DOI:
10.1039/D1RA02605D
(Paper)
RSC Adv., 2021,
11, 17249-17258
A dynamic evolution model of coal permeability during enhanced coalbed methane recovery by N2 injection: experimental observations and numerical simulation
Received
2nd April 2021
, Accepted 4th May 2021
First published on 10th May 2021
Abstract
China boasts abundant coalbed methane (CBM) resources whose output is significantly influenced by the permeability of coal reservoirs. However, the permeability of coal reservoirs in China is generally low, which seriously restricts the efficient exploitation of CBM. To solve this problem, enhanced coalbed methane (ECBM) recovery by N2 injection has been widely adopted in recent years. However, there exists little research conducted on coal permeability behavior during the displacement process. In this work, a series of physical simulation experiments were conducted on CH4 displacement by N2 injection to investigate the dynamic evolution of coal permeability. Based on the dual-porosity medium property of coal, a dynamic evolution model of coal permeability considering the combined effects of matrix shrinkage/swelling and effective stress was proposed to reflect the ECBM recovery process. The accuracy of this theoretical model was verified by matching the numerical simulation results with the experimental data. The findings show that coal permeability increases at a gradually decelerating rate with the passage of displacement time, and finally tends to be stable. In addition, raising N2 injection pressure can dramatically enhance CH4 recovery and shorten the displacement time, which indicates that ECBM recovery by N2 injection is a feasible technical method for low-permeability coal reservoirs. Meanwhile, the model proposed in this study can be applied to the prediction of CBM production, and is of guiding significance for engineering applications.
1 Introduction
Coalbed methane (CBM) is an unconventional gas sharing a symbiotic relationship with coal. China boasts an abundant CBM reserve whose development and usage are meaningful. According to statistics, over 35 trillion m3 of CBM are reserved in coal seams less than 2000 m in depth, featuring enormous development potential.1–3 Since methane (CH4) is the main component of CBM, the calorific value of 1 m3 of CBM is up to about 35.9 MJ which is equivalent to that of natural gas. Moreover, CBM is an efficient and clean energy, which does not produce any waste gas after combustion.4 On the contrary, CBM is also a considerable hazard in underground mining. It may induce gas outbursts and gas explosions that seriously threaten safe production in coal mines. Meanwhile, CBM discharged into the atmosphere may produce a serious greenhouse effect which is 21 times greater than that of CO2. Such a greenhouse effect is extremely destructive to global ecological environment.5 Therefore, the exploitation and utilization of the CBM resource is of great significance to both safe mining and environmental protection.
However, the permeability of coal reservoirs in China is generally low, being only 0.01–5 mD, which brings great challenges to underground CBM development.6,7 Thus, enhancing the permeability of coal reservoirs is the key to improving the efficiency of CBM extraction. Numerous researches have been carried out to investigate the permeability enhancement mechanisms and technologies of coal reservoirs, and measures such as hydraulic flushing, hydraulic fracturing, blasting loosening and advanced borehole have been taken.8–11 Although these measures achieved a certain effect, but they require a large engineering quantity and can hardly be applied in several mining areas. To resolve this problem, enhanced coalbed methane (ECBM) recovery by gas injection (that is, injecting some gases, such as N2 or CO2, into coal seams to displace CBM and promote CBM recovery) has been widely adopted and become a research hotspot worldwide.12,13 So far, the displacement method has been applied successfully in multiple countries including American, Australia, India, etc., and achieved fruitful application effects.14–16 Recently, many scholars have made much research on the displacement mechanism of binary gas flowing in porous media. Based on the molecular motion theory, Hu et al.17 analyzed the self-diffusion and inter-diffusion of CO2–CH4 multi component gas systems in coal. Connell et al.18 carried out a series of enhanced drainage core floods to develop a triple porosity model, which realistically described the observed gas migration during the displacement process. Liu et al.19 conducted CO2-ECBM experiments on cuboid coal samples to monitor the variation on pore pressure, gas flow rate and outlet concentration by increasing injection pressure, also established an effective economic cost model for guiding engineering designs. Kolak and Burruss20 evaluated the potential of mobilizing non-methane hydrocarbons during CO2 storage in different rank coal samples, and acquired mobilized amount of polycyclic aromatic hydrocarbons (PAHs) with increasing of coal rank. Ranathunga et al.21 investigated low rank coal samples to analyze the potential for CO2-ECBM. Result indicated that super-critical CO2 on CH4 recovery was independent of coal rank or maturity, whereas high CO2 pressure may lead to a negative effect on CH4 production. Zeng et al.22 proposed an internally-consistent adsorption–strain–permeability model, including gas adsorption/desorption, coal deformation, and permeability evolution, and described the ECBM process of CH4 displacement by CO2. Moreover, Yang et al.23 conducted molecular simulation to reveal the competitive adsorption mechanism of CH4, CO2, and N2 in coal. Bing et al.24 investigated influences of volumes, frequencies and modes of CO2 injection on methane production and recovery by numerical simulation.
Previous researches indicate that ECBM recovery by CO2 injection works as a result of the competitive adsorption between CO2 and CH4. Therefore, the adsorption capacity of the coal matrix increases and the coal permeability corresponding decreases, which can not achieve a ideal displacement effect.25,26 In contrast, ECBM recovery by N2 injection is more advantageous in low-permeability coal reservoirs. That is due to the adsorption capacity of the coal matrix on N2 is weaker than that on CH4, thereby CH4 cannot be displaced through competitive adsorption.27 Instead, CH4 desorption is promoted through reducing the CH4 partial pressure. Previous studies were mainly focused on the effect of CH4 displacement, and there is a lack of investigation on the dynamic evolution of coal permeability during CH4 displacement by N2 injection.28 however, lacking related researches on N2-ECBM to date, the essential mechanism of ECBM recovery by N2 injection has not been revealed, failing to effectively guide engineering application.
In this study, a series of physical simulation experiments of CH4 displacement by N2 injection were performed on the basis of actual coal seam parameters. The combined effects of matrix swelling/shrinkage and effective stress on the dynamic evolution of coal permeability during N2-ECBM displacement process were analyzed to propose a dynamic evolution model of coal permeability. And the accuracy of the model was verified by matching the numerical simulation results with the experimental data. The findings can serve as a reference for the design, parameter optimization, and theoretical research of gas displacement engineering.
2 Experimental methodology
In the hope of more really reflecting the engineering background, the simulation experiments of CH4 displacement by N2 was carried out using large-sized (Φ 100 mm × 300 mm) samples based on the actual coal seam occurrence parameters. And the coal permeability change was detected under different experimental conditions. The coal samples used in the experiments were anthracite collected in Jiulishan Coal Mine, Henan Province, China. Based on BET (low temperature nitrogen adsorption) and industrial analysis methods,29 the related basic parameters of coal sample are determined. As seen in Table 1, experimental coal samples are rich in pore and fracture structures, which conduces to truly simulating the process of permeation and displacement in original physical state of coal. Therefore, it has a definite guiding significance and reference value for ECBM engineering.
Table 1 Related basic parameters of coal sample
Parameter |
Value |
Total surface area (m2 g−1) |
52.8621 |
Median pore diameter (nm) |
8.13 |
Porosity |
0.08 |
Apparent density (g cm−3) |
1.49 |
Water content (%) |
4.12 |
Ash content (%) |
9.72 |
Volatile content (%) |
11.18 |
Fixed carbon |
74.98 |
2.1 Experimental system
The experiments of CH4 displacement by N2 injection were performed in this work were performed with the self-built displacement system which was mainly composed of a servo loading, gas injection, vacuum extraction, flow measurement, gas sample acquisition, and gas component analysis units. The axial loading on the coal sample was provided by the oil cylinder and stabilized by the accumulator. And the displacement pressure was exerted by the gas cylinder, and the components and concentrations of acquired gas samples during the displacement process were analyzed by the GC-4000A gas chromatograph. The experimental device is illustrated in Fig. 1.
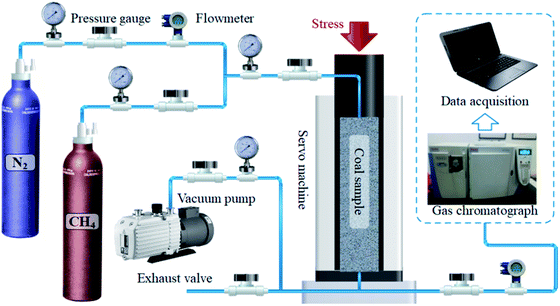 |
| Fig. 1 Diagram of the experimental device. | |
2.2 Preparation of coal samples
Experimental coal samples were anthracite whose initial CBM pressure was 0.5 MPa. First, the raw coal collected from the field was crushed and screened into pulverized coal with the particle size of below 0.25 mm. Then, the screened pulverized coal was evenly added into the displacement chamber and tamped in layers. Finally, the displacement cavity was placed on the experimental platform of the servo machine, and a load of 105 kN was applied on the coal sample at a rate of 100 N s−1, which is equivalent to 13.6 MPa of ground stress, for 24 h to simulate actual occurrence state of coal seam.
2.3 Experimental scheme
2.3.1 Coal permeability tests under different gas source conditions. Firstly, the vacuum unit of the experimental system was turned on to vacuumize the coal samples for more than 12 h, and the pressure in the cavity was less than 100 Pa. Then the coal permeability changes were test by N2 and CH4, respectively, under different gas injection pressure. Coal permeability test is carried out by the steady-state method according to Darcy's law, and the permeability calculation formula is expressed as: |
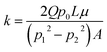 | (1) |
where k is coal permeability, m2; Q is gas flow rate per unit time, m3 s−1; p0 is the standard atmospheric pressure, 1.01 × 105 Pa; L is effective seepage length of coal samples, m; μ is dynamic viscosity of the gas, Pa s; p1 is inlet gas pressure, MPa; p2 is outlet gas pressure; and A is effective seepage cross-sectional area of coal sample, m2.
2.3.2 Coal permeability changes during N2-ECBM process. After the vacuum treatment of the coal samples, the high-purity (99.99%) CH4 was injected into the cavity until the adsorption pressure of coal samples reached the actual coal seam pressure 0.5 MPa, and the equilibrium time was maintained for more than 24 h. Next, high-purity (99.99%) N2 was used for simulating ECBM process. Three groups tests were designed in the displacement experiment, with the injection pressure of N2 was 0.3, 0.5, and 0.7 MPa, respectively. During the process of CH4 displacement by N2, the changes of gas flow rates at the inlet and outlet were recorded, and the gases at the outlet were collected regularly.
3 Results and discussion
3.1 Permeability tests of coal to CH4 and N2
Permeability test of coal to CH4 and N2 has two purposes. One is for analyzing the coal permeability changes under different gas injection pressures, the other is to compare the coal permeability differences between CH4 and N2 injection conditions.
As can be seen from the Table 2, under the same gas source condition, coal permeabilities decreases continuously with the rise of gas injection pressure, and the decline rate gradually decelerates. Changing trends of coal permeabilities are displayed in Fig. 2. The fitting results of experimental data indicate that the coal permeabilities shares a negative exponential relationship with the gas injection pressure under different gas injection conditions of CH4 and N2, with the squared correlation coefficients R2 being 0.9312 and 0.9466, respectively. The results are consistent with the permeability test results of Zhao et al. on the large size anthracite samples from Qinshui 3# coal seam.30 It is worth noting that the permeabilities of coal to N2 is generally higher than that of CH4 under the same injection pressure. As gas injection pressure is 0.54 MPa, the permeability of coal to N2 reaches 1.47 times that of CH4. While the gas injection pressure exceeds a certain threshold, for example, CH4 pressure is greater than 0.65 MPa, the coal permeability shows a sign of recovery trend with an asymmetric “U” pattern variation.
Table 2 Results of coal permeability test
Gas source |
Injection pressure (MPa) |
Coal permeability (mD) |
N2 |
0.24 |
0.78 |
0.35 |
0.62 |
0.44 |
0.44 |
0.54 |
0.37 |
0.65 |
0.30 |
0.74 |
0.27 |
0.84 |
0.26 |
CH4 |
0.25 |
0.51 |
0.35 |
0.40 |
0.48 |
0.31 |
0.54 |
0.25 |
0.64 |
0.22 |
0.76 |
0.20 |
0.8 |
0.21 |
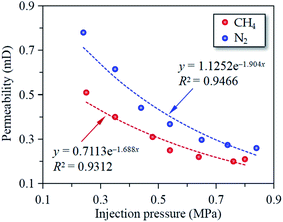 |
| Fig. 2 Coal permeability variation under different gas source and injection pressure conditions. | |
Due to the dual-porosity medium characteristic of coal, the pore matrix is bound to expand with gas adsorbed in coal. Considering that the surrounding and base of the experimental cavity are rigid, the adsorption swelling of the coal matrix leads to shrinkage of the internal seepage channels when a constant stress is applied to the top of the coal sample, which results in a continuous decrease in coal permeability. According to the Langmuir isothermal adsorption curves of coal, the coal adsorption capacity for gas increases with the rise of gas injection pressure, and the adsorption rate gradually decreases and stabilizes until the limit adsorption capacity no longer varies. However, as the pore pressure of coal goes up continuously, the effective stress on the coal sample is reduced. Resultantly, the initially compressed skeleton of the coal matrix expands, which causes the recovery of coal permeability. This phenomenon is also the main reason for the asymmetric U-shaped permeability curve in above-mentioned results. The finding shows that coal permeability change is mainly affected by the adsorption effect of the coal matrix under a low pore pressure. In contrast, the effective stress is dominant at a high pore pressure.
3.2 Coal permeability change during CH4 displacement by N2 injection
Above experimental results indicated that coal permeability variations under different gas injection conditions differ to some extent. Therefore, coal permeability is also in a changes dynamically during CH4 displacement by N2 injection. In this section, the change of coal permeability during displacement process was analyzed under different injection pressures. The initial gas pressure of the coal sample is 0.5 MPa, and the results are presented in Fig. 3.
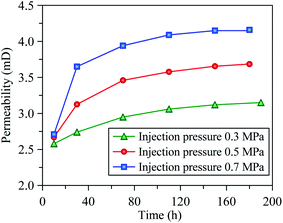 |
| Fig. 3 Coal permeability variation with different N2 injection pressures. | |
As displayed in figure, the variation curves of coal permeability during CH4 displacement by N2 injection both present an increasing trend with injection pressure, but the increase rate slows down gradually. Take 0.5 MPa of N2 gas injection pressure as an example, coal permeability surges rapidly in the initial displacement stage (10–70 min) from the initial 2.67 mD to 3.46 mD by the rate of 0.013 mD min−1. In the middle displacement stage (70–150 min), coal permeability grows slowly from 3.46 mD to 3.66 mD by a reduced rate of 0.003 mD min−1. In the later displacement stage (after 150 min), coal permeability gradually stabilizes at 3.68 mD. The variation curves of coal permeability with different N2 injection pressures reveal that a high gas injection pressure corresponds to a great increase in coal permeability. Based on the experimental data, as the N2 injection pressures are 0.3, 0.5, and 0.7 MPa, the coal permeability increases from 2.58, 2.67, and 2.71 mD to 3.15, 3.68, and 4.16 mD, which enhanced by 22.09%, 38.01%, and 53.51%, respectively over the same time span.
The above results indicate that CH4 displacement by N2 injection can increase the coal permeability. The reason is due to the coal adsorption capacity of N2 is weaker than that of CH4, thereby CH4 cannot be replaced through competitive adsorption. As a result, CH4 displacement by N2 injection causes enhances coal permeability by causing matrix shrinkage. However, the gas adsorption of coal belongs to physical adsorption which is reversible, that is, CH4 adsorption and desorption can reach to an equilibrium state. Once the CH4 pressure decreased, the original equilibrium will be broken, then the CH4 adsorbed on the surface of coal matrix micropores desorb as free gas to reach a new equilibrium, and coal permeability will be changed and finally tended to be stable. Therefore, N2 injection can achieve the replacement and displacement effect by reducing the effective partial pressure of CH4 and promotes its desorption.
3.3 Experimental results of CH4 displacement by N2 injection
The change curves of N2 injection, N2 discharge, and CH4 discharge volume during CH4 displacement by N2 injection are displayed in Fig. 4, which show that CH4 discharge volume changes in a trend of convex functional form, gradually transitioning from the rapid increase in the initial stage to stable at the late. However, N2 injection and discharge volume show opposite change trends, which both increase slowly in the initial stage and changed to a linear growth trend later. Comparing with the variation of three curves, we can find that a higher N2 injection pressure leads to a greater difference between N2 injection and discharge volume, that is, more residual amount of N2 is retained in the coal sample. Due to the limited gas adsorption capacity of the coal matrix, the increase in N2 residual amount results in the decrease of CH4 partial pressure. Depending on the Langmuir adsorption equation, the gas adsorption amount is positively correlated with gas pressure. Therefore, large amounts of adsorbed CH4 in coal will be desorbed to counter the reduction in CH4 partial pressure, thus promoting the displacement and production of CH4. According to the experimental results, the CH4 adsorption amount of coal sample reaches 36.57 L before N2 injection. While CH4 discharge volume corresponding to N2 injection pressure 0.3, 0.5, and 0.7 MPa at 120 min are 25.04, 29.38, and 29.50 L, with the CH4 output rate of 68.47%, 80.34% and 80.67%, respectively. Therefore, CH4 production also increases with N2 injection pressure at the same displacement time.
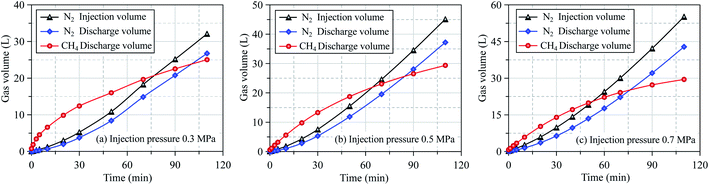 |
| Fig. 4 CH4 displacement by N2 injection under different injection pressures. | |
Combing with the results of coal permeability change during CH4 displacement by N2 injection, it can be found that with N2 injected into coal, a large amount of N2 molecules will be absorbed on the surface of the coal matrix. As a result, CH4 partial pressure falls and coal permeability soars sharply, which leads to CH4 molecules constantly desorbed from coal matrix and rapidly discharged after N2 replacement. During this process, the adsorption of N2 in coal becomes saturated, and coal permeability gradually stabilizes. In this case, the N2 injection, N2 discharge, and CH4 output varies linearly. The experimental results indicate that the coal permeability is a major factor affecting the CH4 displacement effect. Therefore, the dynamic evolution of coal permeability during displacement should be explored for CBM extraction and production.
4 Dynamic evolution mechanism of coal permeability during CH4 displacement by N2 injection
4.1 Dynamic evolution mechanism of coal permeability
The above-mentioned experimental results show that coal permeability changes dynamically during CH4 displacement by N2 injection and that the change of coal permeability directly affects the effect of CH4 discharge and production. As shown in Fig. 5, as a dual-porosity medium, coal comprises micropore-containing matrix and fracture systems. Injection of a certain pressure of N2 into coal decreases the CH4 partial pressure and increases the N2 partial pressure. For this reason, CH4 molecules desorb constantly from the adsorption state into the free state and spread to the fracture systems, while N2 molecules shift from the free state into the adsorption state and enter into the pores of the coal matrix. At the macro level, N2 displaces CH4 on the pore surface, and then matrix swelling/shrinkage is caused by N2 adsorption and CH4 desorption (Fig. 5a and b), thereby affecting the pore volume change of the coal matrix. On the other hand, the injection of N2 raises the pressure of CH4 and N2 gas mixture in the fracture system. According to Terzaghi's effective stress principle, the effective stress of coal will also changed. Relevant researches have disclosed that the coal skeleton deformation induced by the change in effective stress corresponds to the change in the volume of the fracture system, i.e., the deformation of the coal skeleton volume.31,32 Therefore, the coal permeability during displacement is mainly affected by both matrix swelling/shrinkage and effective stress.
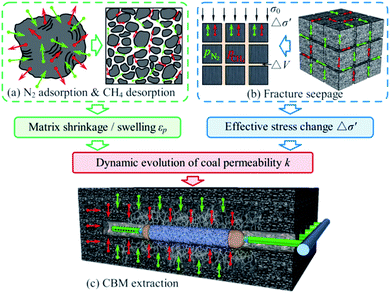 |
| Fig. 5 Evolution mechanism of coal permeability during CH4 displacement by N2 injection. | |
4.2 Effect of matrix swelling/shrinkage and effective stress on coal permeability
4.2.1 Effect of matrix swelling/shrinkage on coal permeability. Previous studies indicated that gas adsorption or desorption occurs in the coal matrix as a result of the change in chemical energy on the pore surface. Once gas adsorption, the free tension of the pore surface will be decreased, thus leading to the decline in the attraction between coal molecules. Thereby, the intermolecular distance enlarged. Macroscopically, this phenomenon is reflected by matrix swelling. In contrast, during gas desorption, the surface chemical energy enhances matrix shrinkage.Based on the surface chemistry theory,32 the change in free energy caused by gas adsorption and desorption on the coal surface can be expressed by Gibbs equation:
|
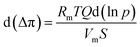 | (2) |
where d(Δπ) is the change in free energy;
Rm is Prussian gas constant, 8.31/(mol K);
T is the temperature, K;
Q is adsorption amount of gas;
S is specific pore surface area; and
Vm is mole volume of gas, 22.4 mol L
−1.
Since N2 partial pressure rises from 0 to p2 and CH4 partial pressure drops from p10 to p1, the integral of eqn (2) can be obtained as follows:
|
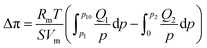 | (3) |
The adsorption capacity of binary gas in coal conforms to the Langmuir adsorption equilibrium equation:
|
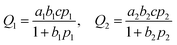 | (4) |
where
a1,
b1,
a2 and
b2 are the Langmuir adsorption constants of CH
4 and N
2, respectively, m
3 t
−1 and Pa
−1;
c is combustibles mass per unit volume of coal, t m
−3;
Q1 and
Q2 are adsorption amount of CH
4 and N
2, respectively.
The variation in free energy on coal surface caused by gas adsorption and desorption can be obtained by simultaneously calculating eqn (2)–(4):
|
 | (5) |
Assuming that coal is under completely ideal constraints, the matrix swelling/shrinkage model of gas adsorption/desorption can be obtained by the principle of elasticity mechanics and energy conservation:33
|
 | (6) |
where
K is bulk modulus, Pa; and
εp is volumetric strain of the coal matrix.
By substituting eqn (5) into eqn (6), the following functional relationship between the volumetric strain of the coal matrix and the thermodynamic parameters can be yielded:
|
 | (7) |
Relevant theoretical and experiments verification reveals that over two-thirds of total adsorption swelling strain is the internal swelling strain variable that changes the fracture volume.34 Assuming that matrix swelling/shrinkage deformation caused during gas adsorption/desorption is reversible. Therefore, during the displacement process, the CH4 partial pressure falls from p10 to p1, while the N2 partial pressure rises from 0 to p2. Then, the volumetric strain of the coal matrix can be expressed as:
|
 | (8) |
4.2.2 Effect of effective stress on coal permeability. According to Terzaghi's principle, the difference between the overlying strata stress acting on the coal reservoir and the fluid pressure in pores and fractures is the effective stress, which can be given as:35where σ′ is the effective stress of the coal seam, MPa; σ0 is the overlying strata pressure, MPa; α is Boit's coefficient; and p is the fluid pressure in pores and fractures, MPa.During the process of CH4 displacement by N2 injection, when the CH4 partial pressure decreases from p10 to p1, the effective stress increases by
Similarly, when N2 partial pressure increases from 0 to p2, the effective stress decreases by
Therefore, the Δσ′ variation can be obtained as follows:
|
Δσ′ = α(p10 − p1 + p2)
| (10) |
Given that the change in effective stress caused by the change in N2 and CH4 gas mixture pressure only induces the volumetric strain of coal skeleton, without altering the swelling/shrinkage amount of coal matrix, thus the coal strain caused by the effective stress variation can be expressed as:11
|
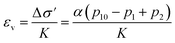 | (11) |
where
εv is volumetric strain of the coal.
4.2.3 Dynamic evolution model of coal permeability. Based on the above analysis, the variation in effective stress only changes the volumetric strain of coal skeleton, whereas the matrix swelling/shrinkage alters the volumetric strain of coal matrix. According to the porosity definition,36 coal porosity equation considering the integrated control of matrix swelling/shrinkage and effective stress can be expressed as follows: |
 | (12) |
Substituting eqn (7) and (10) into (12), we can obtain the porosity evolution model during CH4 displacement by N2 injection.
|
 | (13) |
According to the fracture plate model, the model of coal permeability under the combined effects of effective stress and adsorption swelling effect can be expressed by Kozeny–Carman equation.37
|
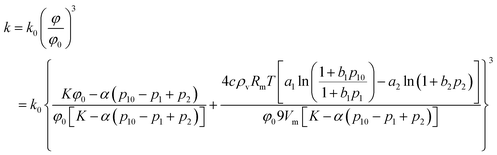 | (14) |
5 Model validation and discussion
5.1 Establishment of numerical simulation
To verify the correctness of the dynamic evolution model of coal permeability, the numerical simulation by COMSOL Multiphysics software was used to match the results between the simulation outcome and experimental data. For the numerical simulation, the theoretical model was embedded in the PDEs module in the form of partial differential to realize secondary development of the software. And then the physical geometric model of the coal sample with the size of 100 mm × 300 mm was built on the base of actual experimental conditions, as shown in Fig. 6.
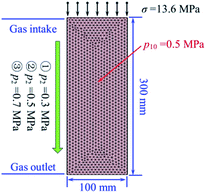 |
| Fig. 6 Physical geometric model. | |
The top of the physical model was subjected to uniformly distributed load, the bottom and surrounding boundaries were constrained by vertical or horizontal forces. In the initial state, the adsorption equilibrium pressure of CH4 gas in the coal sample was 0.5 MPa. The injected N2 flows through the model from top to bottom, and the displaced CH4 was discharged from the bottom. The transient solution method was adopted in the numerical calculation.
The main parameters for model calculation are listed in Table 3.
Table 3 Main parameters for numerical simulation
Parameter |
Value |
Initial porosity φ0 |
0.08 |
Initial permeability k0 |
2.55 mD |
CH4 dynamic viscosity coefficient μ1 |
1.03 × 10−5 Pa s |
CH4 Langmuir constant a1 |
29.41 kg m−3 |
CH4 Langmuir constant b1 |
2.25 MPa−1 |
Coal apparent density ρv |
1.49 × 103 g m−3 |
Biot α |
0.85 |
N2 dynamic viscosity coefficient μ2 |
1.69 × 10−5 Pa s |
N2 Langmuir constant a2 |
23.15 kg m−3 |
N2 Langmuir constant b2 |
1.37 MPa−1 |
5.2 Analysis of numerical simulation results
Fig. 7 displays the cloud image change of coal permeability during CH4 displacement by N2 injection. The graph demonstrates that coal permeability at the initial time (t = 0 min) is equivalent to that of the original state of coal seam unaffected by mining disturbance, with coal permeability is only 2.55 mD.
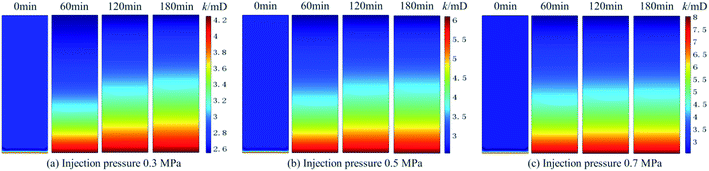 |
| Fig. 7 Cloud image change of coal permeability. | |
While with the passage of displacement time, the coal permeability increases continuously, but the increased extent gradually reduces. Since coal adsorption capacity of N2 is weaker than that of CH4, the coal matrix shrinks and the permeability increases after N2 replaces CH4. However, as N2 injection proceeds, the adsorption capacity of the coal matrix becomes saturated. As a result, the strain variation of the coal matrix continuous lowered, thereby coal permeability stabilizes.
Comparison of the simulation results under different N2 injection pressures can be found that the higher the N2 injection pressure is, the shorter the time is required for coal permeability to reach the equilibrium state, and the greater increase of permeability is correspondingly. Such a phenomenon demonstrates that raising N2 injection pressure can significantly enhance the binary gas convection velocity and form a high gas concentration gradient, thus reducing the displacement time. Eqn (10) indicates that a higher N2 injection pressure corresponds to a smaller effective stress and a larger coal strain, thus leading to a continuously expanding seepage space within coal. Therefore, coal permeability is directly proportional to the gas injection pressure, that is, it increases with the rise of gas injection pressure.
To verify the accuracy of the theoretical model, Fig. 8 presents the matching results between simulation outcome and experimental data of coal permeability during CH4 displacement by N2 injection. As can be observed in figure, the both results share the same change regularity. Coal permeability increases by a gradually decelerating rate with the passage of displacement time and finally stabilizes. The numerical simulation outcome is consistent with the experimental data, thus indicating that the theoretical model can reflect the dynamic evolution of coal permeability during N2-ECBM process with highly applicable levels.
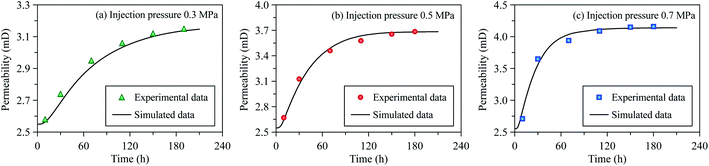 |
| Fig. 8 Matching results between simulation outcome and experimental data. | |
5.3 Discussion
Experimental results of coal permeability change during displacement process show that N2 injection can enhance coal permeability and promote CH4 production and displacement in coal with a positive effect. Therefore, the dynamic evolution model of coal permeability proposed in this work, which comprehensively considers the combined effects of matrix swelling/shrinkage and effective stress, expounds the basic physical process of binary gas relative flow in the process of CH4 displacement by N2 injection. Eqn (14) indicates that with the continuous injection of high-pressure N2 leads to the decrease in CH4 concentration and the increase in N2 concentration in fracture space, thereby promoting the rapid diffusion of free CH4 in the pores and the desorption of adsorbed CH4 molecule. Compared with the current engineering methods, such as hydraulic fracturing, hydraulic slotting and hydraulic flushing, can significantly improve coal permeability, but the resultant “water-lock” effect may slow down CH4 diffusion and desorption. In contrast, the method of CH4 displacement by N2 injection can avoid the above-mentioned engineering problems and boasts the advantage for enhancing CBM recovery. Based on the theoretical model and COMSOL numerical simulation method proposed in this work, the ECBM effect of N2 injection and CH4 discharge volume can be predicted. Therefore, the findings provide an important basis for the design and optimization of displacement engineering and thus serves as a feasible method for ECBM engineering application.
Be noted that, considering the actual influence of geological tectonics and mining activities on coal seam, the large-size raw coal is difficult to collect from the on-site and process into experimental samples as conditions limited. Due to the little discreteness and high repeatability of pulverized coal samples for investigating, this sample is instead of raw coal in this work. Furthermore, related studies also indicate that the permeability response characteristics of both coal samples are basically similar, with little differences in details.38,39 Besides that, the experimental data of coal permeabilities to CH4 and N2 in this work are consistent with researches of Zhao et al.,30 indicating that the pulverized coal samples can be used to investigate the mechanism of N2-ECBM recovery for providing some guiding significance in engineering application. Therefore, the dynamic evolution model of coal permeability proposed in this work can reflect generally change of coal permeability during CH4 displacement by N2 injection. On the basis of the this study, we will conduct relevant field tests in future work to properly modify the theoretical model of eqn (14) for improving the accuracy and applicability to guide actual project.
6 Conclusions
This study carried out a series of physical simulation experiments of CH4 displacement by N2 injection under different injection pressure conditions. And a dynamic evolution model of coal permeability considering the combined effects of matrix shrinkage/swelling and effective stress was proposed to reflect the ECBM recovery process. The main conclusions are obtained as following:
(1) The results of coal permeability tests under different gas source conditions show that coal permeability to N2 is generally higher than that of CH4, and the coal permeability changes in an asymmetric “U” pattern trend with the rise of N2 injection pressure. During CH4 displacement by N2 injection, coal permeability continues to increase at a decelerating rate with the passage of displacement time, and finally becomes stable. Increasing N2 injection pressure is conducive to improving coal permeability and thereby promoting CH4 production.
(2) Given that coal is a dual-porosity medium, the change in effective stress only changes the volumetric strain of coal skeleton, whereas the coal matrix swelling/shrinkage only alters the volumetric strain of the coal matrix. Based on the porosity equation, a dynamic evolution model of coal permeability considering the combined effects of matrix swelling/shrinkage and effective stress during N2-ECBM displacement process is established.
(3) The dynamic evolution model of coal permeability was embedded in the PDEs module of COMSOL Multiphysics software in the form of partial differential. Then, theoretical model was calculated by finite element solver to simulation N2-ECBM effect. And the simulation outcome is consistent with the experimental data, suggesting that the theoretical model can reflect the dynamic evolution of coal permeability during CH4 displacement by N2 injection. The findings of this work can provide an important guiding significance for the design and optimization of N2-ECBM displacement engineering.
Author contributions
All of the authors contributed significantly to this research. Bo Li: conceptualization, investigation, funding. Junxiang Zhang: conceptualization, methodology, investigation, software, writing-original draft. Zhiben Ding: writing-review & editing, supervision, data curation. Bo Wang: visualization, supervision. Peng Li: formal analysis, visualization, software.
Conflicts of interest
There are no conflicts to declare.
Acknowledgements
This work is supported by the National Natural Science Foundation of China (51874125 & 51804356), Research Fund of State and Local Joint Engineering Laboratory for Gas Drainage & Ground Control of Deep Mines (SJF202002), State Key Laboratory Cultivation Base for Gas Geology and Gas Control (WS2020A15), Key Specialized Research and Development Breakthrough in Henan Province (212102310597), Research Funds of Youth Talent and Independent Innovation Research of Zhongyuan University of Technology (K2020QN014 & K2020YY010), Young Key Teachers from Henan Polytechnic University (2019XQG–10), Young Talent Lift Project of Henan Province in 2020 (2020HYTP020), Henan Polytechnic University Foundation for distinguished young (J2020–4).
Notes and references
- L. H. Xu and C. L. Jiang, Initial desorption characterization of methane and carbon dioxide in coal and its influence on coal and gas outburst risk, Fuel, 2017, 203, 700–706 CrossRef CAS.
- Y. Z. Chen, J. Li, H. W. Lu and J. Xia, Tradeoffs in water and carbon footprints of shale gas, natural gas, and coal in China, Fuel, 2020, 263, 116778 CrossRef CAS.
- Y. Qin, A. M. Tim, J. Shen, Z. B. Yang, Y. L. Shen and G. Wang, Resources and geology of coalbed methane in China: A review, Int. Geol. Rev., 2018, 60, 777–812 CrossRef.
- P. G. Rutberg, V. A. Kuznetsov, V. E. Popov, S. D. Popov, A. V. Surov, D. I. Subbotin and A. N. Bratsev, Conversion of methane by CO2 + H2O + CH4 plasma, Appl. Energy, 2015, 148, 159–168 CrossRef CAS.
- J. X. Zhang, B. Li and Y. N. Sun, Dynamic leakage mechanism of gas drainage borehole and engineering application, Int. J. Min. Sci. Technol., 2018, 28, 505–512 CrossRef CAS.
- W. Ju, J. Shen, Y. Qin, S. Z. Meng, C. Li, G. Z. Li and G. Yang, In situ stress distribution and coalbed methane reservoir permeability in the Linxing area, eastern Ordos Basin, China, Front. Earth Sci., 2018, 12, 545–554 CrossRef CAS.
- E. I. Epelle and D. I. Gerogiorgis, A review of technological advances and open challenges for oil and gas drilling systems engineering, AIChE J., 2020, 66, e16842 CrossRef CAS.
- N. Li, B. X. Huang, X. Zhang, Y. Y. Tan and B. L. Li, Characteristics of microseismic wave forms induced by hydraulic fracturing in coal seam for coal rock dynamic disasters prevention, Saf. Sci., 2019, 115, 188–198 CrossRef.
- J. X. Zhang, B. Li and Y. N. Sun, Performance research of a new coal-dust-based composite sealing material for gas–drainage borehole, Mater. Express, 2018, 8, 325–334 CrossRef CAS.
- Q. L. Zou, B. Q. Lin, C. S. Zheng, Z. Y. Hao, C. Zhai, T. Liu, J. Y. Liang, F. Z. Yan, W. Yang and C. J. Zhu, Novel integrated techniques of drilling-slotting-separation-sealing for enhanced coal bed methane recovery in underground coal mines, J. Nat. Gas Sci. Eng., 2015, 26, 960–973 CrossRef CAS.
- Q. Zou and B. Lin, Fluid–solid coupling characteristics of gas-bearing coal subjected to hydraulic slotting: An experimental investigation, Energy Fuels, 2018, 32, 1047–1060 CrossRef CAS.
- C. G. Xu, J. Cai and Y. S. Yu, Effect of pressure on methane recovery from natural gas hydrates by methane–carbon dioxide replacement, Appl. Energy, 2018, 217, 527–536 CrossRef CAS.
- S. S. Tupsakhare and M. J. Castaldi, Efficiency enhancements in methane recovery from natural gas hydrates using injection of CO2/N2 gas mixture simulating in situ combustion, Appl. Energy, 2019, 236, 825–836 CrossRef CAS.
- A. Busch and Y. Gensterblum, CBM and CO2-ECBM related sorption processes in coal: a review, Int. J. Coal Geol., 2011, 87, 49–71 CrossRef CAS.
- S. J. Zheng, Y. B. Yao, D. Elsworth, D. M. Liu and Y. D. Cai, Dynamic fluid interactions during CO2-ECBM and CO2 sequestration in coal seams. Part I: CO2–CH4 interactions, Energy Fuels, 2020, 34, 8274–8282 CrossRef CAS.
- S. K. Sinha and S. D. Gupta, A geological model for enhanced coal bed methane (ECBM) recovery process: a case study from the Jharia coalfield region, India, J. Pet. Sci. Eng., 2021, 201, 108498 CrossRef CAS.
- H. X. Hu, L. Du, Y. F. Xing and X. C. Li, Detailed study on self- and multicomponent diffusion of CO2–CH4, gas mixture in coal by molecular simulation, Fuel, 2017, 187, 220–228 CrossRef CAS.
- L. D. Connell, R. Sander, Z. Pan, M. Camilleri and D. Heryanto, History matching of enhanced coal bed methane laboratory core flood tests, Int. J. Coal Geol., 2011, 87, 128–138 CrossRef CAS.
- Z. D. Liu, Y. P. Cheng, Y. K. Wang, L. Wang and W. Li, Experimental investigation of CO2 injection into coal seam reservoir at in–situ stress conditions for enhanced coalbed methane recovery, Fuel, 2019, 236, 709–716 CrossRef CAS.
- J. J. Kolak and R. C. Burruss, Geochemical investigation of the potential for mobilizing non-methane hydrocarbons during carbon dioxide storage in deep coal beds, Energy Fuels, 2006, 20, 566–574 CrossRef CAS.
- A. S. Ranathunga, M. S. A. Perera, P. G. Ranjith and C. H. Wei, An experimental investigation of applicability of CO2 enhanced coal bed methane recovery to low rank coal, Fuel, 2017, 189, 391–399 CrossRef CAS.
- Q. S. Zeng, Z. M. Wang, L. Q. Liu and J. P. Ye, Modeling CH4 displacement by CO2 in deformed coalbeds during enhanced coalbed methane recovery, Energy Fuels, 2018, 32, 1942–1955 CrossRef CAS.
- Z. Z. Yang, S. Yang, J. X. Han and X. G. Li, Molecular simulation on competitive adsorptions of CO2, CH4, and N2 in deep coal seams, Chem. Technol. Fuels Oils, 2020, 56, 619–626 CrossRef CAS.
- Z. Bing, J. P. Ye, Y. Li, X. T. Han, J. Shen and C. Cao, Simulation of key parameters in CO2 injection for enhanced coalbed methane recovery in a deep well group, Energy Sources, Part A, 2018, 40, 2219–2226 CrossRef CAS.
- C. J. Fan, D. Elsworth, S. Li, Z. W. Chen, M. K. Luo, Y. Song and H. H. Zhang, Modelling and optimization of enhanced coalbed methane recovery using CO2/N2 mixtures, Fuel, 2019, 253, 1114–1129 CrossRef CAS.
- S. A. Mathias, S. Nielsen and R. L. Ward, Storage coefficients and permeability functions for coal-bed methane production under uniaxial strain conditions, Transp. Porous Media, 2019, 130, 627–636 CrossRef CAS.
- Y. Li, Z. Yang and X. G. Li, Molecular simulation study on the effect of coal rank and moisture on CO2/CH4 competitive adsorption, Energy Fuels, 2019, 33, 9087–9098 CrossRef CAS.
- X. D. Du, M. Gu, Z. J. Liu and Y. Zhao, Enhanced shale gas recovery by the injections of CO2, N2 and CO2/N2 mixture gases, Energy Fuels, 2019, 33, 5091–5101 CrossRef CAS.
- K. H. Shi, E. E. Santiso and K. E. Gubbins, Bottom-up approach to the coarse-grained surface model: Effective solid–fluid potentials for adsorption on heterogeneous surfaces, Langmuir, 2019, 35(17), 5975–5986 CrossRef CAS.
- Y. S. Zhao, Y. Q. Hu, D. Yang and J. P. Wei, Experimental study of the gas seepage law of rock related to adsorption under 3D stresses, Chin. J. Rock Mech. Eng., 1999, 18, 651–653 Search PubMed.
- J. P. Wei, B. Li, K. Wang and D. H. Sun, 3D numerical simulation of boreholes for gas drainage based on the pore-fracture dual media, Int. J. Min. Sci. Technol., 2016, 26, 739–744 CrossRef.
- C. Liu and Y. G. Zhang, Interpretation of Gibbs surface excess model for gas adsorption on heterogeneous coal particle, Fuel, 2018, 214, 20–25 CrossRef.
- P. Guo, S. G. Cao, Z. G. Zhang, F. Luo and Y. B. Liu, Theoretical study of deformation model of coal swelling induced by gas adsorption, Rock Soil Mech., 2014, 35, 128–133 Search PubMed.
- J. P. Zhou, X. F. Xian and Y. D. Jiang, A model of adsorption induced coal deformation based on thermodynamics approach, J. China Coal Soc., 2011, 36, 468–472 CAS.
- A. Gilman and R. Beckie, Flow of coal-bed methane to a gallery, Transp. Porous Media, 2000, 41, 1–16 CrossRef CAS.
- Z. Sun, J. T. Shi, T. Zhang, K. L. Wu, D. Feng, F. R. Sun, L. Huang, C. H. Hou and X. F. Li, A fully-coupled semi–analytical model for effective gas/water phase permeability during coal-bed methane production, Fuel, 2018, 223, 44–52 CrossRef CAS.
- J. X. Zhang, Y. W. Liu, P. L. Ren, H. K. Han and S. Zhang, A fully multifield coupling model of gas extraction and air leakage for in-seam borehole, Energy Rep., 2021, 7, 1293–1305 CrossRef.
- S. G. Li, Y. Li, P. Guo, Y. J. Yan and Y. B. Liu, Comparative research on permeability characteristics in complete stress–strain process of briquettes and coal samples, Chin. J. Rock Mech. Eng., 2010, 29, 899–906 Search PubMed.
- H. Y. Jia, K. Wang, Y. B. Wang and K. X. Sun, Permeability characteristics of gas-bearing coal specimens under cyclic loading–unloading of confining pressure, J. China Coal Soc., 2020, 45, 1710–1718 Search PubMed.
|
This journal is © The Royal Society of Chemistry 2021 |