DOI:
10.1039/D1RA03274G
(Paper)
RSC Adv., 2021,
11, 30520-30531
Effect of simulated saliva components on the in vitro digestion of peanut oil body emulsion
Received
27th April 2021
, Accepted 29th August 2021
First published on 14th September 2021
Abstract
The digestion properties of natural oil bodies (OBs) are very important to their potential applications such as traditional fat replacement or bioactive delivery systems. However, study on the complete digestion behaviours of OBs has not been reported yet. In this paper, peanut OBs were extracted by an aqueous medium method, and their digestion behaviour was studied using completed in vitro oral-gastric-intestinal digestion simulation. In particular, the effects of saliva components, mainly α-amylase and mucin, on the digestion of the peanut OBs were systematically investigated. The OB emulsion microstructure, average particle size d4,3, ζ-potential, and surface protein compositions during oral, gastric and intestinal digestion, and the free fatty acid (FFA) release rate of the peanut OBs during intestinal digestion were determined. Interestingly, it was revealed from both the periodic acid-Schiff staining technique and the confocal laser microscopy characterization that glycosidic bonds exist on the surface of the peanut OBs, though how they were produced was unknown. The results from the digestion measurements showed that α-amylase in saliva can break the glycosidic bonds in oral digestion, promoting the digestion of the OBs in the gastric and intestinal environments. Saliva mucin caused bridging flocculation of OBs by electrostatic attraction in the gastric tract, and depletion flocculation of OBs in the intestinal tract. The former hindered the fusion of oil droplets, and the latter promoted FFA release rate by increasing the contacting surface area of OBs with bile salts.
1. Introduction
Oil bodies (OBs), also called oleosomes, are micron- or submicron-sized organelles found mainly in plant seeds and nuts, as well as other parts such as leaves, bulbs and fruits.1 The biogenesis of OBs is a complex process and is initiated by the synthesis of triglyceride (TAG) in the endoplasmic reticulum of the cells of oil-bearing plant tissues.2 The structure of the OBs is mainly composed of a core of TAG, and the surrounding half-unit phospholipid membrane embedded with OB surface proteins.3,4 Depending on the sources, TAGs comprise about 94.0–98.0% (w/w), phospholipids (PLs) 0.6–2.0%, and proteins occupy 0.6–3.0%.5 The main surface proteins of OBs are amphiphilic proteins mainly including oleosin, caleosin and steroleosin, with oleosins being the dominant ones. Oleosin (15–26 kDa) contains 3 domains: the highly hydrophobic proline knot hairpin structure extending into the central domain of TAGs, the hydrophilic C-terminal located on the surface of OBs, and the hydrophilic N-terminal facing the cytoplasm.5 Caleosin (25–35 kDa) and steroleosin (40 kDa) have similar structures to oleosin, but the hydrophobic domains are shorter and the hydrophilic domains are longer. Oleosins and caleosins are believed to be the main structural proteins of OBs.6 There are also some other interactions or structures on the surface of the OBs, such as disulfide bonds between surface proteins, and covalent interaction between protein/phospholipid with glycosyl groups,7,8 which also contribute to the structure of OBs, although the effect of these interactions or groups on the function of oleosomes are not fully understood.2
The OBs can be extracted by the aqueous medium method through soaking, crushing, filtering and centrifugation.5 As-extracted OBs are in the form of aqueous creams or emulsions, which are naturally emulsified without the addition of other surfactants or chemicals, and nutrients such as fat-soluble vitamin E and unsaturated fatty acids, which are the naturally predominant components of the TAG molecules, can be completely preserved. Moreover, the OBs in emulsions extracted in this way have a relatively complete structure and are stable to external physical and chemical disturbances. It is believed that the physical stability of the OB emulsions were due to the protection by the charged layers formed by the surface proteins and phospholipids, as well as the steric hindrance provided by the structure proteins of the OBs.1,9,10 On the other hand, the chemical stability of the OBs, particularly the stability against oxidative stresses is due to the protective effect by oleosins, acting as a barrier to oxidizing agents such as oxygen and hydroperoxides,11 the high amounts of naturally present antioxidants such as carotenoids and tocopherols in OBs,12 and the small peptides released during OB digestion.13 Therefore, due to the unique structure and compositions, safe and energy-saving extraction process, richness in nutrients, and relatively good physicochemical stability, OBs and their aqueous emulsions have received extensive attention. OBs can be potentially used as alternatives to the traditional fat in food design such as beverage development, edible films, coatings, salad dressings, or other products and delivery systems of bioactive compounds.2,14–17
In food design, an interesting and important problem is the fat digestion of the OBs, since it strongly affects the product quality and their functions, such as the bioaccessibility and bioavailability of nutrients and bioactive compounds.2,18–20 Consequently, researchers are interested in how the interface structure affects the digestion behaviours of the OBs.18–22 On the other hand, the process of digestion of OBs is complex, occurring in a sequence of operations including mouth, gastric, and intestine.23 It starts with the mastication in the mouth, which breaks the structure of the food into smaller particles and mixes them thereafter, but it was reported that this force is insufficient to disintegrate seed matrix and hence interfere with the gastrointestinal digestion of the OBs was not found.24–27 In the gastrointestinal digestion, the behaviour of OB emulsions is expected to be similar to that of protein-stabilized emulsions as their surfaces are entirely covered with oleosins,2 and researchers suggested that the structure of protein–phospholipid membrane of OBs may slow down the digestion of oil and thus increase the feeling of fullness.18,19 However, most of the currently used in vitro digestion experiments are not complete, and failed to elucidate the physicochemical changes in OB emulsions after oral process but prior to their digestion in the gastric.2 Particularly, the influence of saliva components on the digestion of OBs has been ignored, which should not be, since saliva components in the oral cavity such as anionic polymer mucin, α-amylase, ions, etc., will cause a change in the structure of emulsions which in turn affects the functional properties of the OBs when used in the food formulation.28–36
Based on these observations, in this study, the peanut OBs were extracted using the pH-controlled aqueous medium method, and the complete in vitro simulated digestion of the peanut OB emulsion starting from the oral cavity was investigated. Effects of saliva components, particularly the α-amylase and mucin, on the behaviour of peanut OBs during oral digestion and the following gastrointestinal digestion were systematically investigated. Most interestingly, we found and proved the existence of glycosidic bonds on the surface of the extracted peanut OBs using different methods, and the effect of them on the digestion of the peanut OBs was revealed.
2. Materials and methods
2.1. Materials
Mucin from porcine gastric (with molecular weight (Mw) of 4000–5500 kDa), pancreatin (activity 4000 U mg−1), Nile red and Nile blue were purchased from Sigma Chemical Co. (St. Louis, MO, USA). Pepsin (activity 3000 U mg−1) and trichloroacetic acid were purchased from Macklin (Shanghai, China). α-Amylase was bought from Shuangxuan Microbe Medium Products Plant (Beijing, China). Lectin Alexa Fluor® 488 conjugate of concanavalin A (Con A, λex: 490 nm, λem: 520 nm, 1 mg mL−1 in 0.1 M sodium bicarbonate buffer, pH 8.3, 1
:
20 v/v) were bought from Thermo Fisher Scientific (Waltham, MA, USA). Other chemicals were of analytical grade and purchased from Sinopharm Chemical Reagent Co. Ltd (Beijing, China). Ultra-pure water was used for the preparation of all solutions.
2.2. Extraction of peanut OBs and composition characterization
The peeled peanuts were soaked in distilled water (1
:
5, w/v) for 18 h at 4 °C. The soaked peanuts were then blended in buffer solution (50 mM Tris–HCl, pH 7.5) containing 0.4 M sucrose and 0.5 M NaCl for 180 s by a commercial food processor (KS-520, Guangzhou City Electric Appliance Co, Ltd, China) to obtain a homogenate of peanuts. The pH of the buffer solution was adjusted using 2 M HCl. The homogenate was filtered through three layers of cheesecloth, and then the filtrate was centrifuged at 10
000×g, 4 °C for 30 min (Heraeus Multifuge X1/X1R, Thermo Fisher Scientific, USA). The upper cream layer of the centrifugate were collected and re-dispersed in the sodium phosphate buffer solution (PBS, 50 mM, pH 7) and centrifuged again as above. Then the collected product was dispersed in 8 M urea solution (1
:
5, w/v) at 25 °C with magnetic stirring for 1 h. The mixture was then washed with the buffer solution again (50 mM Tris–HCl buffer, pH 7.5) for another three times. The final centrifuged and collected creams are the peanut OBs extracts.37,38
The composition including the moisture, the fat content, and the protein content of the extracted peanut OB creams was determined according to AOAC method.39 The moisture content of the peanut OBs was determined by 100 °C oven drying. The fat content of the peanut OBs was determined by the Soxhlet extractor system using petroleum ether as the extraction solvent. For the protein content measurement, nitrogen content of the peanut OBs was first determined by the Kjeldahl method.40 Then the 5.46 Kjeldahl N conversion factor was used to convert percentage of nitrogen to protein content.41
2.3. Emulsion preparation and characterization
The peanut OB emulsions with oil content of 10% (w/w) were prepared by dispersing the OB cream into PBS (50 mM, pH 7), and then magnetic stirring for 30 min.40,42 Droplet size and size distribution of the peanut OB emulsions were determined with the aid of a laser light scattering instrument (Malvern Mastersizer 2000, UK). The refractive index of dispersed phase and continuous phase was 1.47 and 1.33, respectively. The ζ-potential of the droplets in the emulsion was calculated by measuring the electrophoretic mobility of the droplets using a capillary electrophoresis cell (Zetasizer Nano ZS series, Malvern Instruments, Worcestershire, UK). During the measurement, the emulsion sample was diluted 100-fold, and the ζ-potential of the emulsion droplets was determined by measuring the actual electrophoretic mobility UE of charged particles via laser Doppler velocimetry at an angle of 173° according to the equation ζ = 3ηUE/2εf(Ka), where ε is the dielectric constant, η is the viscosity of the medium, and f(Ka) is the Henry function, which is close to 1.5 under the Smoluchowski approximation (refractive index 1.47, dispersant index 1.33, temperature 25 °C, viscosity 0.8872 cP). All measurements were performed at 25 °C and repeated three times. The microstructure of the OB emulsions were characterized using an optical microscope (Olympus IX73, Olympus Corporation, Tokyo, Japan).
2.4. Characterization of fatty acid composition of peanut OBs
In order to estimate the Mw of the triglyceride of peanut OBs, which is needed in the fat digestion experiment, the fatty acid composition of the peanut OB fat was determined by the Nash method.43 The fat in the peanut OBs was first extracted with ether, and then 20 mg of fat sample, 3 mL of n-hexane solution, and 0.5 mL potassium hydroxide–methanol solution (2 M) were loaded in the esterification flask, and the methyl esterification operation was carried out by shaking. Then 1 g of anhydrous sodium sulfate was added to the flask, and the upper layer liquid was filtered through the membrane and injected into the high-performance gas chromatography (7820A, Agilent, US) to characterize the fatty acid composition. Chromatographic column (TG-POLAR 26082-5030, Thermal Scientific) with dimension of 105 m × 0.25 mm × 0.2 μm was used. Injection was done at 270 °C, and temperature of the detector was 280 °C. Temperature was programmed to rise from initial temperature of 100 °C to 180 °C first at a speed of 10 °C min−1, and hold for 6 min at 180 °C; then rise from 180 °C to 200 °C at a speed of 1 °C min−1, and hold for 20 min at 200 °C; finally from 200 °C to 230 °C at a speed of 4 °C min−1, and holding for 10.5 min at 230 °C.
2.5. Characterization of surface proteins of peanut OBs
The composition of the peanut OB surface proteins was analyzed by sodium dodecyl sulfate polyacrylamide gel electrophoresis (SDS-PAGE) method described by Su et al.40 but with slight modification. The peanut OB emulsion with oil content of 10 wt% was used as the initial sample to mix with the SDS buffer directly. The following treatment is the same with Su et al. After electrophoresis, the gel was stained with 0.025% (w/v) Coomassie blue G-250 in 10% (v/v) acetic acid overnight, and destained by methanol in acetic acid and deionized water with 3
:
1
:
6 (v/v/v).
2.6. Characterization of glycosylation of the OBs
Con A staining of glycosidic bonds was first used to determine whether glycosylation exist on the surface of the extracted peanut OBs: 400 μL of each OB emulsion sample (with oil content of 10 wt%) and 20 μL of 1 mg mL−1 Con A (in 0.1 M sodium bicarbonate buffer, pH 8.3, 1
:
20 v/v) were used for staining glycosidic bonds because Con A can selectively bind to the α-mannopyranosyl and α-glucopyranosyl residues on proteins or lipids which results in a green fluorescent complex.7 The microstructure of the Con A stained OB emulsion sample was characterized by Confocal Laser Scanning Microscopy (CLSM).
The periodic acid-Schiff (PAS) technique was then used to determine the existence of glycoproteins on the surface of the OBs according to the method following electrophoresis on acrylamide gels.44 A piece of the acrylamide gel after electrophoresis of the proteins was soaked with 10% trichloroacetic acid for protein fixation for 25 min, and rinsed with distilled water for 2–3 times. Then the gel was soaked with 1% periodic acid for 15 minute oxidation reaction, and rinsed with distilled water for 2–3 times. After this, the gel was soaked with the Schiff reagent and stained for 1 hour in dark. The Schiff reagent was prepared as follows: 1 g basic Fuchsin was dissolved in 200 mL boiling water, and then cooled to 40–50 °C; 1.37 g sodium metabisulfite, 20 mL 1 M HCl, and 30 mL of activated carbon were then added under stirring until the solution became colorless; then the activated carbon was filtered and the filtrate was used as the Schiff reagent. After staining, the gel was washed with 0.5% sodium metabisulfite, and finally stored within acetic acid solution. The PAS-treated gel was finally compared with the one untreated.
2.7. In vitro digestion
A three-stage digestion model consisting of mouth, gastric, and small intestinal phases was used to simulate the conditions of human digestion.45 The compositions of the simulated digestion solutions at each stage are shown in Table 1.
Table 1 The compositions of the simulated digestion solutionsa
Composition |
SSF |
SGF (mg mL−1) |
SIF (mg mL−1) |
As (mg mL−1) |
As + α-amylase (mg mL−1) |
As + mucin (mg mL−1) |
As + α-amylase + mucin (mg mL−1) |
As, simulated oral digestive mother solution; SSF, simulated saliva fluid; SGF, simulated gastric fluid; SIF, simulated intestinal fluid. |
Sodium chloride |
1.594 |
1.594 |
1.594 |
1.594 |
2.0 |
43.740 |
Ammonium nitrate |
0.328 |
0.328 |
0.328 |
0.328 |
— |
— |
Potassium hydrogen phosphate |
0.636 |
0.636 |
0.636 |
0.636 |
— |
— |
Potassium chloride |
0.202 |
0.202 |
0.202 |
0.202 |
— |
— |
Potassium citrate |
0.308 |
0.308 |
0.308 |
0.308 |
— |
— |
Urea |
0.198 |
0.198 |
0.198 |
0.198 |
— |
— |
Sodium lactate |
0.146 |
0.146 |
0.146 |
0.146 |
— |
— |
α-Amylase |
— |
0.600 |
— |
0.600 |
— |
— |
Mucin |
— |
— |
3 |
3 |
— |
— |
Hydrochloric acid |
— |
— |
— |
— |
0.007 |
— |
Pepsin |
— |
— |
— |
— |
3.2 |
— |
Calcium chloride dihydrate |
— |
— |
— |
— |
— |
7.340 |
Bile salt |
— |
— |
— |
— |
— |
2.330 |
Pancreatin |
— |
— |
— |
— |
— |
0.533 |
2.7.1. Oral digestion. In order to investigate the effect of the main saliva compositions on the digestion of the peanut OBs, the SSF (see Table 1 for its composition) was prepared with and without α-amylase or mucin as indicated by As, As + α-amylase, As + mucin, or As + α-amylase + mucin in Table 1, respectively. Each of 7.5 mL of the OB emulsion (with oil of 10 wt%) was mixed with 7.5 mL of the SSF in an incubated shaker, with pH adjusted to 6.8 using 1 M HCl and NaOH. Then the mixed samples were digested for 10 min, and taken out for further characterization or the following gastric digestion.
2.7.2. Gastric digestion. 15 mL of SGF (see Table 1 for its composition) was added to each OB emulsion sample (15 mL) after oral digestion for 10 min. The pH of the mixed system was quickly adjusted to 2.5 with 1 M HCl, and the digestion was carried out in a constant temperature water bath at 37 °C for 2 h.
2.7.3. Intestinal digestion. After the gastric digestion was completed, the pH of the mixed system was quickly adjusted to 7.0 with 1 M NaOH. 7.5 mL of SIF (see Table 1 for its composition) were added to the gastric digested OB emulsion sample (30 mL) with the pH of the mixed system adjusted to 7 with 1 M sodium hydroxide again, and then the sample system was digested in a constant temperature water bath at 37 °C for 2 h.The release rate of free fatty acid (FFA) hydrolyzed from the oil in the peanut OB emulsion under the action of lipase in the intestinal tract was measured following the pH-stat method by Mun et al.46 The total volume of the digestion solution was 37.5 mL. The pH in the reaction vessel was maintained at 7.0 adjusted with 0.1 M NaOH. By counting the volume of NaOH solution used for titrating, the FFA content released during digestion is calculated as:46
where the meanings of the symbols are:
VNaOH, the volume of NaOH solution consumed by neutralizing FFAs (mL);
CNaOH, the molar concentration of NaOH used (mol L
−1);
WLipid, the mass of initial oil in the digestive reaction (mg);
MLipid, the molar mass of triglyceride in peanut OBs (g mol
−1).
2.7.4. Characterization of peanut OBs emulsion at each digestion stage. Since the main function of α-amylase in the saliva is the enzymatic digestion of carbohydrates, although the highly glycosylated mucin mainly plays a lubricating role in the oral cavity,29–36 we should first characterize how the surface proteins of the peanut OBs change after treatment by the simulated saliva fluid and how does this affect the structure of OBs in the following gastric digestion stage. Therefore the proteins of the products of the peanut OB emulsion after oral digestion and during gastric digestion were characterized using SDS-PAGE.The droplet size and size distribution, and the ζ-potential of the peanut OB emulsion samples at different digestion stages were also characterized respectively by the Malvern Mastersizer 2000 and Zetasizer Nano ZS series as mentioned before. The peanut OB emulsions were diluted 100-fold in ultra-pure water, the gastric digested samples were diluted 100-fold in a 10 mM citrate buffer (pH 2.5), and the intestinal digested samples were diluted 100-fold in phosphate buffer solution (pH 7.0).20 The microstructure of the digested OB emulsions was characterized by CLSM. Nile blue (λex: 631 nm, λem: 660 nm) was used to label proteins, and Nile red (λex: 549 nm, λem: 628 nm) to label lipids. At the end of the gastric or intestinal digestion stage, 1 mL of the digested OB emulsion sample was added with 40 μL prepared staining solution for 10 min. Then the stained samples were imaged using CLSM under 60× oil lens.
2.8. Statistical analysis
Each sample was prepared at least three times, and each measurement was repeated at least in triplicates. The statistics were calculated to obtain the averaged value with the standard deviation.
3. Results and discussion
3.1. Structure and compositions of the extracted peanut OBs
Fig. 1a and b show the microstructure and size distribution of the extracted peanut OBs. It can be seen that the peanut OBs are unimodally distributed with averaged particle size d4,3 of 2.55 ± 0.05 μm. From Fig. 1c we can find that the isoelectric point (IEP) of the OB emulsion sample is around pH 4.7 (the pH of each single point in Fig. 1c was obtained by adjusting the pH of the OB emulsion from pH 8 to pH 2 using acid/base, and then ζ-potential was measured at each specific pH during this downward pH change).
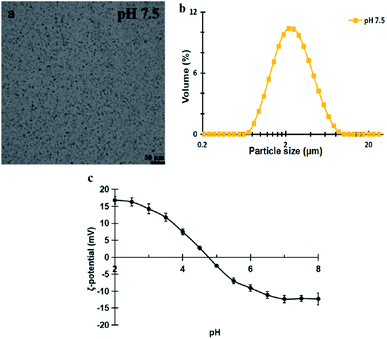 |
| Fig. 1 The microstructure and surface properties of the extracted peanut OBs. (a) Microstructure image from optical microscopy; (b) particle size and size distribution from light scattering measurement; (c) ζ-potential. | |
The moisture content of the peanut OB cream was measured to be about 18.2 ± 0.1% (w/w), and the fat content is about 78.6 ± 0.4% (w/w), and the protein content about 0.646 ± 0.002% (w/w), respectively. Each measured parameter is determined independently, so that the protein content, oil content, and water content do not sum up to 100%. The fatty acids of the extracted peanut OBs were mainly long-chain unsaturated fatty acids of oleic acid and linoleic acid as shown in Table 2. Therefore, the averaged Mw of the triglyceride in peanut OBs can be calculated by their mass weighting, which is 278.25 ± 0.10 g mol−1.
Table 2 Compositions of the peanut OB fatty acids
Types of fatty acids |
Content (wt%) |
Palmitic acid (C16:0) |
13.31 ± 0.05 |
Oleic acid (C18:1n9c) |
49.51 ± 0.02 |
Linoleic acid (C18:2n6c) |
37.18 ± 0.03 |
3.2. Effects of saliva components on oral digestion of peanut OBs
3.2.1. Change of OB surface proteins after oral digestion. After the peanut OBs were digested in the oral stage by the simulated saliva fluid SSF with different components, As, As + α-amylase, As + mucin, or As + α-amylase + mucin as listed in Table 1, the changes of the surface proteins of peanut OBs were characterized using SDS-PAGE first, and the results are shown in Fig. 2a–c. For comparison, the surface protein compositions of the undigested peanut OBs were also characterized. As can be seen (Lane 5 in Fig. 5a and b, and Lane 4 in Fig. 5c), the undigested peanut OB surface contains mainly the endogenous proteins, and some residual exogenous proteins, the similar to what we found previously.40 The endogenous proteins of the extracted peanut OBs include oleosins with Mw of 16–18 kDa, caleosins of 25 and 30 kDa, and steroleosins of 40 kDa. The exogenous proteins are mainly those with Mw greater than 41 kDa.14 However, the compositions of the simulated saliva has obvious effects on the surface proteins of the digested peanut OBs. As shown in Fig. 2a, when the saliva only contained As, the protein bands of peanut OBs hardly changed after they were digested for 1 or 10 min (Lane 1 and 2 respectively in Fig. 2a), compared with the undigested OBs (Lane 5). For comparison, Lane 3 and Lane 4 in Fig. 2a are the electrophoresis of As itself and the marker proteins, respectively. However, if the SSF contained As + α-amylase, as can be seen from Lane 1 and 2 in Fig. 2b, the peanut OB surface proteins shifted their SDS-PAGE-assessed Mw of 16–18 kDa gradually towards about 13–14 kDa, compared with the undigested OBs (Lane 5 in Fig. 2b). Therefore the appearance of α-amylase in the SSF changed the SDS-PAGE-assessed Mw of the surface proteins of the OBs. Since that the main function of α-amylase is the enzymatic digestion of carbohydrates such as starch or glycogen,29 it is speculated that the change in SDS-PAGE-assessed Mw of the OB surface proteins here may be due to that there are glycosidic bonds existing on the proteins, and SDS-PAGE-assessed Mw decreases after the glycosidic bonds being treated by α-amylase. The existence of glycosidic bonds on some OB surface proteins has been reported previously, such as on coconut OBs7 and Arabidopsis thaliana OBs.8 There is also the possibility that the tertiary rearrangements upon adsorption/desorption and interactions with As + α-amylase components, which could alter the hydrodynamic profile of the glycoproteins, thus affecting their mobility on the polyacrylamide gel. However, previous research didn't find such changes of the OB surface proteins after oral digestion,26,27 which probably because only microscope was used to observe the microstructural changes of the OB droplets, but no electrophoresis was executed to characterize the protein composition.
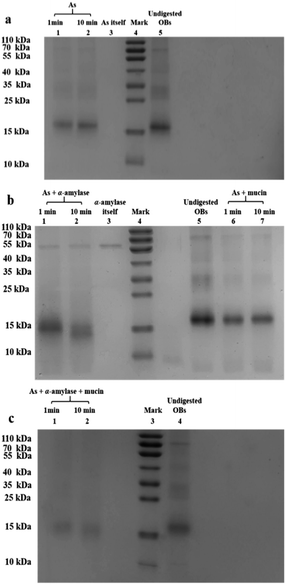 |
| Fig. 2 Effects of saliva component on the peanut OB surface proteins after oral digestion. (a) Lane 1 and 2, proteins after digested by As for 1 and 10 min respectively; Lane 3, As itself; Lane 4, marker proteins; Lane 5, undigested OBs; (b) Lane 1 and 2, OB proteins after digested by As + α-amylases for 1 and 10 min respectively; Lane 3, α-amylase in saliva itself; Lane 4, marker proteins; Lane 5, undigested OBs; Lane 6 and 7, OB proteins after digested by As + mucin for 1 and 10 min respectively; (c) Lane 1 and 2: OB proteins digested by As + α-amylases + mucin for 1 and 10 min, respectively; Lane 3, marker proteins; Lane 4, undigested OBs. | |
When the saliva contained As + mucin, the electrophoretic bands of the peanut OB proteins did not change significantly after digested (Lane 6, 7 in Fig. 2b respectively). Whereas, when the saliva contained As + α-amylase + mucin, as can be seen from Lane 1 and 2 in Fig. 2c, the proteins of 16–18 kDa decreased to about 13–14 kDa respectively as well. Therefore, these results indicate that α-amylase in saliva has an effect on the SDS-PAGE-assessed Mw of the peanut OB surface proteins during oral digestion, while mucin does not.
3.2.2. Particle size and ζ-potential change of the peanut OBs after oral digestion. Fig. 3a shows the particle size distribution of the peanut OBs after digested by different SSF, As, As + α-amylases, As + mucin or As + α-amylases + mucin. It can be seen that different saliva components have no significant effect on the peanut OB particle size, and the peak size of the OBs is almost the same with the peak size of the undigested OBs. At the same time, we did not observe a obvious flocculation of the emulsion after the addition of mucin, though mucin is a well-known flocculant of emulsions. This is probably because both the emulsion droplets and mucin are negatively charged under the pH in mouth (pH 6.8), so the electrostatic repulsion between the droplets and mucin prevents the flocculation to some extent. Together with the electrophoresis results, these results indicate that although the SDS-PAGE-assessed Mw of the surface proteins of the peanut OBs changed after the treatment of α-amylases, the OBs were not completely decomposed. This is consistent with the results by previous studies that no change in the microstructure morphology of OBs was observed using optical microscope after the oral digestion.26,27
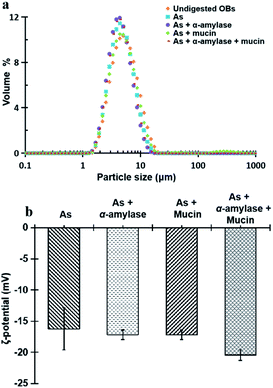 |
| Fig. 3 Particle size and size distribution (a) and ζ-potential (b) of peanut OBs after digested by different simulated saliva components at pH 6.8. | |
Fig. 3b displays the ζ-potential of the peanut OBs after digested by different SSF. It can be seen that addition of α-amylase or mucin, increases the value of the ζ-potential of the peanut OBs slightly. The increase of ζ-potential value by mucin was probably due to the negatively charged nature of themselves. For the α-amylases, on one hand, the α-amylase is also negatively charged at pH 6.8 in the SSF; while on the other hand, α-amylase may not only change the SDS-PAGE-assessed Mw of the OB surface proteins, but also destroy the structure of the OBs, such as glycosidic bonds on the surface proteins,7,8 which may also lead to changes in the charge properties of surface proteins. Therefore, in the following, we conducted an in-depth analysis of whether there are glycosidic bonds existing on the surface proteins of the peanut OBs, and how they interact with α-amylase and consequently affect the digestion of the peanut OBs before moving to the gastric and intestinal environment.
3.2.3. Microstructure of the peanut OBs with glycosidic bonds by CLSM. Con A can selectively bind to the α-mannopyranosyl and α-glucopyranosyl residues on proteins or lipids, forming a complex with green fluorescence.7 Fig. 4a, c, e, and f show the CLSM fluorescent micrographs of the Con A-stained peanut OBs undigested or digested by α-amylases. It was found that compared with OBs under light field (Fig. 4b), after Con A staining, the outer surface of the peanut OBs displayed green fluorescence (Fig. 4a), which indicates that glycosidic bonds do exist on the surface of the peanut OBs, though how the glycosides was produced was unknown. In order to eliminate the influence of sucrose which was added in the extraction process on the production of glycosides, the peanut OBs extracted without adding sucrose were also stained by Con A for comparison in Fig. 4 c and d. Comparing with the light field image in Fig. 4d, the outer surface of the peanut OBs in Fig. 4c still showed green fluorescence after Con A staining. This indicates that the existing glycosidic bonds on the surface of the peanut OBs were not produced by the sucrose added during the extraction process. The fluorescent and corresponding light field images for the peanut OBs digested by saliva containing As + α-amylases for 10 min and overnight, are also shown in Fig. 4e, f, g and h, respectively. It can be observed that the green fluorescence of the outer surface of individual peanut OB partially or completely disappeared after digested by As + α-amylases (indicated by the paired red/green/blue arrows). These results indicate there are glycosidic bonds on the surface of the peanut OBs, while α-amylase can destroy these glycosidic bonds to some extent during oral digestion.
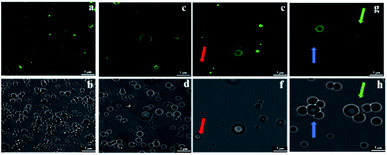 |
| Fig. 4 CLMS and light field images of the peanut OB surface before and during oral digestion. (a) & (b) Undigested OBs stained with Con A; (c) & (d) undigested OBs extracted without sucrose and stained with Con A; (e) & (f) OBs digested by As + α-amylase for 10 min and stained with Con A; (g) & (h) OBs digested by As + α-amylase overnight and stained with Con A. Green dots represent glucosides. Arrows of the same color are guide for comparison. | |
3.2.4. SDS-PAGE of peanut OBs with glycoproteins using PAS staining. We also tried PAS staining method to prove the existence of glycoproteins on the peanut OB surface. Fig. 5a and b show the SDS-PAGE results of the peanut OB proteins stained by Coomassie blue and PAS respectively, for comparison. The electrophoresis using PAS staining uses periodic acid to oxidize the glycogen of the glycosidic bonds, changing the hydroxyls on the adjacent C–C bonds into aldehyde groups.44 The resultant aldehyde compounds combine with Schiff base producing complex with purplish red fluorescence.44 Comparing the results of Coomassie blue staining in Fig. 5a, it can be observed in Fig. 5b that the proteins with Mw of 16–18 kDa are stained by PAS, and the darkness of the band increases with the increase of sample concentration (from 5 μl to 20 μl). The proteins with other Mw did not appear in the PAS electrophoresis image. These results indicate that glycosidic bonds may exist on the 16–18 kDa proteins of the peanut OBs. Jolivet et al., who used AgNO3 to stain the protein electrophoresis gel, also found the existence of glycosylated proteins in Arabidopsis thaliana OBs.8 They further identified two of the glycosylated proteins using nano-chromatography-mass spectrometry: an aquaporin and a glycosylphosphatidylinositol-anchored protein with unknown function. Protein glycosylation was also found through SDS-PAGE electrophoresis analysis in previous report,47 which indicate that with the increase of the size of the saccharides, a large number of high Mw glycation aggregates appeared. Therefore, combined with the observation of glycosidic bonds by CLMS in Fig. 4, the stained proteins by PAS in Fig. 5b, the shift of the electrophoresis bands to lower SDS-PAGE-assessed Mw of OB surface proteins after digested by α-amylase in Fig. 2b, and the slight change in ζ-potential in Fig. 3b, we conclude that at least part of the surface proteins of OBs formed glycosidic bonds, and α-amylase can act on these glycosidic bonds, causing the change of the SDS-PAGE-assessed Mw of proteins on the OB surface. Alternatively, as we mentioned above, there is the possibility that upon adsorption/desorption and interaction with the As component, tertiary rearrangements might also occur and alter the hydrodynamic characteristics of the glycoproteins and thus affect their mobility on the polyacrylamide gels.
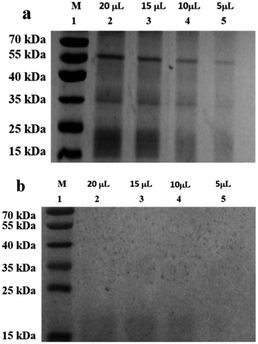 |
| Fig. 5 SDS-PAGE image of undigested peanut OBs using different staining methods with loading concentration as indicated. (a) Coomassie blue staining; (b) PAS staining. M, protein markers. The measurement for each staining method was performed for 3 times, and the resultant best figure is displayed in grey color. | |
3.3. Effects of saliva components on the gastric digestion of peanut OBs
3.3.1. Change in surface proteins. After oral digestion, the peanut OBs were further digested under the simulated gastric conditions, where pepsin hydrolyzes peptide bonds on the N-terminus sides of aromatic residues of proteins.48 Coomassie blue SDS-PAGE was first used to investigate the hydrolysis of the peanut OB surface proteins by pepsin after digestion by using different saliva components for 10 min. The results are shown in Fig. 6a–d. After oral digested by As and the following gastric digestion by SGF, we can see from Fig. 6a that, the 16–18 kDa oleosins, the 25 and 30 kDa caleosin, the 40 kDa steroleosin and the exogenous proteins with Mw greater than 41 kDa were all gradually degraded since the darkness of the SDS-PAGE bands decreased with the increase of digestion time. This means all of the peanut OB surface proteins were hydrolyzed to lower Mw peptides (about 6.5–14 kDa). This result is consistent to previous study by Gallier et al.,20 who found that almond OBs were rapidly digested in the gastric environment, and after 15 min of simulated gastric digestion, no further proteolysis was observed, and only several undigested peptides were detected.
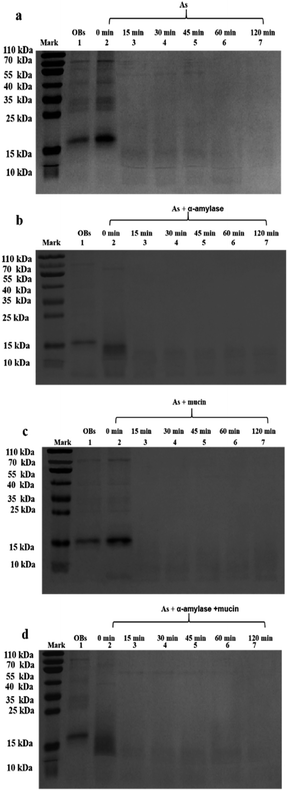 |
| Fig. 6 Effects of saliva component on Mw of the peanut OB surface proteins during gastric digestion. SSF containing (a) As; (b) As + α-amylases; (c) As + mucin; (d) As + α-amylases + mucin. In each frame: Lane 0, the marker proteins with Mw as indicated; Lane 1, undigested OBs; Lane 2–7, gastric digestion for 0, 15, 30, 45, 60, and 120 min, respectively. | |
In contrast, after oral digestion by As + α-amylase and the following gastric digestion by SGF, we can see by comparing Lane 2 with Lane 1 in Fig. 6b, that the peanut OB proteins of 16–18 kDa were shifted to lower SDS-PAGE-assessed Mw at 0 min during the gastric digestion. This is induced by the decomposition of the glycosidic bonds on the peanut OB surface by α-amylase as we discussed above. As the gastric digestion continued, e.g., for 15 minutes, the original 16–18 kDa oleosins have been decomposed into even lower Mw polypeptides of about 6.5–14 kDa (as shown in Lane 3 in Fig. 6b). No further change of the electrophoresis bands was seen after digestion for 120 min (Lane 3–7 in Fig. 6b).
During the oral digestion by As + mucin and the following gastric digestion by SGF, there was no significant change in the protein Mw at 0 min of gastric digestion compared to the undigested peanut OB proteins (Lane 2 compared to Lane 1 in Fig. 6c). After gastric digestion for 15 min, all oleosins (16–18 kDa) and other proteins with higher Mw have been decomposed, and there were no significant change in the electrophoresis lanes later until gastric digestion for 120 min (Lane 3–7 in Fig. 6c), similar to the results by As in Fig. 6a. Fig. 6d shows the SDS-PAGE results for the peanut OBs after oral digestion by As + α-amylase + mucin and following gastric digestion by SGF. Similar change in protein bands to that in Fig. 6b for OBs after oral digestion by As + α-amylase can be seen.
These results indicate that though the proteins on the peanut OB surface were decomposed due to the digestion of the glycosidic bonds by α-amylase in the saliva, no significant change was observed in the SDS-PAGE for these proteins during further gastric digestion.
3.3.2. Droplet size, microstructure and ζ-potential. As shown in Fig. 7a, after oral digestion by different SSF and the following gastric digestion by SGF for 120 min, at which point the gastric digestion was expected to be completed, the digested OBs showed an irregular multimodal distribution, and the average particle size d4,3 of the OBs were larger than that of the undigested oil bodies (2.55 ± 0.05 μm from Fig. 1b). This was similar to the result observed for sunflower seed OBs by White et al.19 The order of the average particle size d4,3 was As + α-amylase + mucin (109.25 ± 6.85 μm) > As + mucin (80.62 ± 9.79 μm) > As (56.53 ± 4.57 μm) > As + α-amylase (45.72 ± 5.23 μm). A peak around 4 μm appeared for the OBs after oral digested by As + α-amylase in Fig. 7a. The images in Fig. 7b shows the CLSM images of the peanut OBs after oral digestion with different SSF and the following gastric digestion for 120 min. It can be seen in Fig. 7b1 that the size of the peanut OBs are larger than that of the undigested ones as shown in Fig. 1a. By the action of pepsin, the surface structure of oil bodies was destroyed to some extent and the oil droplets fused into large oil droplets due to the insufficiently strong surface. Meanwhile, aggregated OBs are seen as well, which may be caused by the weak electrostatic attraction between oil droplets at this pH. In the image in Fig. 7b2, after oral digestion by As + α-amylases and gastric digestion, the oil droplets are much larger than those in Fig. 7b1, probably because the oil droplet interface was destroyed more severely due to the presence of α-amylase. Therefore oil droplet coalesced into more and larger droplets. There are also droplets with smaller droplet size in Fig. 7b2 as shown by the green spots. Intensive aggregations after oral digestion by As + mucin appeared in Fig. 7b3, which is probably caused by the strong electrostatic attraction between mucin and the oil droplets as mucin is negatively charged and oil droplets are positively charged at this acidic pH. In Fig. 7b4, more aggregations of oil droplets than other samples and larger oil droplets were seen under the action of both α-amylases and mucin.
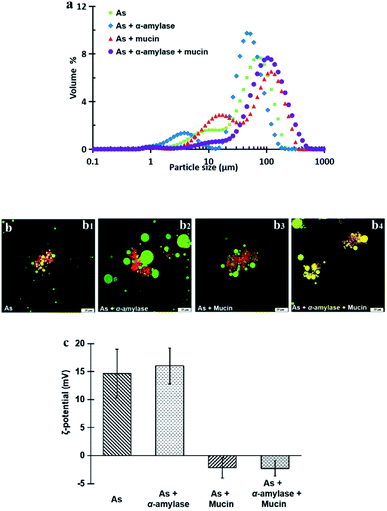 |
| Fig. 7 Effects of saliva component on the digested OB emulsions after gastric digestion for 120 min: (a) droplet size distribution, (b) the CLMS images with SSF as indicated (b1–b4 represent the OBs after the action of As, As + α-amylase, As + mucin or As + α-amylase + mucin) and (c) the ζ-potential at pH 2.5. Scale bars at the bottom right in (b) represent 25 μm. | |
In Fig. 7c, the ζ-potential for the peanut OBs after oral digestion by As and the following gastric digestion for 120 min, is about 14.7 mV, which is similar to that of soybean OBs in a previous study.18 Combining this with the results in droplet size analysis and in SDS-PAGE about the protein change during gastric digestion, we speculate that the peanut OBs coalesce during gastric digestion due to the hydrolysis and destruction of peanut OB surface proteins by pepsins. Furthermore, the destruction of the surface proteins causes the charge on the surface of the OB droplets to decrease, resulting in insufficient electrostatic repulsion or steric hindrance, and consequently the OBs became aggregated to some extent.22,49 The ζ-potential of the digested OB emulsion was positively charged with around 16.0 ± 3.2 mV after oral digestion by As + α-amylase and the following gastric digestion (in Fig. 7c). Since α-amylase is a protein, its own positive charges contribute to the positive ζ-potential value of the digested OB emulsion. Liu et al. showed as well that the soybean OB-stabilized emulsion had increased positive charges as the degree of enzymolysis increased.22 Therefore the increase of droplet size of the peanut OBs here is mostly from their coalescence rather than aggregation. In addition, it was reported that the glycosylation of the membrane proteins plays a protective role against hydrolysis by pepsin.50 We believe that this enhanced coalescence phenomenon was because of the destruction of glycosidic bonds on the OB surface proteins by α-amylase, which in turn promote the hydrolysis of the OB surface proteins by the pepsins. This strong digestive effect continues until the late stage of the digestion. The ζ-potential of the digested OBs after oral digestion by As + mucin and the following gastric digestion was slightly negatively charged with value around −2.2 ± 1.8 mV (Fig. 7c), which may be due to the neutralization by the negative charge of mucin.51 Thus the strong aggregations (Fig. 7b3) can be explained by the bridging flocculation of the digested droplets caused by the negatively charged mucin.28 The ζ-potential of the digested OBs after oral digestion by As + α-amylase + mucin (Fig. 7c) was similar to that of the digested OBs after oral digestion by As + mucin. But from the CLSM image in Fig. 7b4, larger oil droplets with aggregations are seen, which are expected due to both coalescence and flocculation caused by the combination of α-amylase and mucin as illustrated above in Fig. 7b2 and b3 respectively.
3.4. Effects of saliva components on intestinal digestion of peanut OBs
3.4.1. Droplet size, microstructure and ζ-potential. As seen in Fig. 8a, after oral digestion by different SSF, gastric digestion by SGF and intestinal digestion by SIF for 120 min, the particle size of peanut OBs was reduced compared to the particle size after gastric digestion (in Fig. 7a), and the size distribution shows a wide multimodal distribution. In the intestinal digestion stage, under the action of bile salts, the surface proteins of OBs can be replaced, and the internal oil inside the OBs will be hydrolyzed by the pancreatin.52 Then as the droplet surfaces were destroyed further, the oil inside the droplets was continuously digested resulting in the slight decrease of the droplet size.53 The CLSM image in Fig. 8b1 shows that after the action of As in SSF and the following gastric and intestinal digestion, the droplets aggregated (yellow) to some extent while there were still small oil droplets (green) distributed, which is consistent with the droplet size distribution (with averaged size of 52.58 ± 2.55 μm) measured by the light scattering in Fig. 8a. The digestion products of oil bodies probably also spontaneously coalesced to form larger oil droplets than the oil bodies themselves although the total amount of oil droplets decreased. One difference between the gastric digestion and intestinal digestion stage is that the OB droplets were negatively charged in the intestinal environment while positively charged in the gastric environment. Therefore the flocculation of the droplets became weaker due to the increase of the repulsion between droplets (also supported by the ζ-potential results displayed in Fig. 8c). The image in Fig. 8b2 shows that after the action of As + α-amylase and the following gastric and intestinal digestion, both the aggregation (yellow) and the oil droplets (green) are smaller than those in Fig. 8b1. These are due to the decomposition of the glycosidic bonds by the α-amylase which facilitated the replacement of the surface proteins on the peanut OBs by bile salts and speeded up fat digestion. In contrast, Lesmes et al.54 found inefficient displacement of glycated proteins at the oil-water interface during the digestion of β-lactoglobulin–dextran Maillard conjugates in the small intestine, which may impede lipase adsorption or lipolytic activity. This was probably because that the glycosidic bonds were not broken by α-amylase.
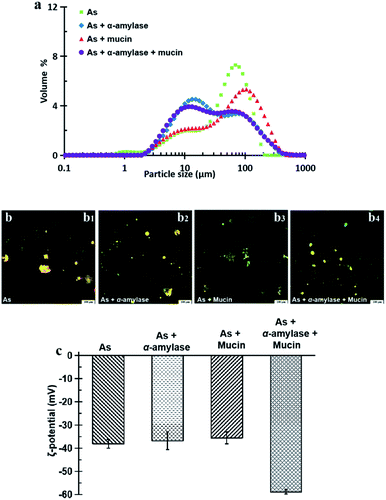 |
| Fig. 8 Effects of saliva component on the digested OB emulsions after intestinal digestion for 120 min: (a) droplet size distribution, (b) the CLMS images with SSF as indicated (b1–b4 represent the OBs after the action of As, As + α-amylase, As + mucin or As + α-amylase + mucin) and (c) ζ-potential at pH 7.0. Green dots in (b) are the remaining oil droplets while yellow dots are the remaining oil droplet and protein mixtures. Scale bars at the bottom right in (b) represent 100 μm. | |
The CLSM image in Fig. 8b3 shows that after the action of As + mucin and the following gastric and intestinal digestion, the oil droplets and the aggregations are more evenly distributed. Mucin mainly plays a lubricating role in the oral cavity, and it cannot be digested by SGF or SIF either. From the original mucin added in SSF to the following gastric and intestinal digestion, however, the relative concentration of mucin to oil droplets in the intestinal digestion stage increased compared to that in the oral digestion stage as the oil droplets were digested in the intestinal environment. Therefore, the high concentration non-adsorbing negatively charged biopolymer of mucin in the aqueous phase of the OB emulsion are expected to increase the attraction between the oil droplets (negatively charged protein emulsions) through the depletion effect.28,51 The results for the peanut OBs after oral digestion by SSF of As + α-amylase + mucin, gastric digestion by SGF, and intestinal digestion by SIF are displayed in Fig. 8b4, which can be viewed as the superposition of results shown in Fig. 8b2 and b3. Clearly, they are from the combination effects of both α-amylase and mucin in the saliva.
All of the peanut OB emulsions had negative ζ-potentials after digestion by SSF with different components, SGF, and SIF as shown in Fig. 8c. The digested products of the intestinal finally were composed of a large amount of the mixture of PLs, FFA and polypeptides, or undigested OBs which are all negatively charged.52,55 When the simulated saliva contains both α-amylase and mucin (As + α-amylase + mucin), the negative potential was the largest, which was mainly due to the dual effect of α-amylase and mucin.
3.4.2. FFA released during intestinal digestion of the peanut OBs. By measuring the FFA release rate at different times during the intestinal digestion process, the digestion rate and degree of the oil digested in the emulsion can be reflected.20 As seen in Fig. 9, the rate of FFA release was fast in the first 10 min, but then slowed down from 20 min until 120 min since the accumulation of lipolytic products at the interface of the OBs prevented the pancreatic lipase from accessing the triglyceride core.52 There was no significant difference in the early stage of FFA release among the four investigated SSF, but in the later stages, the As + mucin + α-amylase treated OBs has the highest FFA release amount, followed by the As + mucin treated OBs, then the As + α-amylase treated OBs, while the As-treated OBs had the lowest FFA release amount. After the action of pancreatin, the digested OBs spontaneously coalesced forming larger oil droplets. The α-amylase in the saliva destroys the glycosidic bonds on the surface of the peanut OBs as discussed above, which facilitate the replacement of the surface proteins by bile salts in the intestinal tract, and consequently both the release rate and the amount of fat was increased during the intestinal digestion. The mucin could cause depletion flocculation of the peanut OBs in the intestinal, which may hinder the fusion of oil droplets and thus promoting fat digestion by increasing their contacting surface area with bile salts.54 The superposition of the two effects results in the maximum release of FFA for OBs treated by SSF of As + mucin + α-amylase.
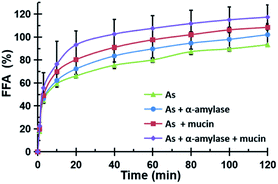 |
| Fig. 9 Effects of saliva components on the FFA release rate of the peanut OB emulsion at different times during intestinal digestion. | |
4. Conclusions
This study investigated the effects of the main saliva components on the digestion of the peanut OBs extracted by aqueous medium method using complete in vitro oral-gastric-intestinal digestion simulation. With CLMS technique using Con A as the staining agent and the electrophoresis characterization using PAS method, glycosidic bonds were found to exist on the surface of the peanut OBs. When saliva components contain α-amylase, it can break the glycosidic bonds on the surface of the OBs, and the OBs structure is disrupted more severely and form larger oil droplets as they coalesced, and thus promoting the digestion of the peanut OBs in the gastric and intestinal environment. When saliva components contain mucin, bridging flocculation of OBs occurs in gastric environment, and depletion flocculation in intestinal environment, in addition to the oil droplets fusion. The former hinders the fusion of oil droplets in the gastric digestion and the latter promotes fat digestion by increasing the contacting surface area of OBs with bile salts in the intestinal environment.
Conflicts of interest
There are no conflicts to declare.
Acknowledgements
The research was supported by Hubei Provincial Department of Education (D20181403) and National Natural Science Foundation of China (31571797, 31401649). Nan Yang was also supported by Hubei University of Technology (YXQN2016001).
References
- A. H. C. Huang, Oil bodies and oleosins in seeds, Annu. Rev. Plant Biol., 1992, 43(1), 177–200 CrossRef CAS.
- A. A. Fani, A. Dave and H. Singh, Nature-assembled structures for delivery of bioactive compounds and their potential in functional food, Front. Chem., 2020, 8, 564021 CrossRef PubMed.
- J. T. Tzen and A. H. Huang, Surface structure and properties of plant seed oil bodies, J. Cell Biol., 1992, 117(2), 327–335 CrossRef CAS PubMed.
- J. T. C. Tzen, C. Y. Zao and P. Laurent, et al., Lipids, proteins, and structure of seed oil bodies from diverse species, Plant Physiol., 1993, 101(1), 267–276 CrossRef CAS PubMed.
- C. V. Nikiforidis, A. Matsakidou and V. Kiosseoglou, Composition, properties and potential food applications of natural emulsions and cream materials based on oil bodies, RSC Adv., 2014, 4(48), 25067–25078 RSC.
- A. Barre, M. Simplicien and G. Cassan, et al., Oil bodies (oleosomes): Occurrence, structure, allergenicity, Rev. Fr. Allergol., 2018, 58(8), 574–580 CrossRef.
- A. C. Dave, A. Ye and H. Singh, Structural and interfacial characteristics of oil bodies in coconuts (Cocos nucifera L.), Food Chem., 2019, 276, 129–139 CrossRef CAS PubMed.
- P. Jolivet, E. Roux and S. d'Andrea, et al., Protein composition of oil bodies in Arabidopsis thaliana ecotype WS, Plant Physiol. Biochem., 2004, 42(6), 501–509 CrossRef CAS PubMed.
- D. Iwanaga, D. Gray and E. A. Decker, et al., Stabilization of soybean oil bodies using protective pectin coatings formed by electrostatic deposition, J. Agric. Food Chem., 2008, 56(6), 2240–2245 CrossRef CAS PubMed.
- B. I. Zielbauer, A. J. Jackson and S. Maurer, et al., Soybean oleosomes studied by small angle neutron scattering (SANS), J. Colloid Interface Sci., 2018, 529, 197–204 CrossRef CAS PubMed.
- D. A. Gray, G. Payne and D. J. McClements, et al., Oxidative stability of echium plantagineum seed oil bodies, Eur. J. Lipid Sci. Technol., 2010, 112(7), 741–749 CrossRef CAS.
- I. D. Fisk, D. A. Whit and A. Carvalho, et al., Tocopherol-an intrinsic component of sunflower seed oil bodies, J. Am. Oil Chem. Soc., 2006, 83(4), 341–344 CrossRef CAS.
- J. Ding, J. Wen and J. Wang, et al., The physicochemical properties and gastrointestinal fate of oleosomes from non-heated and heated soymilk, Food Hydrocolloids, 2020, 100, 105418 CrossRef CAS.
- L. Zhao, Y. Chen and Y. Chen, et al., Effects of pH on protein components of extracted oil bodies from diverse plant seeds and endogenous protease-induced oleosin hydrolysis, Food Chem., 2016, 200, 125–133 CrossRef CAS PubMed.
- A. Shakerardekani, R. Karim and F. Mirdamadiha, The effect of sorting on aflatoxin reduction of pistachio nuts, J. Food Agric. Environ., 2012, 10(1), 459–461 CAS.
- A. Matsakidou, C. G. Biliaderis and V. Kiosseoglou, Preparation and characterization of composite sodium caseinate edible films incorporating naturally emulsified oil bodies, Food Hydrocolloids, 2013, 30(1), 232–240 CrossRef CAS.
- C. V. Nikiforidis, C. G. Biliaderis and V. Kiosseoglou, Rheological characteristics and physicochemical stability of dressing-type emulsions made of oil bodies-egg yolk blends, Food Chem., 2012, 134(1), 64–73 CrossRef CAS.
- N. Wu, X. Huang and X. Yang, et al., In vitro assessment of the bioaccessibility of fatty acids and tocopherol from soybean oil body emulsions stabilized with ι-carrageenan, J. Agric. Food Chem., 2012, 60(6), 1567–1575 CrossRef CAS PubMed.
- D. A. White, I. D. Fisk and S. Makkhun, et al., In vitro assessment of the bioaccessibility of tocopherol and fatty acids from sunflower seed oil bodies, J. Agric. Food Chem., 2009, 57(13), 5720 CrossRef CAS PubMed.
- S. Gallier and H. Singh, Behavior of almond oil bodies during in vitro gastric and intestinal digestion, Food Funct., 2012, 3(5), 547 RSC.
- F. Beisson, N. Ferté and S. Bruley, et al., Oil-bodies as substrates for lipolytic enzymes, Biochim. Biophys. Acta, Mol. Cell Biol. Lipids, 2001, 1531(1), 47–58 CrossRef CAS.
- C. Liu, R. Wang and S. He, et al., The stability and gastro-intestinal digestion of curcumin emulsion stabilized with soybean oil bodies, LWT–Food Sci. Technol., 2020, 131, 109663 CrossRef CAS.
- M. Boland, Human digestion a processing perspective, J. Sci. Food Agric., 2016, 96(7), 2275–2283 CrossRef CAS PubMed.
- G. H. Carpenter, The secretion, components, and properties of saliva, Annu. Rev. Food Sci. Technol., 2013, 4(1), 267–276 CrossRef CAS PubMed.
- T. Grassby, G. Mandalari and M. M. L. Grundy, et al., In vitro and in vivo modeling of lipid bioaccessibility and digestion from almond muffins: The importance of the cell-wall barrier mechanism, J. Funct. Foods, 2017, 37, 263–271 CrossRef CAS PubMed.
- M. M. L. Grundy, T. Grassby and G. Mandalari, et al., Effect of mastication on lipid bioaccessibility of almonds in a randomized human study and its implications for digestion kinetics, metabolizable energy, and postprandial lipemia, Am. J. Clin. Nutr., 2015, 101(1), 25–33 CrossRef CAS PubMed.
- P. R. Ellis, C. W. Kendall and Y. Ren, et al., Role of cell walls in the bioaccessibility of lipids in almond seeds, Am. J. Clin. Nutr., 2004, 80(3), 604–613 CrossRef CAS PubMed.
- A. Sarkar, K. K. T. Goh and H. Singh, Colloidal stability and interactions of milk-protein-stabilized emulsions in an artificial saliva, Food Hydrocolloids, 2009, 23(5), 1270–1278 CrossRef CAS.
- N. Rohleder and U. M. Nater, Determinants of salivary alpha-amylase in humans and methodological considerations, Psychoneuroendocrinology, 2009, 34(4), 469–485 CrossRef CAS PubMed.
- S. Lei, Biomimetic surfaces of biomaterials using mucin-type glycoproteins, Trends Glycosci. Glycotec, 2000, 12(66), 229–239 CrossRef.
- S. M. Rafiquzzaman, E. Y. Kim and Y. R. Kim, et al., Antioxidant activity of glycoprotein purified from Undaria pinnatifida measured by an in vitro digestion model, Int. J. Biol. Macromol., 2013, 62, 265–272 CrossRef CAS PubMed.
- M. Espert, J. Borreani and I. Hernando, et al., Relationship between cellulose chemical substitution, structure and fat digestion in o/w emulsions, Food Hydrocolloids, 2017, 69, 76–85 CrossRef CAS.
- S. Sabouri, A. J. Wrigh and M. Corredig, et al., In vitro digestion of sodium caseinate emulsions loaded with epigallocatechin gallate, Food Hydrocolloids, 2017, 69, 350–358 CrossRef CAS.
- H. Singh and A. Ye, Structural and biochemical factors affecting the digestion of protein-stabilized emulsions, Curr. Opin. Colloid Interface Sci., 2013, 18(4), 360–370 CrossRef CAS.
- U. Gawlik-Dziki, D. Dziki and B. Baraniak, et al., The effect of simulated digestion in vitro on bioactivity of wheat bread with Tartary buckwheat flavones addition, LWT–Food Sci. Technol., 2009, 42(1), 137–143 CrossRef CAS.
- Z. Zhang, R. Zhang and L. Zou, et al., Encapsulation of curcumin in polysaccharide-based hydrogel beads: Impact of bead type on lipid digestion and curcumin bioaccessibility, Food Hydrocolloids, 2016, 58, 160–170 CrossRef CAS.
- N. Yang, C. X. Su and Y. M. Zhang, et al., In situ nanomechanical properties of natural oil bodies studied using atomic force microscopy, J. Colloid Interface Sci., 2020, 570, 362–374 CrossRef CAS PubMed.
- N. Yang, Y. N. Fang and C. X. Su, et al., Structure and tribology of κ-carrageenan gels filled with natural oil bodies, Food Hydrocolloids, 2020, 107, 105945 CrossRef CAS.
- AOAC, Official methods of analysis of international, Association of Official Analytical Chemists, Arlington, VA, USA, 2000 Search PubMed.
- C. X. Su, Y. N. Feng and J. Ye, et al., Effect of sodium alginate on the stability of natural soybean oil body emulsions, RSC Adv., 2018, 8(9), 4731–4741 RSC.
- J. B. Misra, Variation in nitrogen-to-protein conversion factor for peanut, Peanut Sci., 2001, 28(2), 48–51 CrossRef CAS.
- Y. M. Zhang, N. Yang and Q. Wang, et al., Improving the stability of oil body emulsions from diverse plant seeds using sodium alginate, Molecules, 2019, 24(21), 3856 CrossRef CAS PubMed.
- A. M. Nash and E. N. Frankel, Limited extraction of soybeans with hexane, J. Am. Oil Chem. Soc., 1986, 63(2), 244–246 CrossRef CAS.
- R. M. Zacharius, T. E. Zell and J. H. Morrison, et al., Glycoprotein staining following electrophoresis on acrylamide gels, Anal. Biochem., 1969, 30(1), 148–152 CrossRef CAS PubMed.
- M. Minekus, M. Alminger and P. Alvito, et al., A standardised static in vitro digestion method suitable for food an international consensus, Food Funct., 2014, 5(6), 1113–1124 RSC.
- S. Mun, Y. R. Kim and D. J. McClements, Control of β-carotene bioaccessibility using starch-based filled hydrogels, Food Chem., 2015, 173, 454–461 CrossRef CAS PubMed.
- M. Zhang, J. Zheng and K. Ge, et al., Glycation of α-lactalbumin with different size saccharides: Effect on protein structure and antigenicity, Int. Dairy J., 2014, 34(2), 220–228 CrossRef CAS.
- J. S. Fruton, S. Fujii and M. H. Knappenberger, The mechanism of pepsin action, Proc. Natl. Acad. Sci. U. S. A., 1961, 47(6), 759 CrossRef CAS PubMed.
- A. Sarkar, K. K. T. Goh and R. P. Singh, et al., Behaviour of an oil-in-water emulsion stabilized by β-lactoglobulin in an in vitro gastric model, Food Hydrocolloids, 2009, 23(6), 1563–1569 CrossRef CAS.
- S. Gallier, A. Ye and H. Sing, Structural changes of bovine milk fat globules during in vitro digestion, J. Dairy Sci., 2012, 95(7), 3579–3592 CrossRef CAS PubMed.
- E. Silletti, M. H. Vingerhoeds and W. Norde, et al., The role of electrostatics in saliva-induced emulsion flocculation, Food Hydrocolloids, 2007, 21(4), 596–606 CrossRef CAS.
- S. Gallier, H. Tate and H. Singh, et al., In vitro gastric and intestinal digestion of a walnut oil body dispersion, J. Agric. Food Chem., 2013, 61(2), 410–417 CrossRef CAS PubMed.
- M. Golding and T. J. Wooster, The influence of emulsion structure and stability on lipid digestion, Curr. Opin. Colloid Interface Sci., 2010, 15(1–2), 90–101 CrossRef CAS.
- U. Lesmes and D. J. McClements, Controlling lipid digestibility: Response of lipid droplets coated by β-lactoglobulin-dextran Maillard conjugates to simulated gastrointestinal conditions, Food Hydrocolloids, 2012, 26, 221–230 CrossRef CAS.
- F. Liu, C. Ma and R. Zhang, et al., Controlling the potential gastrointestinal fate of β-carotene emulsions using interfacial engineering: Impact of coating lipid droplets with polyphenol-protein-carbohydrate conjugate, Food Chem., 2017, 221, 395–403 CrossRef CAS PubMed.
|
This journal is © The Royal Society of Chemistry 2021 |
Click here to see how this site uses Cookies. View our privacy policy here.