DOI:
10.1039/D1RA03783H
(Paper)
RSC Adv., 2021,
11, 21170-21178
Selective removal of toxic organic dyes using Trӧger base-containing sulfone copolymers made from a metal-free thiol-yne click reaction followed by oxidation†
Received
14th May 2021
, Accepted 9th June 2021
First published on 15th June 2021
Abstract
Three copolymers TCP1–3 bearing Trӧger's base (TB) units intercalated with various thioether groups were synthesized using a catalyst-free thiol-yne click reaction. TCP1–3 display excellent solubility in common organic solvents allowing for their structural, and photophysical characterization. The thioether groups in TCP1–3 were selectively oxidized into their respective sulfone derivatives under mild oxidation reaction conditions affording the postmodified copolymers TCP4–6. Investigation of organic dye uptake from water by TCP1–6 proved their efficiency as selective adsorbents removing up to 100% of the cationic dye methylene blue (MEB) when compared to anionic dyes, such as Congo red (CR), methyl orange (MO) and methyl blue (MB). The sulfone-containing copolymers TCP4–6 display superior and faster MEB removal efficiencies with respect to their corresponding synthons TCP1–3.
1. Introduction
Organic dyes, which could be either anionic such as methyl orange (MO), methyl blue (MB) and Congo red (CR) or cationic like methylene blue (MB) are widely used in pharmaceutical, food, textile, and paper industries.1 Most ionic organic dyes are water-soluble, non-biodegradable, and biologically active revealing mutagenic and carcinogenic properties.2,3 Consequently, the aforementioned dyes are classified as pollutants causing adverse environmental impacts and are also considered highly toxic due to their bioaccumulation, and skin and eye irritation, besides being potential allergens causing various respiratory complications.4,5 Consequently, removal of toxic organic dyes from freshwater resources using viable and efficient techniques is deemed crucial. Among the various techniques that have been developed to remove organic dyes from water,6–8 adsorption proved to be promising because it is usually employed under ambient conditions and is highly efficient, cost-effective, and simple.9,10
Trӧger's base (TB)11 is a bowl-shaped heterocyclic compound which has been thoroughly investigated as a chiral building block, particularly in supramolecular systems.12–16 Since the last decade, there has been a growing interest in the synthesis of copolymers with TB units for applications in gas storage and separation materials.12,17–19 The introduction of TB in the copolymer's structure bestows the latter with better gas selectivity and enhanced microporosity properties. The intricate structure of TB allows for trapping acidic gases more efficiently, whereas its rigid bicyclic bridge with a twist angle of 117° leads to zigzag copolymer chains with a high fractional free volume (FFV).20,21 These interesting features have prompted researchers to employ TB in the synthesis of solution-processable polymers of intrinsic microporosity (PIMs) for gas separation membranes.22–29 PIMs bearing Trӧger's base (PIM-TBs) units reveal enhanced separation properties for several gas pairs, such as, O2/N2, H2/N2, H2/CH4, CO2/N2, and CO2/CH4, where Robeson's upper bounds for permeability and selectivity had to be redefined.30–32 Although there are numerous reports on TB-containing materials for gas separation applications, other properties have been scarcely investigated. For instance, the optical and electrochemical properties have been reported for some TB-containing polymers,33 whereas the adsorption of picric acid from water was evaluated for other derivatives.34 Recently, covalent organic polymers containing TBs were employed for the removal of acid dyes from aqueous media.35
Click chemistry dates back to the early work of Sharpless et al.36 and was since then considered to be a conspicuous synthetic tool37 because of several advantages, among others, mild reaction conditions, fast reaction rates, versatility, stereospecificity, and high yields.38–40 Click reactions have been employed in various fields, namely, pharmaceuticals, bioconjugated products, materials, and polymers.41 Amongst the wide range of click reactions reported in the literature, nucleophilic ring opening, Michael addition, Diels–Alder, and azide-yne are the most prominent.42–46 Recently, thiols click reactions have seen a noticeable surge and were employed under several reaction conditions e.g. metal catalysts, radical initiators, and UV-irradiation.47–49 Thiol-yne click reactions have been reported in the synthesis of functional materials for various applications,13,41,50,51 notably, to make conjugated polymers52 where sulfur atoms act as bridging groups between the aromatic building blocks which, consequently, leads to a superior conductivity.39,53
The oxidation of thioether copolymers into their corresponding sulfone derivatives has proven to be an effective technique to increase the polarity of the resulting copolymers53 and bestow them with improved thermal, mechanical, and electrical properties.54–56 Copolymers bearing sulfone groups are widely utilized in fuel cells,57 organic light emitting diodes (OLEDs),54,58 chemosensors,59 proton exchange,60 organic solvent nanofilters,61 and gas separation membranes.62 Typically, thioether groups can be oxidized into sulfones either by employing oxone63 or hydrogen peroxide.64
Trӧger's base copolymers connected by thioether units TCP1–3 were synthesized via a metal free thiol-yne click polymerization reaction under mild reaction conditions. TCP1–3 were subsequently oxidized into their corresponding sulfone target copolymers TCP4–6 using the environmentally friendly hydrogen peroxide. Dye adsorption properties of TCP1–6 were tested using various organic dyes, revealing a higher affinity for the Trӧger's base containing sulfone copolymers to adsorb cationic dyes when compared to their thioether synthons.
2. Experimental
2.1 General
All the reactions were carried out under inert atmosphere using dry Argon. All chemical reagents were used without further purification as purchased from Aldrich, Merck, Alfa Aesar, CDH, and HiMedia unless otherwise specified. Anhydrous solvents, namely, hexane, DCM, THF, methanol, diethyl ether, and acetone were further dried over molecular sieves and deoxygenated by bubbling with argon gas for 30 minutes. The Trӧger's Base derivative TB was synthesized using a reported procedure in the literature65 which was slightly changed (cf. the ESI file†). Thin layer chromatography was performed on aluminum sheets coated with silica gel 60 F254 and revealed using a UV lamp. NMR (1H: 600 MHz, 13C: 150 MHz) spectra were recorded on Bruker BioSpin GmbH 600 MHz spectrometer using CD2Cl2 or DMSO-d6 as a solvent with the chemical shifts (δ) given in ppm and referred to tetramethylsilane (TMS). Electron impact high-resolution mass spectra (EI-HRMS) were recorded on a Thermo (DFS) with a standard PFK (perfluorokerosene) as lock mass. The analyzed data is converted to accurate mass employing X-Calibur accurate mass calculation software. UV-vis spectra were recorded on Shimadzu UV1800 spectrophotometer. Photoluminescence spectra were recorded on an Agilent G9800 Cary Eclipse Fluorescence spectrophotometer. Agilent Gel Permeation Chromatography (GPC/SEC) equipped with two columns (PL mixed-C) and calibrated against twelve monodisperse polystyrene (PS) standards, using THF as eluent at a flow rate of 1.0 mL min−1, was employed to determine the relative weight-average (Mw), number-average (Mn) molecular weights, and polydispersity index (Đ = Mw/Mn) of all the reported copolymers. FTIR spectra were recorded on FT/IR-6300 type A instrument using a KBr matrix.
2.2 Synthesis
2.2.1 Synthesis of monomer TB-S. Diethynyl Trӧger's base TB (149 mg, 0.5 mmol, 1 eq.) and 3,4-difluorobenzenethiol 1 (0.110 mL, 1.0 mmol, 2 eq.) in THF (5.0 mL) were charged in a Schlenk tube under argon. The reaction mixture was heated at 50 °C overnight and the resulting solution was evaporated under reduced pressure. The desired product was isolated using silica gel column chromatography with ethyl acetate/hexane (10
:
90 v/v) as the eluent affording a pale-yellow solid (215 mg, 73%). 1H-NMR (600 MHz, CD2Cl2, ppm): δ 7.39–7.12 (m, 10H, ArH), 6.82 (m, 2H, alkene-CH), 6.69 (m, 4H, alkene-CH), 6.53 (m, 2H, alkene-CH), 4.57 (d, 2H, J = 16 Hz, methylene-CH), 4.34 (m, 2H, methylene-CH), 4.01 (d, 2H, J = 16 Hz, methylene-CH), 2.41 (s, 6H, methyl-CH); 13C-NMR (150 MHz, CD2Cl2, ppm): δ 151.17, 149.51, 146.13, 133.64, 131.51, 129.63, 128.26, 126.82, 125.51, 124.67, 122.24, 121.20, 120.06, 118.26, 118.21, 117.88, 67.66, 55.09, 17.32; EI-HRMS: m/z calculated for (M˙+) C33H26F4N2S2 590.1468 found 590.1467; FTIR (KBr, cm−1): 3023 (
C–H str), 2953 (–C–H str), 1601 (conjugated alkene C
C str), 1505 (Ar C–C str), 1266 (C–F str), 963 (trans; C
C ben), 766 (cis; C
C ben).
2.2.2 Synthesis of copolymer TCP1 (procedure A). Diethynyl TB (149 mg, 0.5 mmol, 1 eq.) and 1,4-benzenedithiol 2a (71 mg, 0.5 mmol, 1 eq.) in THF (20 mL) were reacted in a Schlenk tube under argon at 50 °C for 24 hours. The resulting solution was further diluted with THF (20 mL) and added dropwise to 250 mL of hexane while stirring. The precipitate was filtered off and washed successively with hexane (20 mL), acetone (20 mL), and methanol (20 mL). Pale yellow solid (175 mg, 85%). 1H-NMR (600 MHz, CD2Cl2, ppm): δ 7.34–7.22 (bm, 4H, ArH), 7.09 (brd, 2H, ArH), 6.79 (brd, 2H, alkene-CH), 6.50 (brd, 2H, alkene-CH), 4.56 (br, 2H, methylene-CH), 4.33 (br, 2H, methylene-CH), 4.02 (br, 2H, methylene-CH), 2.43 (s, 6H, methyl-CH); 13C-NMR (150 MHz, CD2Cl2, ppm): δ 145.39, 133.16, 132.75, 131.72, 130.18, 130.09, 129.74, 129.59, 128.28, 127.92, 126.71, 124.66, 122.13, 67.64, 55.23, 16.96; GPC (THF); Mw (g mol−1): 8675 Mn (g mol−1): 2840, Đ: 3.0; FTIR (KBr, cm−1): 3011 (
C–H str), 2939 (–C–H str), 1601 (conjugated alkene C
C str), 1474 (Ar; C–C str), 962 (trans; C
C ben), 726 (cis; C
C ben); UV-vis: (THF, 10−7 M), λmax [nm] = 325.
2.2.3 Synthesis of copolymer TCP2. TCP2 was prepared following procedure A with: TB (149 mg, 0.5 mmol, 1 eq.), biphenyl-4,4′-dithiol 2b (105 mg, 0.5 mmol, 1 eq.) in THF (20 mL). Pale yellow solid (195 mg, 80%). 1H-NMR (600 MHz, CD2Cl2, ppm): δ 7.56–7.43 (bm, 8H, ArH), 7.02 (bm, 2H, ArH), 6.81–6.71 (m, 2H, alkene-CH), 6.52 (brd, 2H, alkene-CH), 4.64 (br, 2H, methylene-CH), 4.35 (br, 2H, methylene-CH), 4.03 (br, 2H, methylene-CH), 2.47 (s, 6H, methyl-CH); 13C-NMR (150 MHz, CD2Cl2, ppm): δ 145.34, 133.20, 132.78, 132.40, 131.86, 129.93, 129.62, 129.39, 128.22, 127.96, 127.49, 127.43, 126.71, 124.67, 124.60, 123.69, 122.14, 67.67, 55.21, 16.99; GPC (THF); Mw (g mol−1): 7796 Mn (g mol−1): 2732, Đ: 2.8; FTIR (KBr, cm−1): 3021 (
C–H str), 2941 (–C–H str), 1605 (conjugated alkene C
C str), 1476 (Ar; C–C str), 969 (trans; C
C ben), 807 (C–H ben), 716 (cis; C
C ben); UV-vis: (THF, 10−7 M), λmax [nm] = 330.
2.2.4 Synthesis of copolymer TCP3. TCP3 was prepared following procedure A with: TB (149 mg, 0.5 mmol, 1 eq.), 4,4′-bis(mercaptomethyl)biphenyl 2c (123 mg, 0.5 mmol, 1 eq.) in THF (20 mL). Pale-yellow solid (225 mg, 87%). 1H-NMR (600 MHz, DMSO-d6, ppm): δ 7.90–7.33 (bm, 10H, ArH), 7.14 (brd, 1H, ArH), 7.08 (brd, 1H, ArH), 6.35 (brd, 2H, alkene-CH), 4.48 (br, 2H, methylene-CH), 4.17 (br, 2H, methylene-CH), 4.08–3.97 (br, 6H, benzyl & methylene-CH), 2.31 (s, 6H, methyl-CH); 13C-NMR (150 MHz, DMSO-d6, ppm): δ 139.82, 132.64, 130.63, 129.95, 129.26, 128.84, 127.75, 127.01, 67.17, 55.11, 40.52, 17.35; GPC (THF); Mw (g mol−1): 4305 Mn (g mol−1): 1502, Đ: 2.8; FTIR (KBr, cm−1): 3027 (
C–H str), 2947 (–C–H str), 1605 (conjugated alkene C
C str), 1477 (Ar; C–C str), 951 (trans; C
C ben), 807 (C–H ben); UV-vis: (THF, 10−7 M), λmax [nm] = 296.
2.2.5 Synthesis of the sulfone monomer TB-SO2. To a stirring suspension of TB-S (50 mg, 0.08 mmol) in acetic acid (2.5 mL), was added dropwise 1 mL of aqueous hydrogen peroxide (aq. H2O2, 30 wt%). The reaction mixture was stirred at 80 °C for 15 min. The resulting pale-yellow precipitate was filtered off and washed with deionized water (20 mL) and diethyl ether (20 mL) then dried under vacuum to yield TB-SO2 as a pale-yellow solid (54 mg, 98%). EI-HRMS: m/z calculated for (M˙+) C33H26O4N2F4S2 654.1265 found 654.1261; FTIR (KBr, cm−1): 3046 (
C–H str), 2927 (–C–H str), 1604 (conjugated alkene C
C str), 1504 (Ar; C–C str), 1330 (O
S
O as-str), 1274 (C–F str), 1136 (O
S
O s-str), 972 (trans; C
C ben), 684 (cis C
C ben).
2.2.6 Synthesis of TCP4 (procedure B). To a stirring solution of TCP1 (50 mg, 0.12 mmol) in acetic acid (2.5 mL), was added dropwise 1 mL of a 30 wt% aqueous solution H2O2. The reaction mixture was stirred at 80 °C for 15 min. The precipitate was filtered off and washed with deionized water (20 mL) and diethyl ether (20 mL) then dried under vacuum to yield TCP4 as a pale-yellow solid (57 mg, 100%). 1H-NMR (600 MHz, DMSO-d6, ppm): δ 8.06–6.73 (bm, 10H, ArH & alkene-CH), 4.65–4.28 (m, 6H, methylene-CH), 2.49 (s, 6H, methyl-CH); FTIR (KBr, cm−1): 3039 (
C–H str), 2942 (–C–H str), 1613 (conjugated alkene C
C str), 1317 (O
S
O as-str), 1145 (O
S
O s-str), 991 (trans; C
C ben), 688 (cis C
C ben); UV-vis: (THF, 10−6 M), λmax [nm] = 297.
2.2.7 Synthesis of TCP5. TCP5 was prepared following procedure B with: TCP2 (50 mg, 0.1 mmol), acetic acid (2.5 mL), and 30 wt% of aq. H2O2 (1 mL). Pale-yellow solid (52 mg, 93%). 1H-NMR (600 MHz, DMSO-d6, ppm): δ 7.92–6.72 (bm, 14H, ArH & alkene-CH), 4.64–4.30 (m, 6H, methylene-CH), 2.49 (s, 6H, methyl-CH); FTIR (KBr, cm−1): 3050 (
C–H str), 2918 (–C–H str), 1605 (conjugated alkene C
C str), 1476 (Ar; C–C str), 1313 (O
S
O as-str), (1148 O
S
O s-str), 995 (trans; C
C ben), 819 (C–H ben), 722 (cis C
C ben); UV-vis: (THF, 10−6 M), λmax [nm] = 281.
2.2.8 Synthesis of TCP6. TCP6 was prepared following procedure B with: TCP3 (50 mg, 0.1 mmol), acetic acid (2.5 mL), and 30 wt% of aq. H2O2 (1 mL). Pale-yellow solid (53 mg, 95%). 1H-NMR (600 MHz, DMSO-d6, ppm): δ 7.64–6.57 (bm, 14H, ArH & alkene-CH), 4.60–3.98 (m, 6H, methylene-CH), 2.49 (s, 6H, methyl-CH); FTIR (KBr, cm−1): 3029 (
C–H str), 2918 (–C–H str), 1644 (conjugated alkene C
C str), 1501 (Ar; C–C str), 1311 (O
S
O as-str), 1138 (O
S
O s-str), 819 (C–H ben); UV-vis: (THF, 10−6 M), λmax [nm] = 271.
3. Results and discussion
3.1 Synthesis
The feasibility of the metal-free thiol-yne click reaction was tested by preparing the prototypical monomer TB-S from the reaction of diethynyl Trӧger's base TB with two equivalents of 3,4-difluorobenzenethiol 1 in THF at 50 °C, and which afforded the desired monomer in 73% yield (Scheme 1). The structure of TB-S was confirmed by 1H- and 13C-nuclear magnetic resonance (NMR), high-resolution mass spectroscopy (HRMS), and FTIR spectroscopy (see Fig. S1, S8, S15 and S17 in the ESI file†).
 |
| Scheme 1 Synthesis of monomer TB-S. | |
Synthesis of the Trӧger's base containing copolymers TCP1–3 (Scheme 2) was carried out following similar reaction conditions to those described above to make TB-S, but which were optimized by studying the effect of changing the medium temperature, reactants' concentration, and reaction time. Consequently, the four-day reaction of TB and 2a at room temperature (30 °C) afforded copolymer TCP1 with an average weight molar mass Mw ≈ 5.1 KDa and a polydispersity index Đ = 2.5. When the temperature of the reaction medium was raised to 50 °C, Mw increased to ∼8.6 kDa (Đ = 3.0). Nevertheless, a further increase of the reaction temperature to 70 °C led to a drop in the molar mass (Mw) of TCP1 to ∼7.5 kDa (Đ = 3.0, Table 1).
 |
| Scheme 2 Synthesis of copolymers TCP1–3. | |
Table 1 Effect of temperature on thiol-yne click polymerization reaction of TB and 2aa
Entry |
Product |
T (°C) |
Mw (g mol−1) |
Mn (g mol−1) |
Đ |
Reaction conditions: equimolar amount of TB (0.025 M) and 2a in THF. |
1 |
TCP1 |
30 |
5125 |
2050 |
2.5 |
2 |
TCP1 |
50 |
8675 |
2840 |
3.0 |
3 |
TCP1 |
70 |
7535 |
2485 |
3.0 |
The monomer's concentration effect on the copolymer chain growth was also investigated. Table 2 depicts that when the reactants' concentration in the medium is 5 × 10−2 M, a molar mass Mw of 6.6 kDa was recorded for TCP1 (Table 2, entry 1). The target copolymer TCP1 was obtained in a higher weight average molar mass, Mw, when the reactants' concentration in THF was diluted to 2.5 × 10−2 M (Table 2, entry 2). Further dilution of the reactants' concentration to 1 × 10−2 M afforded TCP1 with a lower Mw of 4.9 kDa.
Table 2 Effect of monomer concentration on polymerization reaction of TB and 2aa
Entry |
Product |
CM × 10−2 [M] |
Mw (g mol−1) |
Mn (g mol−1) |
Đ |
Reaction conditions: equimolar amount of TB and 2a in THF at 50 °C. |
1 |
TCP1 |
5.0 |
6655 |
1564 |
4.2 |
2 |
TCP1 |
2.5 |
8675 |
2840 |
3.0 |
3 |
TCP1 |
1.0 |
4900 |
1800 |
2.7 |
Table 3 summarizes the effect of changing the reaction time on TCP1 chain growth where a 60 minute reaction yielded the target copolymer with an average weight molar mass Mw = 5.6 kDa (Table 3, entry 1). The prolongation of the reaction time to six hours afforded TCP1 with Mw of 7.1 kDa (Table 3, entry 3) and a further duration extension to 24 hours increased Mw to ∼8.6 kDa (Table 3, entry 5). Nevertheless, when the reaction was left for three additional days, the target copolymer TCP1 was isolated in a lower yield () with reduced average molar mass values, which could be explained by the formation of insoluble copolymer networks when the reaction is left for a prolonged period (Table 3, entry 6).
Table 3 Effect of reaction time on thiol-yne click polymerization of TB and 2aa
Entry |
Product |
Time |
Mw (g mol−1) |
Mn (g mol−1) |
Đ |
Reaction conditions: equimolar amount of TB (0.025 M) and 2a in THF at 50 °C. |
1 |
TCP1 |
60 min |
5687 |
1820 |
3.1 |
2 |
TCP1 |
2 h |
6475 |
2290 |
2.8 |
3 |
TCP1 |
4 h |
7100 |
2595 |
2.7 |
4 |
TCP1 |
6 h |
7160 |
2450 |
2.9 |
5 |
TCP1 |
24 h |
8675 |
2840 |
3.0 |
6 |
TCP1 |
4 d |
7535 |
2485 |
3.0 |
Copolymers TCP2–3 were synthesized by applying the optimized reaction conditions described above to make TCP1. It is noteworthy that all the target copolymers TCP1–3 were obtained in very good yields (80–87%) and found to be highly soluble in common organic solvents, like, chloroform, dichloromethane, and tetrahydrofuran, which allowed for their structural characterization by instrumental analysis, namely, 1H- and 13C-NMR, gel permeation chromatography (GPC), FTIR, UV-vis and emission spectroscopy (see Fig. 2–4 and the related figures in the ESI file†).
Fig. 1 portrays the comparative 1H-NMR spectra of synthon TB and target copolymer TCP1. The chemical shifts of the terminal alkyne in the former, detected at 3.04 ppm, completely disappeared in the 1H-NMR spectrum of TCP1. In addition, the chemical shifts observed in the region ranging from 7.34 ppm to 6.35 ppm can be attributed to vinylic and aromatic protons whereas the protons located at 2.43 ppm are assigned to the methyl (–CH3) group. It is noteworthy that the proton of the thiol group detected at 3.39 ppm in the 1H-NMR spectrum of monomer 2a was not found in that of TCP1, and therefore, indicating the successful formation of the latter copolymer and complete consumption of both monomers TB and 2a (see Fig. 1 and S2 in the ESI file†).
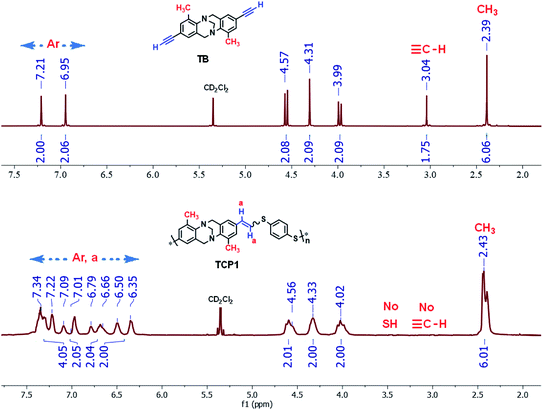 |
| Fig. 1 Comparative 1H-NMR of TB (up) and TCP1 (down). | |
Gel permeation chromatography (GPC) analyses of TCP1–3 reveal the formation of copolymers with a weight average molar mass Mw ranging from ∼4.3 kDa to ∼8.6 kDa with a polydispersity index (Đ = Mw/Mn) of 2.8–3.0 (Table 4).
Table 4 Summary of GPC results of copolymers TCP1–3a
Entry |
Co-monomers |
Copolymer |
Yield (%) |
Mw (g mol−1) |
Mn (g mol−1) |
Đ |
Reaction conditions: TB, [M] = 0.025 M, 50 °C, 24 hours. |
1 |
2a |
TCP1 |
85 |
8675 |
2840 |
3.0 |
2 |
2b |
TCP2 |
80 |
7796 |
2732 |
2.8 |
3 |
2c |
TCP3 |
87 |
4305 |
1502 |
2.8 |
Selective oxidation of the thioether groups into their corresponding sulfones was carried out using the prototypical monomer TB-S which underwent a reaction with hydrogen peroxide in acetic acid at 80 °C for 15 minutes (Scheme 3). The desired product TB-SO2 was isolated quantitatively by simple filtration from the reaction mixture and its formation was confirmed by EI-HRMS and FTIR absorption spectra (see Fig. S16 and S17 in the ESI file†).
 |
| Scheme 3 Synthesis of monomer TB-SO2. | |
Hence, selective oxidation reactions of the thioether groups of copolymers TCP1–3 into their corresponding sulfone moieties were carried out using the same reaction condition applied to make TB-SO2, which afforded the sulfone-bearing copolymers TCP4–6 (Scheme 4). These latter were found to be insoluble in common organic solvents (e.g. THF, DCM, and CHCl3) and were only sparingly soluble in polar aprotic solvents, namely, DMF and DMSO. The structures of TCP4–6 were confirmed by 1H-NMR, FTIR, UV-visible and emission spectroscopy (see Fig. 3, 4 and the related figures in the ESI file†).
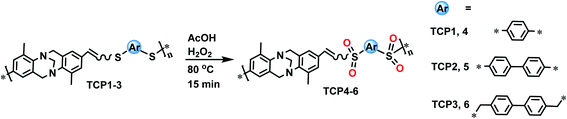 |
| Scheme 4 Synthesis of copolymer TCP4–6. | |
Fig. 2 divulges the comparative FTIR absorption spectra of the thioether containing copolymer TCP2 and its corresponding sulfonated compound TCP5. The asymmetric and symmetric stretching vibrations peaks of the sulfone (O
S
O) moiety in TCP5 were detected at 1313 cm−1 and 1148 cm−1, respectively. It is noteworthy that subtle shifts could be noticed in the stretching vibration peaks of the aromatic
C–H groups of TCP2 and TCP5, at 3021 cm−1 and 3050 cm−1, respectively. On the other hand, the stretching vibration peaks of the aliphatic –C–H groups of TCP2 and TCP5, were detected at 2941 cm−1 and 2918 cm−1, respectively. FTIR absorption spectra of TCP4–6 disclose their fingerprint functional groups' stretching and bending vibration peaks. The aromatic (
C–H) and aliphatic (–C–H) stretching vibrations were detected in the range of ∼3029–3050 cm−1 and 2918–2942 cm−1, respectively. Similarly, the absorption bands observed in the regions of 1605–1644 cm−1, 991–995 cm−1, and 688–722 cm−1, could be attributed to the characteristic trans and cis conjugated alkenes, respectively. Moreover, the distinctive asymmetric and symmetric stretching vibrations of sulfone (O
S
O) were identified in the range of 1311–1317 cm−1, and 1138–1148 cm−1, respectively. The typical aromatic C–C and C–H bending vibrations were also found at 1476–1501 cm−1 and ∼819 cm−1, respectively. It is worthwhile to note that the FTIR absorption peak of sulfoxide usually detected at ∼1030 cm−1 was not observed in any of the sulfonated copolymers TCP4–6 (see Fig. 2 and S18, S19 in the ESI file†), hence, confirming the complete oxidation of the thioethers moieties of TCP1–3 into their sulfone groups in TCP4–6.
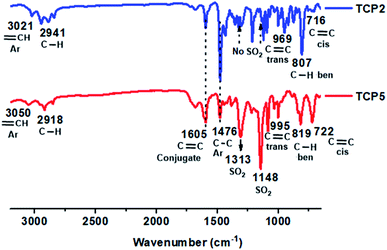 |
| Fig. 2 Comparative FT-IR spectra of TCP2 (blue) and TCP5 (red). | |
Fig. 3 portrays the UV-vis absorption and emission spectra of the thioether containing copolymers TCP1–3 and their corresponding sulfonated ones TCP4–6. TCP1 shows a strong UV absorption band detected at 325 nm but which is blue shifted to 297 nm for its sulfonated derivative TCP4. The same feature is encountered in copolymers TCP2,5 and their respective compounds TCP3,6 (see Fig. 3 and S20 in the ESI file†). The emission spectra of TCP1, which contains phenyl spacers between the thioethers, displays a broad spectrum with an intensity maximum at 395 nm whereas its corresponding sulfonated copolymer TCP4 illustrates a ∼19 nm bathochromic shift with an emission peak detected at 414 nm. Interestingly, copolymers TCP2 and TCP3, which contain biphenyl spacers, reveal emission maxima at 410 and 464 nm, respectively. Nevertheless, the sulfonated derivatives of these latter, namely, TCP5 and TCP6 disclose emission peaks at 324 and 326 nm, respectively, thus, revealing hypsochromic shifts of with respect to their synthons TCP2 and TCP3 by ∼86 and 138 nm, respectively (see Fig. 3 and S20 in the ESI file†).
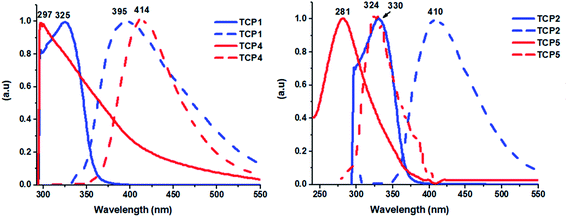 |
| Fig. 3 Normalized UV-vis absorption (CM = 10−7 M in THF, solid lines) and emission (CM = 10−8 M in THF, dotted lines) spectra of: (A) TCP1,4 (left) TCP2,5 (right) (absorption maxima were used as the excitation wavelengths). | |
3.2 Dye adsorption studies
The thioether containing copolymers were investigated as possible adsorbents of organic dyes (Fig. 4). Therefore, TCP1 was soaked in aqueous solutions of three anionic dyes, precisely, Congo red (CR), methyl orange (MO), and methyl blue (MB) in addition to methylene blue (MEB) which is cationic. The adsorption efficiency of TCP1 towards CR, MO, MB, and MEB from water was monitored by recording the UV-visible absorbance spectra of the aqueous solutions after the addition of the target copolymer at different time intervals. The dyes removal experiments were carried out by stirring at room temperature a 5 mL aliquot of a 5 mg L−1 aqueous solution of the hitherto mentioned dyes in presence of 5 mg of TCP1 in (Fig. 5).
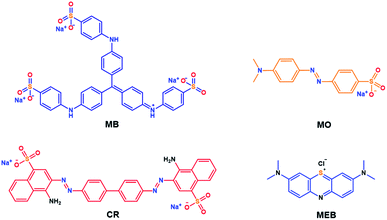 |
| Fig. 4 Structures of methyl blue (MB), methyl orange (MO), congo red (CR), and methylene blue (MEB). | |
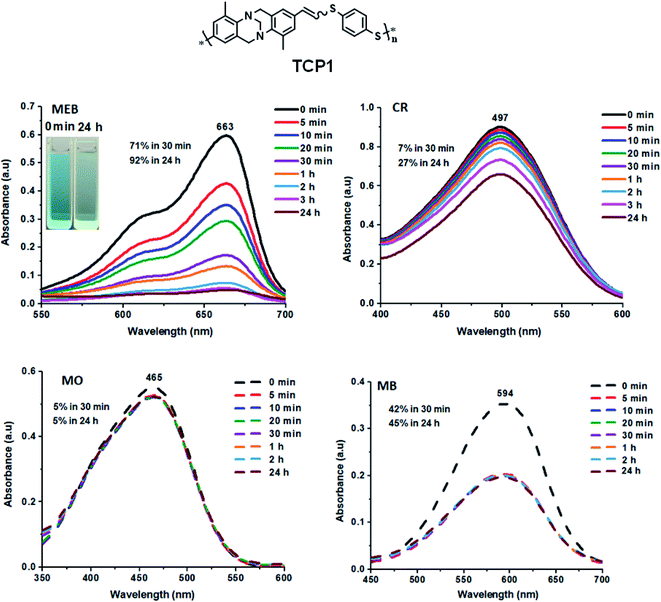 |
| Fig. 5 TCP1 adsorption of methylene blue (MEB), Congo red (CR), methyl orange (MO), and methyl blue (MB) at various time intervals (inset: photographs showing the color change upon dye adsorption). | |
A noticeable decrease in the absorbance intensity of MEB was observed, revealing a ∼71% removal efficiency after 30 minutes of TCP1 addition at room temperature, and which reached 92% after 24 h. On the other hand, TCP1 disclosed lower adsorption efficacies towards the anionic dyes CR, MO, and MB with 27%, 5%, and 45%, respectively (Fig. 5). Similar results were obtained when thioether containing copolymers TCP2–3 were tested as adsorbents.
Interestingly, sulfonated copolymers TCP4–6 displayed superior adsorption efficiencies towards MEB when compared to their corresponding thioether copolymer synthons TCP1–3 (see Fig. 6 and S21, S22 in the ESI file†). TCP1–3 revealed MEB ∼25–71% uptake efficiencies from water after 30 min which increased to ∼45–92% after 24 h. Nevertheless, the sulfonated copolymers TCP4–6 portrayed enhanced and faster MEB uptake efficacies ranging from ∼76% to 93% after 30 min and reached quantitative adsorption (∼100%) after 2-4 h (see Table S1 in the ESI file† which summarizes the dye adsorption properties of TCP1–6). The noticeable improvement in MEB dye adsorption properties for TCP4–6 could be explained by the presence of sulfone groups, which are more polar when compared to thioether units, hence, resulting in a more pronounced charge separation, and therefore, enhancing the electrostatic attraction between the adsorbent and adsorbates.66 Recyclability tests of the sulfonated copolymers to MEB were investigated using TCP6 as a model adsorbent which revealed a 100% efficiency for three successive cycles that slightly dropped to 96% uptake in the fourth cycle (see Fig. S23, in the ESI file†). It is noteworthy that, to the best of our knowledge, these results are either superior or equivalent to the adsorption capacities reported in the literature.67–71
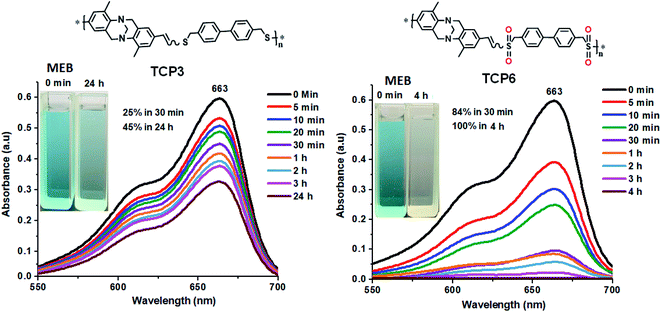 |
| Fig. 6 Methylene blue (MEB) dye adsorption by TCP3,6 at various time intervals (insets: photographs showing the color change upon dye adsorption). | |
4. Conclusion
Three novel poly(vinylene sulfone)s containing Trӧger's base units, TCP4–6, were synthesized in very good yields via a metal-free click thiol-yne reaction affording copolymers TCP1–3 and which underwent post-modification of their thioether units into sulfones using green oxidation reaction conditions. It is worthwhile to mention that copolymers TCP4–6 disclose fast and selective adsorption of methylene blue with uptake efficiencies reaching ∼93% after 30 minutes of adding the target materials to the aqueous solutions. The facile catalyst-free synthetic methodology to make these copolymers and their excellent selective dye adsorption properties promote them as potential materials for water treatment.
Data availability
The raw data required to reproduce these findings are available upon request.
Conflicts of interest
The authors declare no conflict of interests.
Acknowledgements
The project was partially supported by Kuwait Foundation for the Advancement of Sciences (KFAS) under project code: PN18-14SC-03 and P314-34SC-01. MS and SM would also like to thank KFAS (PR17-14SC-02) the general facilities projects GS01/03 and GS03/08 at Kuwait University for their support.
References
- Y. Kumar, S. Rani, J. Shabir and L. S. Kumar, ACS Omega, 2020, 5, 13250–13258 CrossRef CAS PubMed.
- N. U. M. Nizam, M. M. Hanafiah, E. Mahmoudi, A. A. Halim and A. W. Mohammad, Sci. Rep., 2021, 11, 8623 CrossRef CAS PubMed.
- B. Parmar, K. K. Bisht, G. Rajput and E. Suresh, Dalton Trans., 2021, 50, 3083–3108 RSC.
- A. Narula and C. P. Rao, ACS Omega, 2019, 4, 5731–5740 CrossRef CAS PubMed.
- S. Krishnan, S. Chatterjee, A. Solanki, N. Guha, M. K. Singh, A. K. Gupta and D. K. Rai, ACS Appl. Nano Mater., 2020, 3, 11203–11216 CrossRef CAS.
- J. Lin, F. Lin, X. Chen, W. Ye, X. Li, H. Zeng and B. van der Bruggen, Ind. Eng. Chem. Res., 2019, 58, 11003–11012 CrossRef CAS.
- S. Jain, S. Mishra and T. K. Sarma, ACS Sustainable Chem. Eng., 2018, 6, 9771–9783 CrossRef CAS.
- L. Cheng, M. Wei, L. Huang, F. Pan, D. Xia, X. Li and A. Xu, Ind. Eng. Chem. Res., 2014, 53, 3478–3485 CrossRef CAS.
- S. Shetty, N. Baig, A. Hassan, S. Al-Mousawi, N. Das and B. Alameddine, RSC Adv., 2021, 11, 14986–14995 RSC.
- N. Baig, S. Shetty, S. Al-Mousawi and B. Alameddine, Polym. Chem., 2021, 12, 2282–2292 RSC.
- J. Tröger, J. Prakt. Chem., 1887, 36, 225–245 CrossRef.
- Q. Qian, P. A. Asinger, M. J. Lee, G. Han, K. Mizrahi Rodriguez, S. Lin, F. M. Benedetti, A. X. Wu, W. S. Chi and Z. P. Smith, Chem. Rev., 2020, 120, 8161–8266 CrossRef CAS PubMed.
- K. Wang, K. Amin, Z. An, Z. Cai, H. Chen, H. Chen, Y. Dong, X. Feng, W. Fu, J. Gu, Y. Han, D. Hu, R. Hu, D. Huang, F. Huang, F. Huang, Y. Huang, J. Jin, X. Jin, Q. Li, T. Li, Z. Li, Z. Li, J. Liu, J. Liu, S. Liu, H. Peng, A. Qin, X. Qing, Y. Shen, J. Shi, X. Sun, B. Tong, B. Wang, H. Wang, L. Wang, S. Wang, Z. Wei, T. Xie, C. Xu, H. Xu, Z.-K. Xu, B. Yang, Y. Yu, X. Zeng, X. Zhan, G. Zhang, J. Zhang, M. Q. Zhang, X.-Z. Zhang, X. Zhang, Y. Zhang, Y. Zhang, C. Zhao, W. Zhao, Y. Zhou, Z. Zhou, J. Zhu, X. Zhu and B. Z. Tang, Mater. Chem. Front., 2020, 4, 1803–1915 RSC.
- A. L. Whiting, K. I. Dubicki and F. Hof, Eur. J. Org. Chem., 2013, 2013, 6802–6810 CrossRef CAS.
- R. B. P. Elmes, M. Erby, S. A. Bright, D. C. Williams and T. Gunnlaugsson, Chem. Commun., 2012, 48, 2588–2590 RSC.
- E. B. Veale, D. O. Frimannsson, M. Lawler and T. Gunnlaugsson, Org. Lett., 2009, 11, 4040–4043 CrossRef CAS PubMed.
- Z. Zhu, J. Zhu, J. Li and X. Ma, Macromolecules, 2020, 53, 1573–1584 CrossRef CAS.
- C. Y. Chuah, K. Goh, Y. Yang, H. Gong, W. Li, H. E. Karahan, M. D. Guiver, R. Wang and T.-H. Bae, Chem. Rev., 2018, 118, 8655–8769 CrossRef CAS PubMed.
- E. Madrid, Y. Rong, M. Carta, N. B. McKeown, R. Malpass-Evans, G. A. Attard, T. J. Clarke, S. H. Taylor, Y.-T. Long and F. Marken, Angew. Chem., Int. Ed., 2014, 53, 10751–10754 CrossRef CAS PubMed.
- T. Zhang, L. Deng and P. Li, Ind. Eng. Chem. Res., 2020, 59, 18640–18648 CrossRef CAS.
- M. Carta, R. Malpass-Evans, M. Croad, Y. Rogan, J. C. Jansen, P. Bernardo, F. Bazzarelli and N. B. McKeown, Science, 2013, 339, 303–307 CrossRef CAS PubMed.
- H. Dou, M. Xu, B. Wang, Z. Zhang, G. Wen, Y. Zheng, D. Luo, L. Zhao, A. Yu, L. Zhang, Z. Jiang and Z. Chen, Chem. Soc. Rev., 2021, 50, 986–1029 RSC.
- X. Ma, Z. Zhu, W. Shi, W. Ji, J. Li, Y. Wang and I. Pinnau, J. Mater. Chem. A, 2021, 9, 5404–5414 RSC.
- S.-L. Li, Z. Zhu, J. Li, Y. Hu and X. Ma, Polymer, 2020, 193, 122369 CrossRef CAS.
- Z.-X. Low, P. M. Budd, N. B. McKeown and D. A. Patterson, Chem. Rev., 2018, 118, 5871–5911 CrossRef CAS PubMed.
- N. B. McKeown, Nat. Mater., 2016, 15, 706–707 CrossRef CAS PubMed.
- I. Rose, M. Carta, R. Malpass-Evans, M.-C. Ferrari, P. Bernardo, G. Clarizia, J. C. Jansen and N. B. McKeown, ACS Macro Lett., 2015, 4, 912–915 CrossRef CAS.
- R. Williams, L. A. Burt, E. Esposito, J. C. Jansen, E. Tocci, C. Rizzuto, M. Lanč, M. Carta and N. B. McKeown, J. Mater. Chem. A, 2018, 6, 5661–5667 RSC.
- M. Carta, M. Croad, R. Malpass-Evans, J. C. Jansen, P. Bernardo, G. Clarizia, K. Friess, M. Lanč and N. B. McKeown, Adv. Mater., 2014, 26, 3526–3531 CrossRef CAS PubMed.
- X. Ma, H. W. H. Lai, Y. Wang, A. Alhazmi, Y. Xia and I. Pinnau, ACS Macro Lett., 2020, 9, 680–685 CrossRef CAS.
- R. Swaidan, B. Ghanem and I. Pinnau, ACS Macro Lett., 2015, 4, 947–951 CrossRef CAS.
- B. S. Ghanem, R. Swaidan, X. Ma, E. Litwiller and I. Pinnau, Adv. Mater., 2014, 26, 6696–6700 CrossRef CAS PubMed.
- W. Li and T. Michinobu, Macromol. Chem. Phys., 2016, 217, 863–870 CrossRef CAS.
- S. Shanmugaraju, D. Umadevi, A. J. Savyasachi, K. Byrne, M. Ruether, W. Schmitt, G. W. Watson and T. Gunnlaugsson, J. Mater. Chem. A, 2017, 5, 25014–25024 RSC.
- V. P. Jejurkar, G. Yashwantrao and S. Saha, New J. Chem., 2020, 44, 12331–12342 RSC.
- H. C. Kolb, M. G. Finn and K. B. Sharpless, Angew. Chem., Int. Ed., 2001, 40, 2004–2021 CrossRef CAS PubMed.
- O. Konuray, X. Fernández-Francos, S. De la Flor, X. Ramis and À. Serra, Polymers, 2020, 12, 1084 CrossRef CAS PubMed.
- B. Alameddine, N. Baig, S. Shetty, S. Al-Mousawi and F. Al-Sagheer, Polymer, 2018, 154, 233–240 CrossRef CAS.
- A. Marrocchi, A. Facchetti, D. Lanari, S. Santoro and L. Vaccaro, Chem. Sci., 2016, 7, 6298–6308 RSC.
- J.-P. Meyer, P. Adumeau, J. S. Lewis and B. M. Zeglis, Bioconjugate Chem., 2016, 27, 2791–2807 CrossRef CAS PubMed.
- J. C. Worch, C. J. Stubbs, M. J. Price and A. P. Dove, Chem. Rev., 2021 DOI:10.1021/acs.chemrev.0c01076.
- S. Nayab, V. Trouillet, H. Gliemann, P. G. Weidler, I. Azeem, S. R. Tariq, A. S. Goldmann, C. Barner-Kowollik and B. Yameen, Inorg. Chem., 2021, 60, 4397–4409 CrossRef CAS PubMed.
- J. Sinha, M. Podgórski, A. Tomaschke, V. L. Ferguson and C. N. Bowman, Macromolecules, 2020, 53, 6331–6340 CrossRef CAS.
- J. Huang, H. Su, M. Bao, L. Qiu, Y. Zhang and X. Xu, Org. Biomol. Chem., 2020, 18, 3888–3892 RSC.
- S. K. Perala and S. Ramakrishnan, Polym. Chem., 2019, 10, 1626–1635 RSC.
- G. H. Lonca, C. Tejo, H. L. Chan, S. Chiba and F. Gagosz, Chem. Commun., 2017, 53, 736–739 RSC.
- D. Funes-Hernando, P. Hermosilla, E. Vispe, A. Di Giuseppe, R. Castarlenas, L. A. Oro and J. J. Pérez-Torrente, Polym. Chem., 2018, 9, 1298–1302 RSC.
- S. S. Zalesskiy, N. S. Shlapakov and V. P. Ananikov, Chem. Sci., 2016, 7, 6740–6745 RSC.
- A. B. Lowe, Polymer, 2014, 55, 5517–5549 CrossRef CAS.
- J. Du, D. Huang, H. Li, A. Qin, B. Z. Tang and Y. Li, Macromolecules, 2020, 53, 4932–4941 CrossRef CAS.
- B. Yao, T. Hu, H. Zhang, J. Li, J. Z. Sun, A. Qin and B. Z. Tang, Macromolecules, 2015, 48, 7782–7791 CrossRef CAS.
- B. Yao, J. Mei, J. Li, J. Wang, H. Wu, J. Z. Sun, A. Qin and B. Z. Tang, Macromolecules, 2014, 47, 1325–1333 CrossRef CAS.
- E. Caron and M. O. Wolf, Macromolecules, 2017, 50, 7543–7549 CrossRef CAS.
- N. Jürgensen, A. Kretzschmar, S. Höfle, J. Freudenberg, U. H. F. Bunz and G. Hernandez-Sosa, Chem. Mater., 2017, 29, 9154–9161 CrossRef.
- T. Zhang, Y. Du, F. Müller, I. Amin and R. Jordan, Polym. Chem., 2015, 6, 2726–2733 RSC.
- J. A. van Hensbergen, T. W. Gaines, K. B. Wagener, R. P. Burford and A. B. Lowe, Polym. Chem., 2014, 5, 6225–6235 RSC.
- P. Kallem, N. Yanar and H. Choi, ACS Sustainable Chem. Eng., 2019, 7, 1808–1825 CrossRef CAS.
- J. Kosai, Y. Masuda, Y. Chikayasu, Y. Takahashi, H. Sasabe, T. Chiba, J. Kido and H. Mori, ACS Appl. Polym. Mater., 2020, 2, 3310–3318 CrossRef CAS.
- A. Hirose, K. Tanaka, R. Yoshii and Y. Chujo, Polym. Chem., 2015, 6, 5590–5595 RSC.
- G. Li, C. Zhao, X. Li, D. Qi, C. Liu, F. Bu and H. Na, Polym. Chem., 2015, 6, 5911–5920 RSC.
- S. Yuan, J. Wang, X. Li, J. Zhu, A. Volodine, X. Wang, J. Yang, P. Van Puyvelde and B. Van der Bruggen, J. Membr. Sci., 2018, 549, 438–445 CrossRef CAS.
- C. A. Jeffs, M. W. Smith, C. A. Stone, C. G. Bezzu, K. J. Msayib, N. B. McKeown and S. P. Perera, Microporous Mesoporous Mater., 2013, 170, 105–112 CrossRef CAS.
- Y. Matsumoto and A. Takasu, Polym. J., 2018, 50, 187–196 CrossRef CAS.
- R. J. Reddy and A. H. Kumari, RSC Adv., 2021, 11, 9130–9221 RSC.
- B. Dolenský, J. Elguero, V. Král, C. Pardo and M. Valík, in Advances in Heterocyclic Chemistry, ed. A. R. Katritzky, Academic Press, 2007, vol. 93, pp. 1–56 Search PubMed.
- B. M. Thamer, A. Aldalbahi, M. Moydeen, M. Rahaman and M. H. El-Newehy, Polymers, 2021, 13, 20 CrossRef CAS PubMed.
- K. Tiwari, P. Sarkar, S. Modak, H. Singh, S. K. Pramanik, S. Karan and A. Das, Adv. Mater., 2020, 32, 1905621 CrossRef CAS PubMed.
- S. Biswas, T. U. Rashid, T. Debnath, P. Haque and M. M. Rahman, J. Compos. Sci., 2020, 4, 16 CrossRef CAS.
- M. Yan, W. Huang and Z. Li, Int. J. Biol. Macromol., 2019, 136, 927–935 CrossRef CAS PubMed.
- Q. Jin, Y. Li, D. Yang and J. Cui, RSC Adv., 2018, 8, 1255–1264 RSC.
- T. M. Budnyak, S. Aminzadeh, I. V. Pylypchuk, D. Sternik, V. A. Tertykh, M. E. Lindström and O. Sevastyanova, J. Environ. Chem. Eng., 2018, 6, 4997–5007 CrossRef CAS.
Footnote |
† Electronic supplementary information (ESI) available. See DOI: 10.1039/d1ra03783h |
|
This journal is © The Royal Society of Chemistry 2021 |
Click here to see how this site uses Cookies. View our privacy policy here.