.33 |
3.31 |
5.77 |
5.73 |
1 : 0 |
3.35 |
3.36 |
5.80 |
5.82 |
The crystalline interplanar spacing in the HRTEM images also proved that the synthesized products were pure ternary ZnxCd1−xS QDs rather than mixtures of ZnS and CdS. If a mixture of ZnS and CdS exists, the crystalline interplanar spacing would be either ZnS or CdS. However, the crystalline interplanar spacing of the prepared product, as pure ternary ZnxCd1−xS QDs, lies between ZnS and CdS. In this study, the measured interplanar distance values (dhkl) of alloyed ZnxCd1−xS QDs (0 < x < 1) are smaller than the corresponding standard values d(111) = 3.36 Å of CdS (JCPDS: 65-2887) and larger than d(111) = 3.12 Å of ZnS (JCPDS: 65-5476) cubic structures. Based on the above analysis, the product by prepared biological means is purely alloyed ZnxCd1−xS QDs rather than a mixture of ZnS and CdS.
2.1.3 PL of the biosynthesized ternary ZnxCd1−xS alloyed QDs. A strong PL is necessary for the applications of QDs in biolabeling and biosensing in life science and medicine. Especially, multicolor QDs are highly desired because they can be applied for the simultaneous biolabeling and biosensing of multiple target cells and biomolecules.2 Therefore, the PL of the SRB-derived ZnxCd1−xS QDs and the variation of PL curves under various molar ratios of Cd2+ to Zn2+ were characterized (Fig. 4). It was observed that the SRB-derived ZnxCd1−xS QDs displayed rather strong PL activity and that PL emission shifted from 450 nm to 590 nm, which almost covers the entire visible spectral region, when the molar ratio of Cd2+ to Zn2+ increased from 1
:
50 to 1
:
5, indicating that the absorption and emission peaks of the SRB-synthesized ZnxCd1−xS QDs can be easily tuned by simply changing the ratio of precursor heavy metals in the solution. As an excellent blue emitter, the PL peak of ZnxCd1−xS QDs was redshifted with difficulty to 460–596 nm when the ratio of Cd/Zn in synthesized QDs was increased to 0.25–1.0, and even as high as 4–9, using wet chemical methods.24,33–35 In contrast, the current study demonstrates that the SRB biosynthesis not only carried out quick and controlled production of ZnxCd1−xS QDs with tunable PL but also easily allowed redshift to 450–590 nm at a very low Cd/Zn ratio of 0.02–0.2.
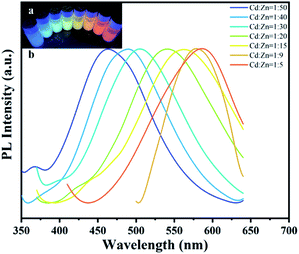 |
| Fig. 4 PL spectra of the SRB-derived ternary ZnxCd1−xS alloyed QDs at different molar ratios of Cd/Zn. The molar ratios of Cd2+ and Zn2+ ranged from 1 : 50 to 1 : 5. Inset: image of the QDs under 350 nm UV radiation. | |
2.2 EP's role in mediating ZnxCd1−xS QD synthesis and enhancing PL redshift
The functional groups on the surface of ZnxCd1−xS QDs were analyzed using FTIR to explore the interactions between the ZnxCd1−xS QDs and SRB-derived biomolecules during biosynthesis (Fig. 5a). It was confirmed that the SRB-derived ZnxCd1−xS QDs contained abundant functional groups, pointing to the amide (3293 cm−1, 1651 cm−1, 1541 cm−1, 1240 cm−1 and 1057 cm−1), C–H (2927 cm−1) and COOH (1405 cm−1) as distinct signals of protein functional groups,15,38 suggesting that the quick in vitro synthesis of ZnxCd1−xS QDs is mediated by EPs. A further analysis was carried out to explore the species and amino acid contents of the QD-adhered EPs at different molar ratios of Cd2+/Zn2+ (Fig. 5b). It was found that the biosynthesized ZnxCd1−xS QDs from different molar ratios of Cd2+/Zn2+ have almost identical amino acid compositions and percentage contents (Fig. 5c), demonstrating that the quick in vitro biosynthesis of ZnxCd1−xS QDs under different molar ratios of Cd2+/Zn2+ is mediated by the same EPs (Fig. 5d–j). The mediated EPs include high total concentrations of acidic amino acids (22.8–25.0 mol%) and extremely high total concentrations of nonpolar amino acids (51.7–55.0%), with Glu and Leu, respectively, ranking first (15.5–17.2%) and second (10.1–12.3%) as acidic and nonpolar amino acids. The high content of negatively charged acidic amino acids offers a very large number of sites for absorption of Zn2+ and Cd2+ by the EPs to induce massive nucleation, as the first step for the quick biosynthesis of ZnxCd1−xS QDs. On the other hand, the extremely high nonpolar amino acid content causes a strong hydrophobic bridging and interlinkage of the EPs to form smaller cavities that control the growth of ZnxCd1−xS QDs.15
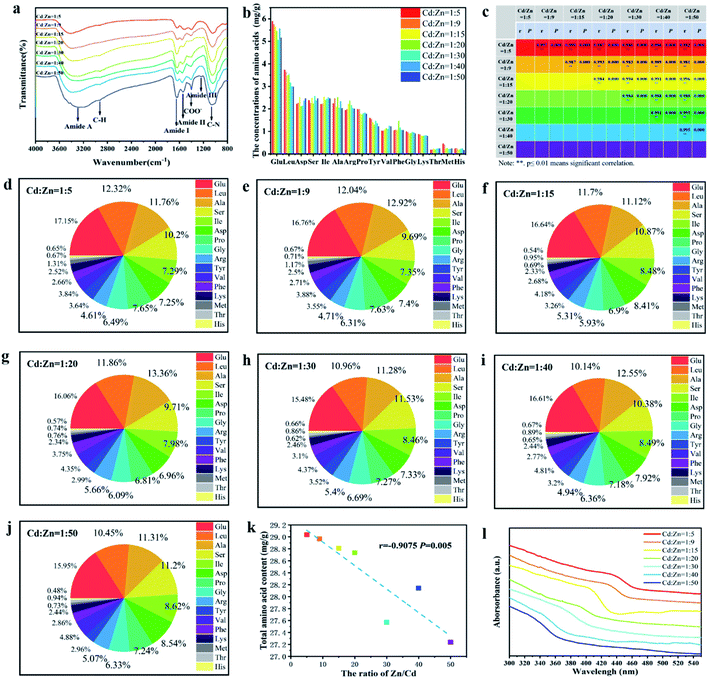 |
| Fig. 5 FTIR and UV-vis images of the SRB-derived ZnxCd1−xS QDs as well as the contents of adhered EPs and their amino acid compositions under different molar ratios of Cd/Zn. FTIR spectrum of the ZnxCd1−xS QDs (a), the amino acid species of the adhered EPs and their contents (mg g−1) in the as-prepared ZnxCd1−xS QDs (b), similarity analysis of amino acid composition and percentage contents (c), percentage of each amino acid under different molar ratios of Cd/Zn ranging from 1/5 to 1/50 (d–j), Pearson correlation analysis between the ratio of Zn/Cd and total amino acid content (k), UV-vis absorption of the SRB-derived ZnxCd1−xS alloyed QDs at different molar ratios of Cd2+ and Zn2+ from 1 : 5 to 1 : 50 (l). | |
More interestingly, it was a surprise to discover that the amount of EPs adhered to ZnxCd1−xS QDs was significantly elevated with the increase of Cd2+ concentration (a highly toxic metal) in the ternary alloyed QDs (Fig. 5k). This phenomenon might be due to a unique detoxification mechanism by the peculiar SRB through coating by the EPs free from cysteine to decrease the toxicity of toxic heavy metals,15 different from the most common detoxification mechanisms by phytochelatin (PC) and metallothionein (MT), which are rich in cysteine, to chelate heavy metal ions via the thiol groups.39,40 Because the SRB can produce a large quantity of H2S (S2−) at a high speed due to its dissimilatory nature, it secretes the unique cysteine-free EPs containing high acidic amino acid content to strongly absorb the toxic metals by electrostatic attraction as an efficient detoxification method. In contrast, the detoxification mechanisms guided by PC and MT to chelate heavy metal ions using cysteine is inefficient when facing a high content of toxic heavy metals. The efficient detoxification mechanism by the unique EPs may be linked to a smart strategy of SRB to deal with high concentrations of toxic metals.
The adhered EPs contain various amino acids, including acidic and nonpolar amino acids. The short chains (C–H, N–H, O–H) of the amino acid or the swing of the unbonded dangling bonds (C–C, C–N) result in energy loss of the nanocrystal charge radiation transition in the form of phonons (heat energy), causing redshift in the luminescence peaks.35 Because more EPs were adhered to the ZnxCd1−xS QDs in the case of high Cd2+ concentration, a greater electron transition energy loss occurred due to the increased amino acid content, leading to a larger redshift in the UV-vis absorption from 340 to 440 nm when the molar ratio of Cd2+/Zn2+ gradually rose from 1/50 to 1/5 (Fig. 5l). Accordingly, an increasing redshift of PL spectra occurred from 450 to 590 nm at a very low Cd/Zn ratio of 0.2–0.02 because of the enhancing effect of the increasing EP coating on the ZnxCd1−xS QDs (Fig. 4). Both the low Cd2+ concentration and high EP content bring about low biotoxicity of the ternary QDs with tunable multicolour, covering almost the whole visible region, which is of importance for their wide application in the fields of life science and medicine.32
2.3 Gastric acid and thermal stability of biosynthesized ZnxCd1−xS QDs
The stability of structure and fluorescence is necessary for the practical application of the ternary ZnxCd1−xS QDs in biolabeling and biosensing for imaging, tracking and sensing particles or cells.2–4 Hence, the changes in XRD and PL of the biosynthesized ZnxCd1−xS QDs with the molar ratio of Cd2+/Zn2+ at 1
:
30 in simulated gastric acid or at higher temperature were monitored (Fig. 6). The results showed that the XRD image of ZnxCd1−xS QDs almost remained unchanged in the simulated gastric acid for 3 days or after heat treatment at as high as 150 °C for 2 h (Fig. 6a and b), indicating that the biosynthesized ZnxCd1−xS QDs have a highly stable chemical structure and are resistant to both gastric acid and high temperature. However, a slight decrease occurred in the PL intensity in both cases, with 87% and 80% of PL intensity retention at the maximum emission peak in the simulated gastric acid for 3 days or after thermal treatment at 150 °C for 2 h, respectively (Fig. 6c and d), showing that the biosynthesized ZnxCd1−xS QDs are also rather stable in the performance of PL. The high stability in both structure and PL activity suggests a great application potential of the ZnxCd1−xS QDs in the fields of life science and medicine.
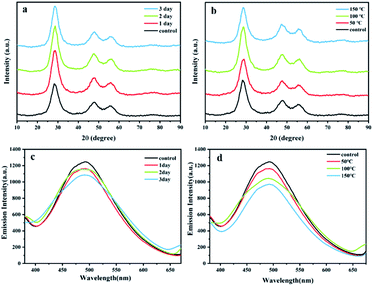 |
| Fig. 6 Gastric acidic or thermal stability of biosynthesized ZnxCd1−xS QDs. The changes in XRD pattern and PL spectra of biosynthesized ZnxCd1−xS QDs with molar ratio of Cd2+/Zn2+ at 1/30 under simulated gastric acid conditions (pH 1.5 HCl + 0.1 g mL−1 pepsin) and different contact times, from 1 day to 3 days ((a) for XRD, (c) for PL), or under heat treatment for 2 h at different temperatures, from 50 °C to 150 °C ((b) for XRD, (d) for PL). | |
To improve the thermal and acidic stability of metal sulfide QDs in both structure and PL activity, an expensive amphiphilic oligomer, PMAO, has been utilized to modify the ZnSe/CdS/ZnS core/shell QD fabricated by wet chemical process. The PL intensity of the QDs-PMAO retained over 85% after treatment in acid solution for 30 min, and the PL intensity dropped to 76% at 90 °C.31 In contrast, the biosynthesized ZnxCd1−xS QDs exhibit much higher stability in structure and PL, with 87% PL retention after 3 days of contact in simulated gastric acid and 80% retention at 150 °C for 2 h (Fig. 6). The high stability of the biogenic ZnxCd1−xS QDs is possibly attributed to the strong electrostatic attraction between Zn2+/Cd2+ and the high content of negatively charged acidic amino acids in the EPs, leading to very firm adhesion of EPs to ZnxCd1−xS QDs to form solid aggregations, which not only enhance the resistance of EPs to pepsin but also boosts the resistance of ZnxCd1−xS to strong acid and high temperature.
2.4 Biotoxicity test of the biosynthesized ZnxCd1−xS QDs
High safety and low cytotoxicity are of critical importance for the applications of metal-based QDs in the life science and medical fields, such as biolabeling, biotracing and bioimaging. Morphological and biochemical alteration, loss of cell functionality, chromosome damage and even cell death frequently take place during the uptake of QDs by cells.2 The cytotoxicity of the SRB-derived ZnxCd1−xS QDs with different molar ratios of Cd2+ to Zn2+ under altered concentrations was assessed by using the MC3T3-E1 cell viability test. It was found that at each QD dosage, the viability of cells kept declining with the increase of the molar ratio of Cd2+ to Zn2+ from 1
:
50 to 1
:
5 due to the highly toxic nature of Cd2+; an evident decrease of the cell viability also occurred when the concentration of the biosynthesized ZnxCd1−xS QDs increased from 10 to 2000 mg L−1 at each molar ratio of Cd2+ to Zn2+ (Fig. 7a). However, the biogenic ZnxCd1−xS QDs with the highest Cd2+/Zn2+ ratio at 1/5 witnessed a rather high cell viability of 81.6% at 100 mg L−1 QD concentration; 51.5% cell viability still occurred even at the highest QD concentration of 2000 mg L−1 (Fig. 7a), suggesting that the biosynthesized ZnxCd1−xS QDs have rather low cytotoxicity. An inhibition zone test also showed that the biosynthesized ZnxCd1−xS QDs at 100 mg L−1 had no effect on the growth of E. coli DH5α, whereas the chemosynthetic ZnxCd1−xS used as a control strongly inhibited cell growth (Fig. 7b), further demonstrating that the biosynthesized ZnxCd1−xS QDs are much less toxic and highly safe due to the coated EPs on the QD surface.
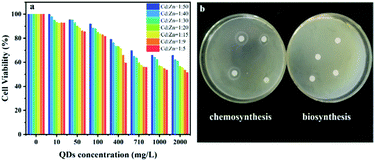 |
| Fig. 7 Biotoxicity test of the biosynthesized ZnxCd1−xS QDs. Cell viability of MC3T3-E1 after 120 h of contact with the biogenic ZnxCd1−xS QDs at varied concentrations ranging from 10 to 2000 mg L−1; the initial cell concentration was 3.52 × 105 cells per mL, and a sample with no added ZnxCd1−xS QDs acted as control (a). The inhibition zone assays for chemosynthetic and biosynthetic ZnxCd1−xS at a concentration of 100 mg L−1 using E. coli DH5α as model microorganism (b). | |
In the chemical synthesis of metal sulfide QDs, expensive biocompatible organic molecules are widely applied to produce a passivation layer on the surface of QDs in order to reduce their biotoxicity.33,34 Coating CdS QDs by 3-mercaptopropionic acid resulted in a cell viability of 92% at a low QD concentration of 0.1 mg L−1.41 CdS conjugates capped by chitosan-based ligands acquired 65% cell viability at a lower concentration of 0.02 mg L−1.42 The ZnxCd1−xS QDs with equal molar concentration of Cd and Zn, capped by sodium carboxymethyl cellulose, had a cell viability above 95% at an extremely low concentration of 2.09 × 10−3 mg L−1.33 In contrast, the SRB-derived ZnxCd1−xS QDs gained cell viability of 99.9%–92.7%, 95.4%–85.5% and 91.9%–81.6% at QDs concentrations of 10, 50 and 100 mg L−1 when the molar ratio of Cd2+ to Zn2+ increased from 1
:
50 to 1
:
5, respectively (Fig. 7a). The results demonstrated that the biogenic ZnxCd1−xS QDs have 2–4 orders of magnitude higher biocompatibility and biosafety than their counterparts produced by chemical routes due to their low Cd content and capping by unique EPs, which endows the biosynthesized ternary ZnxCd1−xS QDs with a great application potential in medical and biological fields.
2.5 Cell imaging performance of the biosynthesized ZnxCd1−xS QDs
In vitro HeLa cell imaging was used to test the cell imaging performance of the biosynthesized ZnxCd1−xS QDs (Fig. 8a–c). Fig. 8a shows that the cells fully kept their integrity in both membrane and morphology after 24 h incubation with the biogenic ZnxCd1−xS QDs at 10 mg mL−1 due to their excellent biocompatibility and high biosafety. Fig. 8b shows that the biosynthesized ZnxCd1−xS QDs, which reacted with the cells, emitted bright yellow-green PL after 24 h of contact. Fig. 8c demonstrates that the biogenic ZnxCd1−xS QDs successfully entered the cells and labelled them via PL imaging. Further, it was found that the cells kept growing, and the cell membrane integrity was maintained despite a longer contact period of 72 h between the cells and the biogenic ZnxCd1−xS QDs (Fig. 8d–g), demonstrating that the ZnxCd1−xS QDs had no effect on the growth of cells concomitant with cell imaging owing to the excellent biocompatibility. The results showed that the biosynthesized ZnxCd1−xS QDs can be used as a probe for real-time optical cellular imaging as a potential alternative to conventional organic dyes with high toxicity and poor stability.2–4
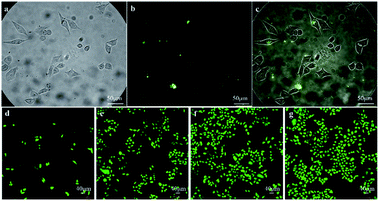 |
| Fig. 8 Cell imaging using the biosynthesized ZnxCd1−xS QDs. HeLa cells phagocytose the QDs after 24 h of contact ((a) bright field; (b) PL image; (c) overlay image). Growth of cells and membrane integrity over the course of 72 h incubation in the presence of ZnxCd1−xS QDs ((d) cell population after 24 h contact; (e) population after 48 h contact; (f) population after 72 h contact; (g) population after 72 h culture without QDs as control). The biosynthesized ZnxCd1−xS QDs have a molar ratio of Cd2+ to Zn2+ of 1 : 20; the irradiation wavelength was 340–380 nm; the emission wavelength was 550 nm; membrane integrity and cell morphology were evaluated using calcein-AM/PI; the membrane damage is shown by red cell fluorescence; the membrane integrity is shown by green cell fluorescence. In the case of control, the ZnxCd1−xS QDs were absent. | |
This is the first study to biosynthesize ternary ZnxCd1−xS QDs using the mixed SRB. The SRB-derived active supernatant, which contains huge amounts of biogenic S2− and high content of peculiar EPs, accounts for the quick extracellular biosynthesis. A very short duration of 30 minutes is required by the active supernatant to give as high as 3.5 g L−1 of ZnxCd1−xS QD productivity, which is comparable to chemical routes. The as-prepared ZnxCd1−xS QDs have good crystallinity and well-distributed and monodisperse spheres, with ACS of 3.50–4.64 nm, which is independent of the molar ratio of Cd2+ to Zn2+. When the molar ratio of Cd2+ to Zn2+ increases from 1
:
50 to 1
:
5, however, the PL emission wavelength of the biosynthesized ZnxCd1−xS QDs elevates from 450 nm to 590 nm to obtain multicolor QDs, which almost covers the entire visible region. On the other hand, the biogenic multicolor ZnxCd1−xS QDs not only have high stability in both chemical structure and PL emission to resist gastric acid and high temperature, but they also have 2–4 orders of magnitude higher biocompatibility and biosafety than the chemically synthesized counterparts. The biogenic ZnxCd1−xS QDs can enter growing HeLa cells and label them via PL imaging, and no toxic effect on cell growth and cell membranes was observed for over 72 h of contact. The high productivity, homogeneous narrow size, PL-tunable multicolor QDs, with great structure and PL stability and extremely excellent biocompatibility and biosafety, endows the SRB-derived ZnxCd1-xS alloyed QDs with incomparable advantages in biolabeling, biotracing and bioimaging.
Biosynthesis of metal-based nanoparticles or QDs has been universally accepted as environmentally friendly and energy-saving, with less pollution and producing biocompatible products, which is more desirable for application in the life science and medical fields.1–3 In the contest with chemical routes, however, biosynthesis has long been criticized as having low productivity, being time consuming, with poor control over size, shape and crystallinity, keeping this much-needed technology away from commercial applications.3,13 The current work entirely breaks through the existing bottlenecks of biosynthesis to drive it from lab to practical application with the help of the mixed SRB. The peculiar EPs secreted by the mixed SRB plays a decisive role in the high yield, tunable PL, stable structure and excellent biocompatibility of the ZnxCd1−xS QDs. The abnormally high concentration of acidic amino acids (Glu and Asp, 22.8–25.0 mol%) in the peculiar EPs is responsible for the rapid electrostatic absorption of Zn2+/Cd2+ for quick nucleation and biosynthesis, while the extremely high content of nonpolar amino acids (Leu, Ala, Ile, Pro, Gly, Val, Phe and Met, 51.7–55.0 mol%) is responsible for controlling the growth of QDs through the formation of tiny cavities via hydrophobic bridging and interlinkage.15 The far tight adhesion between the EPs and the ZnxCd1-xS QDs due to strong electrostatic reaction accounts for their high stability in the face of gastric acid conditions and high temperature. Particularly, when the molar ratio of Cd2+ to Zn2+ increased from 1
:
50 to 1
:
5, the amount of EPs adhered into the ZnxCd1−xS QDs also evidently increased, which not only enhanced the PL redshift from 450 nm to 590 nm at a very low Cd/Zn ratio of 0.02–0.2 but also markedly improved the biocompatibility and biosafety. The multiple functions of the SRB-derived EPs in mediating the biosynthesis of ZnxCd1−xS QDs are unique, totally different from the most common EPs, such as PC and MT, which are rich in cysteine as a detoxification mechanism.39,40
3 Experimental
3.1 Chemicals, microorganisms and media
All chemicals were analytical grade, obtained from Beijing Chemical Industry and used as received without further purification, including lactic acid as an electron donor, Na2SO4 (SO42−) as an electron acceptor, and ZnSO4/CdCl2 as precursor Zn2+/Cd2+ for the biosynthesis of ZnxCd1−xS QDs. Deionized water was used to prepare the solutions for both cell culture and biosynthesis of ZnxCd1−xS QDs.
The mixed SRB, which had performed the highest-ever extracellular biosynthesis of binary metal sulfide QDs,15 was applied to fabricate the ternary ZnxCd1−xS alloyed QDs in a modified, easier and faster manner in this work. The media contained 1.0 g L−1 NH4Cl, 0.5 g L−1 K2HPO4, 0.5 g L−1 MgSO4, 0.1 g L−1 CaCl2, 0.1 mol L−1 lactic acid, and 0.1 mol L−1 Na2SO4, at pH 7.2.
3.2 Quick biosynthesis of alloyed ZnxCd1−xS QDs
Fresh media was inoculated with the mixed SRB at a density of 10% (v/v), then the inoculated media was anaerobically incubated at 35 °C. When the mixed SRB reached logarithmic phase after about 4 days of culture, the turbidity of SRB (OD600) increased from 0.1 to 0.6. At that time, the cells were removed by centrifuging at 8000 rpm for 10 min to obtain the supernatant, which contained massive amounts of biogenic H2S (S2−) from sulfate bioreduction and the peculiar EPs secreted by the mixed SRB.15 Subsequently, 200 mL of the SRB-derived active supernatant was mixed with 200 mL of mixed ZnSO4 and CdCl2 solutions at different molar ratios (Zn
:
Cd = 0
:
1, 5
:
1, 9
:
1, 15
:
1, 20
:
1, 30
:
1, 40
:
1, 50
:
1, 1
:
0; total concentration of Zn2+ and Cd2+ = 0.1 mol L−1). The EPs-mediated precipitation reaction between biogenic S2− and the mixed solution of Zn2+ and Cd2+ occurred in a 500 mL beaker with a magnetic stirrer (30 rpm, 25 °C). After 30 minutes of contact, the colorless transparent solution gradually changed into a milky-white or slightly milky-yellow suspension liquid, displaying the formation of alloyed ZnxCd1−xS QDs. Then, the resulting ternary ZnxCd1−xS QDs were collected by centrifuging (8000 rpm, 10 min), washed three times, respectively, with deionized water and ethanol. Finally, the ZnxCd1−xS QDs were freeze-dried under vacuum at −80 °C, ground into a powder and sealed for use.
3.3 Characterization of the extracellular biosynthesized ZnxCd1−xS QDs
The productivity of ternary ZnxCd1−xS QDs synthesized using the SRB-derived supernatant and the utilization efficiencies of Zn2+ and Cd2+ were obtained by calculating the difference of Zn2+ and Cd2+ concentrations before and after precipitation using inductively coupled plasma optical emission spectrometry (ICP-OES, PerkinElmer Optima 8300, USA). The crystalline phase of the vacuum-dried powder ZnxCd1−xS alloyed QDs was characterized using X-ray diffraction (XRD, Hitachi Rigaku, Tokyo, Japan) with Cu Kα radiation (λ = 1.5418 Å). The morphology and size of the ZnxCd1−xS alloyed QDs were analyzed using transmission electron microscopy (TEM, Hitachi H-800, Tokyo, Japan) at an acceleration voltage of 200 kV with a CCD camera, and further examination was performed using high-resolution transmission electron microscopy (HRTEM). The size distribution and average crystallite size (ACS) of the as-prepared ZnxCd1−xS QDs were calculated based on the TEM images using the Nano Measurer 1.2 software. For this purpose, 10 pictures and 100 single nanoparticles were randomly chosen. The purity and composition of the ternary QDs were characterized using energy-dispersive X-ray spectroscopy (EDS, Oxford) with an instrument operating at 20 kV. Infrared absorption spectra of the ZnxCd1−xS QDs were analyzed by Fourier transform infrared spectroscopy (FTIR) using a Thermo Nicolet iS10 spectrophotometer. The thick films of the analytes were prepared by employing a mixture of KBr and the sample. UV-vis absorption and photoluminescence (PL) emission spectra were measured using a Hitachi F-4500 fluorescence spectrometer.
3.4 Amino acid analysis of the mediating proteins coating the ZnxCd1−xS QDs
The species and concentrations of the amino acids in the mediating proteins attaching to the ZnxCd1−xS QDs were analysed based on standard procedure (ISO 13903-2005). A certain small amount of the dried powder of ZnxCd1−xS QDs was added to a 12 mol L−1 HCl solution in a hydrolysis flask, followed by adding a few drops of phenol. The hydrolysis flask was sealed after the air was completely displaced with nitrogen. The sealed hydrolysis flask was then placed in a thermotank at 110 °C for 24 h to hydrolyze the proteins. The hydrolysate was then cooled and filtered, and HCL was removed under reduced pressure at 60 °C. Finally, the residual was dissolved in a sodium citrate buffer (pH 2.2) to determine the species and concentrations of amino acids using an amino acid analyser (L-8900, Hitachi high-Technologies Corp., Japan).
3.5 Stability assessment of ZnxCd1−xS QDs under sterilization and gastric juice
In order to assess the thermal stability of the as-prepared ZnxCd1−xS QDs after sterilization for applications in medicine and life sciences, variation in PL and XRD images of the resulting product synthesized at Zn/Cd = 30
:
1 was measured; QDs were respectively pre-treated at 50 °C, 100 °C and 150 °C for 2 h in a aqueous solution containing 2.0 g L−1 of ZnxCd1−xS QDs. To assess their stability in a gastric acidic environment, the dried powder of ZnxCd1−xS QDs at 2.0 g L−1 was placed into a simulated stomach juice (a mixed solution of HCl at pH 1.5 and pepsin at 0.1 g mL−1) to contact at 37 °C for 1, 2, and 3 days. The ZnxCd1−xS QDs were harvested at different contact times to monitor the changes of PL and XRD images. The raw samples without thermal treatment and acidic contact were used as controls.
3.6 Biotoxicity test of the ZnxCd1−xS QDs produced by the SRB-derived supernatant
3.6.1 MTT assay. All biological tests were carried out according to ISO 10993-5: 2009 (Biological evaluation of medical devices: Tests for in vitro cytotoxicity). MTT assay was done using 3-(4,5-dimethylthiazol-2yl)2,5-diphenyltetrazolium bromide; MC3T3-E1 cells were plated (3 × 104 cells per well) in 96-well plates. Cell populations were synchronized in serum-free media for 24 h. Subsequently, the media was suctioned out and replaced with an equal volume of Dulbecco's modified Eagle's medium (DMEM) containing 10% fetal bovine serum (FBS) and incubated for 24 h. The as-prepared ZnxCd1−xS QD colloidal solutions were added into individual wells at final concentrations of 0.01, 0.05, 0.1, 0.4, 0.71, 1.0 and 2.0 g L−1, respectively. Controls were run with cells and DMEM. After 120 h, all media were aspirated and replaced with 60 L of culture media containing serum in each well. MTT (5 mg mL−1, Sigma-Aldrich, USA) was added into each well and incubated for 4 h in an oven (37 °C, 5% CO2). Next, 40 μL SDS (Sigma-Aldrich, USA) solution/4% HCl was placed in each well, followed by incubation for 16 h in an oven. Next, 100 μL sample was removed from each well and transferred to a 96-well plate. The absorbance values (Abs) were measured at 595 nm on an iMark™ Microplate Absorbance Reader (Bio-Rad). Percentage cell viability was calculated according to eqn (1). The values of the controls (wells with cells, and no samples) were set to 100% cell viability. |
 | (1) |
3.6.2 Inhibition zone test. The model organism Escherichia coli (E. coli) DH5α was used for the inhibition zone test. The inhibition zone of ZnxCd1−xS QDs was determined by disk diffusion method in the agar plates. Filter papers impregnated with ZnxCd1−xS QDs were placed on an E. coli DH5α culture dish to monitor the growth inhibition of surrounding cells. The powder ZnxCd1-xS QDs from Zn2+/Cd2+ = 5
:
1, the highest ratio of Cd/Zn in this study, was used to test the biological toxicity. Chemically synthesized ZnxCd1-xS was obtained by precipitating the same mixed solution of Zn2+ and Cd2+ with 0.1 mol L−1 Na2S instead of the SRB-derived supernatant. Both biogenic and chemically synthesized ZnxCd1-xS at 0.1 g L−1 were compared for their biological toxicity based on the inhibition zone test.
3.7 Application assessment of biosynthesized ZnxCd1−xS QDs in life science & medicine
3.7.1 Membrane integrity (calcein AM/PI staining). Cell apoptosis was evaluated by calcein AM/PI staining, with AM as the live cell stain (green fluorescence) and PI as dead cell stain (red fluorescence). HeLa cells were plated (1.5 × 105 cells per well) in 6-well plates. The cell populations were synchronized in serum-free media for 24 h. After that, the media was suctioned and replaced with Dulbecco's modified Eagle's medium (DMEM) containing 10% fetal bovine serum (FBS), then incubated for 24 h. The as-prepared ZnxCd1−xS QD sample solutions were added to individual wells at final concentrations of 10 mg mL−1 for 24 h, 48 h and 72 h, respectively. Controls had cells and DMEM. After co-incubation, the cells in the six-well plates were rinsed with phosphate buffered saline (PBS) to remove excess serum. Then, 2 mM calcein-AM and 2.5 mg mL−1 PI were thoroughly mixed with the cells and co-incubated in the dark for 15 min at 37 °C. The cells were then washed and soaked in PBS for 15 min to eliminate nonspecific staining. The cells were observed under a confocal microscope (OLYMPUS, FV10-MPSU).
3.7.2 Cell imaging of the biosynthesized ZnxCd1−xS QDs. HeLa cells were cultured in high-glucose DMEM media supplemented with 10% FBS and 1% penicillin/streptomycin for 24 h at 37 °C in a humidified 5% CO2 incubator. Suspensions of the biosynthesized ZnxCd1−xS alloyed QDs at 10 mg mL−1 were prepared from the stock solution with serum-containing DMEM. HeLa cells were seeded in six-well plates with a density of 1.5 × 105 cells per well for the uiptake experiments. The growth medium was replaced with the ZnxCd1−xS QD suspensions for another 24 h of incubation at 37 °C. The cells were then fixed and recorded with a fluorescence microscope with an emission wavelength at 340–380 nm after the excess ZnxCd1−xS QDs were removed by washing 3 times with warm PBS.
4 Conclusions
The SRB-derived supernatant is capable of quick and extracellular biosynthesis of the ternary ZnxCd1−xS QDs. Under mild conditions, as high as 3.5 g L−1 of ZnxCd1−xS QDs with ACS of 3.50–4.64 nm was produced by the active supernatant within 30 minutes, which is comparable to the chemical route. By changing the molar ratio of Cd2+ to Zn2+, the PL emission of the ZnxCd1−xS QDs increases from 450 to 590 nm to yield multicolor QDs. Compared with the chemically synthesized counterparts, the biogenic ZnxCd1−xS QDs have greater resistance against gastric acid and high temperature as well as 2–4 orders of magnitude higher biocompatibility and biosafety, successfully entering the growing HeLa cells and labelling them via PL imaging without detectable harm to cell growth and cell membranes for 72 h. The SRB-secreted peculiar EPs containing abnormally high content of acidic amino acids and extremely high concentration of nonpolar amino acids play a dominant role in the quick and extracellular biosynthesis of ZnxCd1−xS QDs. The carboxyls in the acidic amino acids offer a large number of negatively charged sites for the absorption of Zn2+/Cd2+ for rapid nucleation, while the nonpolar amino acids form small cavities to control the growth of QDs. On the other hand, the electrostatic force-driven firm links between the ZnxCd1−xS QDs and the peculiar EPs, as well as the increasing amount of EPs adhered to the QDs in smart response to the increasing Cd2+ content, account for the high stability in structure and PL emission as well as excellent biocompatibility and biosafety. The multiple functions of the EPs are unique in this biosynthesis.
Conflicts of interest
There are no conflicts to declare. The manuscript was written through contributions of all authors. All authors have given approval to the final version of the manuscript.
Acknowledgements
We highly appreciate and thank financial support from the National Key Research and Development Program (2018YFC1900304, 2018YFC1900301), National Natural Science Foundation of China (21777007), Beijing Natural Science Foundation (8172042), and China Postdoctoral Science Foundation (2020M680364).
Notes and references
- C. T. Matea, T. Mocan, F. Tabaran, T. Pop, O. Mosteanu, C. Puia, C. Iancu and L. Mocan, Int. J. Nanomed., 2017, 12, 5421–5431 CrossRef CAS PubMed.
- I. V. Martynenko, A. P. Litvin, F. Purcell-Milton, A. V. Baranov, A. V. Fedorov and Y. K. Gun'ko, J. Mater. Chem. B, 2017, 5, 6701–6727 RSC.
- J. Mal, Y. V. Nancharaiah, E. D. van Hullebusch and P. N. L. Lens, RSC Adv., 2016, 6, 41477–41495 RSC.
- M. Geszke-Moritz and M. Moritz, Mater. Sci. Eng., C, 2013, 33, 1008–1021 CrossRef CAS.
- O. V. Singh, Bio-Nanoparticles–Biosynthesis and Sustainable Biotechnological Implications, Wiley-Blackwell, John Wiley & Sons, Inc., Hoboken, New Jersey, 2015, p. 33 Search PubMed.
- C. Burda, X. Chen, R. Narayanan and M. A. El-Sayed, Chem. Rev., 2005, 105, 1025–1102 CrossRef CAS.
- K. N. Thakkar, S. S. Mhatre and R. Y. Parikh, Nanomedicine, 2010, 6, 257–262 CrossRef CAS.
- V. Bansal, A. Bharde, R. Ramanathan and S. K. Bhargava, Adv. Colloid Interface Sci., 2012, 179, 150–168 CrossRef.
- J. Aguiera-Sigalat, S. Rocton, J. F. Sánchez-Royo, R. E. Galian and J. Pérez-Prieto, RSC Adv., 2012, 2, 1632–1638 RSC.
- M. R. Hosseini and M. N. Sarvi, Mater. Sci. Semicond. Process., 2015, 40, 293–301 CrossRef.
- J. G. Sandana Mala and C. Rose, J. Biotechnol., 2014, 170, 73–78 CrossRef CAS PubMed.
- T. J. Park, K. G. Lee and S. Y. Lee, Appl. Microbiol. Biotechnol., 2016, 100, 521–534 CrossRef CAS PubMed.
- M. R. Hosseini, M. Schaffie, M. Pazouki, A. Schippers and M. Ranjbar, Mater. Sci. Semicond. Process., 2013, 16, 250–255 CrossRef CAS.
- K. Prasad and A. K. Jha, J. Colloid Interface Sci., 2010, 342, 68–72 CrossRef CAS PubMed.
- S. Y. Qi, S. H. Yang, J. Chen, T. Q. Niu, Y. F. Yang and B. P. Xin, ACS Appl. Mater. Interfaces, 2019, 11, 10442–10451 CrossRef CAS.
- C. Gallardo, J. P. Monrás, D. O. Plaza, B. Collao, L. A. Saona, V. Durán-Toro, F. A. Venegas, C. Soto, G. Ulloa, C. C. Vásquez, D. Bravo and J. M. Pérez-Donoso, J. Biotechnol., 2014, 187, 108–115 CrossRef CAS PubMed.
- T. J. Park, S. Y. Lee, N. S. Heo and T. S. Seo, Angew. Chem., Int. Ed., 2010, 49, 7019–7024 CrossRef CAS PubMed.
- Y. Zhang, H. Yang, X. An, Z. Wang, X. Yang, M. Yu, R. Zhang, Z. Sun and Q. Wang, Small, 2020, 16, 2001003 CrossRef CAS PubMed.
- M. Bouroushian, Electrochemistry of metal chalcogenides, Springer Science & Business Media, New York, 2010, p. 26 Search PubMed.
- N. G. Imam and M. B. Mohamed, Superlattices Microstruct., 2014, 73, 203–213 CrossRef CAS.
- I. Devadoss and S. Muthukumaran, Phys. E, 2015, 72, 111–119 CrossRef CAS.
- V. P. Kumar, A. Y. Sharma, D. K. Sharma and D. K. Dwivedi, Optik, 2014, 125, 1209–1211 CrossRef CAS.
- J. Datta, M. Das, A. Dey, S. Halder, S. Sil and P. P. Ray, Appl. Surf. Sci., 2017, 420, 566–578 CrossRef CAS.
- M. Yang, Y. Wang, Y. Ren, E. Liu, J. Fan and X. Hu, J. Alloys Compd., 2018, 752, 260–266 CrossRef CAS.
- O. Y. Wang, L. Wang, Z. H. Li, Q. L. Xu, Q. L. Lin, H. Z. Wang, Z. L. Du, H. B. Shen and L. S. Li, Nanoscale, 2018, 10, 5650–5657 RSC.
- X. Q. Li, D.-E. Yin, S.-Z. Kang, J. Mu, J. Wang and G. D. Li, Colloids Surf., A, 2011, 384, 749–751 CrossRef CAS.
- S. Bhandari, R. Begum and A. Chattopadhyay, RSC Adv., 2013, 3, 2885–2888 RSC.
- H. He, C. Li, Y. Tian, P. Wu and X. Hou, Anal. Chem., 2016, 88, 5892–5897 CrossRef CAS.
- N. Yin, L. Liu, P. Li and S. Zhao, Luminescence, 2018, 33, 630–635 CrossRef CAS PubMed.
- V. I. Kochubey, E. K. Volkova and J. G. Konyukhova, J. Biomed. Opt., 2013, 19, 011020 CrossRef PubMed.
- S. Wang, J. J. Li, Y. Lv, R. Wu, M. Xing, H. Shen, H. Wang, L. S. Li and X. Chen, Nanoscale Res. Lett., 2017, 12, 380 CrossRef PubMed.
- S. Qu, F. Sun, Z. Qiao, J. Li and L. Shang, Small, 2020, 16, 1907633 CrossRef CAS PubMed.
- A. A. P. Mansur, F. G. De Carvalho, R. L. Mansur, S. M. Carvalho, L. C. De Oliveira and H. S. Mansur, Int. J. Biol. Macromol., 2017, 96, 675–686 CrossRef CAS PubMed.
- Z. Bujňáková, M. Baláž, E. Dutková, P. Baláž, M. Kello, G. Mojžišová, J. Mojžiš, M. Vilková, J. Imrich and M. Psotka, J. Colloid Interface Sci., 2017, 486, 97–111 CrossRef PubMed.
- M. A. Osman, A. G. Abd-Elrahim and A. A. Othman, J. Alloys Compd., 2017, 722, 344–357 CrossRef CAS.
- L. Jing, S. V. Kershaw, Y. Li, X. Huang, Y. Li, A. L. Rogach and M. Gao, Chem. Rev., 2016, 116, 10623–10730 CrossRef CAS PubMed.
- M. Liu, Y. He, H. Chen, H. Zhao, W. You, J. Shi, L. Zhang and J. Li, Int. J. Hydrogen Energy, 2017, 42, 20970–20978 CrossRef CAS.
- L.-J. Tian, W.-W. Li, T.-T. Zhu, G.-H. Zhao, X.-W. Liu, J.-C. Dong, P.-F. An, J.-Y. Ma, F. Shen, C. Qian, B. Hue and H.-Q. Yu, J. Mater. Chem. A, 2019, 7, 18480–18487 RSC.
- E. Grill, E.-L. Winnacker and M. H. Zenk, FEBS Lett., 1986, 197, 115–120 CrossRef CAS.
- H. V. Perales-Vela, J. M. Peña-Castro and R. O. Cañizares-Villanueva, Chemosphere, 2006, 64, 1–10 CrossRef CAS PubMed.
- H. Li, M. Li, W. Y. Shih, P. I. Lelkes and W.-H. Shih, J. Nanosci. Nanotechnol., 2011, 11, 3543–3551 CrossRef CAS PubMed.
- H. S. Mansur, A. A. P. Mansur, A. Soriano-Araujo and Z. I. P. Lobato, Green Chem., 2015, 17, 1820–1830 RSC.
|
This journal is © The Royal Society of Chemistry 2021 |
Click here to see how this site uses Cookies. View our privacy policy here.