DOI:
10.1039/D1RA02557K
(Review Article)
RSC Adv., 2021,
11, 17809-17827
Biomaterial design strategies to address obstacles in craniomaxillofacial bone repair
Received
31st March 2021
, Accepted 10th May 2021
First published on 17th May 2021
Abstract
Biomaterial design to repair craniomaxillofacial defects has largely focused on promoting bone regeneration, while there are many additional factors that influence this process. The bone microenvironment is complex, with various mechanical property differences between cortical and cancellous bone, a unique porous architecture, and multiple cell types that must maintain homeostasis. This complex environment includes a vascular architecture to deliver cells and nutrients, osteoblasts which form new bone, osteoclasts which resorb excess bone, and upon injury, inflammatory cells and bacteria which can lead to failure to repair. To create biomaterials able to regenerate these large missing portions of bone on par with autograft materials, design of these materials must include methods to overcome multiple obstacles to effective, efficient bone regeneration. These obstacles include infection and biofilm formation on the biomaterial surface, fibrous tissue formation resulting from ill-fitting implants or persistent inflammation, non-bone tissue formation such as cartilage from improper biomaterial signals to cells, and voids in bone infill or lengthy implant degradation times. Novel biomaterial designs may provide approaches to effectively induce osteogenesis and new bone formation, include design motifs that facilitate surgical handling, intraoperative modification and promote conformal fitting within complex defect geometries, induce a pro-healing immune response, and prevent bacterial infection. In this review, we discuss the bone injury microenvironment and methods of biomaterial design to overcome these obstacles, which if unaddressed, may result in failure of the implant to regenerate host bone.
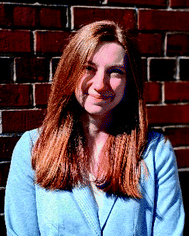 Marley J. Dewey | Marley Dewey is a recent graduate from the Harley Lab at the University of Illinois Urbana-Champaign. She received her B.S. in Chemical Engineering from the University of Maine (2016) and her PhD in Materials Science and Engineering from the University of Illinois Urbana-Champaign (2021). Her research in the Harley Lab involves regeneration of craniomaxillofacial bone defects by modification of mineralized collagen scaffolds. She investigates their potential to inhibit bacterial surface attachment, modulate the immune response to repair, promote multiple cell types to synergistically repair bone, and incorporate 3D-printed structures into the collagen scaffolds to create composite materials with improved mechanics. |
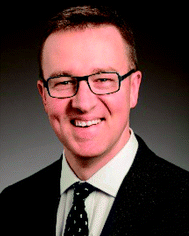 Brendan A.C. Harley | Brendan Harley is the Robert W. Schaefer Professor in the Dept. of Chemical and Biomolecular Engineering at the University of Illinois Urbana-Champaign. He received a B.S. in Engineering Sciences from Harvard University (2000), a Sc.D. in Mechanical Engineering from MIT (2006), and performed postdoctoral studies at the Joint Program for Transfusion Medicine at Children's Hospital Boston (2006–2008). His research group develops biomaterial platforms to instruct endogenous cell activities in the context of musculoskeletal and craniofacial tissue regeneration, hematopoietic stem cell biomanufacturing, as well as to investigate endometrial pathologies and invasive brain cancer. |
Craniomaxillofacial bone defects
Craniomaxillofacial (CMF) bone defects often involve large defects in the bones that make up the skull or jaw, and can arise from trauma associated with high-energy impacts, congenital defects, and cancer.1,2 Congenital defects, such as cleft lip and palate, have a frequency of 1 in 700 live births, and oral cancer and dentures can lead to bone resection or resorption by the body.2 The occurrence of these defects in times of war has increased in recent years, with 29% of injuries sustained in Iraq and Afghanistan classified as CMF defects.3 Due to the critical size of missing bone in these defects, host bone is unable to naturally bridge the gap in missing tissue and regenerate fully, and thus surgical intervention is required for successful healing. Multiple factors lead to additional challenges in healing of these defects, such as their irregular size and shape, multiple cell types involved, and the likelihood of chronic inflammation and infection, which will be discussed in more detail later in this chapter.
The bone microenvironment
Bone is a complex structure composed of multiple cell types and having various mechanical properties. Of note, bones of the skull and jaw have different mechanics and structure than long bones and the spinal column.
Bone is comprised of organic and inorganic materials, with type I collagen fibers and glycosaminoglycans making up the organic material, and hydroxyapatite mineral crystals as the inorganic. Bone is also anisotropic in nature, with mechanical properties varying in the direction of load application.4 There exist two different types of bone, cortical and cancellous bone, which have similar compositions but different structural properties. Cortical bone is the stronger of the two and surrounds the softer cancellous bone. Cortical bone generally has a Young's Modulus between 15–20 GPa and approximately 10% porosity, while cancellous bone has a 10-fold weaker Young's Modulus between 0.1–2 GPa and a high porosity of 50–90%.5,6 For skull bones in particular, stiffnesses can range from 0.36–6 GPa, and variability can be attributed to differences in thickness of the skull at various regions.7 Thicknesses ranging from 3–15 mm have been observed in the occipital region, with an average of 8 mm thickness in the occipital region and 4 mm in the temporal.8 Additionally, the surrounding soft tissue of the periosteum has an impact on these mechanics and is rarely investigated together with the bone.9 Based on a small study of human skull bones the volume ratio of cancellous bone to the entire bone volume ranged from 0.7–0.8,9 and although the cancellous portion of bone is much weaker, the open-porous nature allows quick invasion of blood vessels and nutrient transport.5 Without this vascular formation bone will become necrotic, leading to resorption and bone loss.5,10
Aside from mechanics, the bone microenvironment is composed of multiple cell types, all which act together to maintain healthy bone homeostasis. These include cells important for new bone formation, vascular formation, and bone resorption. Cells involved in bone formation and maintenance include mesenchymal stem cells, osteoblasts, and osteocytes (Fig. 1).
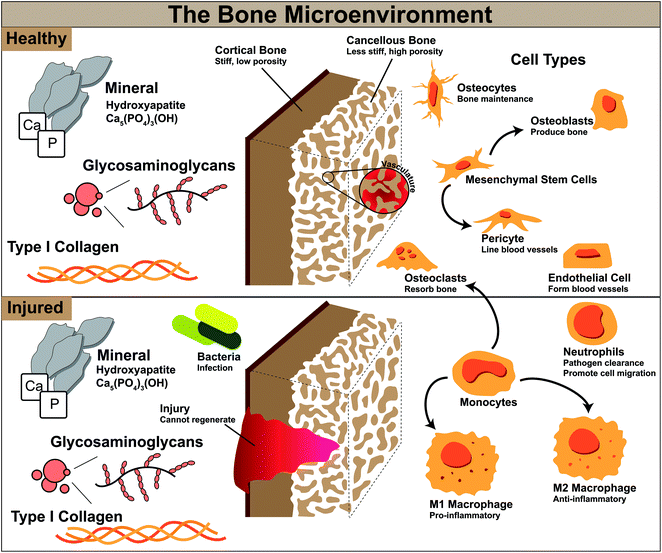 |
| Fig. 1 Cell types involved in bone homeostasis and during injury and their functions. | |
Mesenchymal stem cells (MSCs) are a cell type which can self-replicate and differentiate into many different cell types such as bone, cartilage, muscle, fat, and tendon, and are known to migrate to sites of injury to aid in repair.11,12 Differentiation of these stem cells along the bone lineage can result in osteoblasts, which are required to form new bone by secretion of bone matrix proteins.5 When osteoblasts mature they are incorporated into the bone matrix and become osteocytes, which remain within the matrix and have been associated with bone turnover and adaption.13 Endothelial cells and pericytes are important for vasculature formation to deliver nutrients and other cell types throughout bone. Pericytes originate from MSCs and line the outside of blood vessels, and endothelial cells form tubes which make up these vessels.14 Angiogenesis has been associated with osteogenesis, and construction of highly vascular networks within bone leads to its successful maintenance.15 Finally, osteoclasts are responsible for bone resorption. Osteoblasts and osteoclasts work together to maintain bone homeostasis, maintaining normal bone density, porosity, and strength. Without osteoclasts ectopic or excess bone could occur and without osteoblasts bones may become brittle and thin.16,17 These various cell types work together synergistically to maintain healthy bone in our body, and without one cell type or its functions our bone and our bodies would not be able to function normally.
The bone injury microenvironment
Bone is a complex microenvironment and healing these defects is particularly challenging due to the multiple cell types and various mechanical properties. CMF defects introduce an additional challenge due to the large volume of bone missing and the body's inability to heal this on its own.
In general, bones heal via a process known as endochondral ossification or intramembranous ossification. These two processes have similar healing outcomes; however, endochondral ossification involves a cartilage intermediate associated mostly with long bone healing, while intramembranous ossification does not involve cartilage formation and is associated with the flat bones of the skull and jaw.2,18,19 Many methods to regenerate bone focus on the direct method of bone formation, intramembranous ossification, where mesenchymal stem cells directly differentiate to osteoblasts. Conversely, endochondral ossification is a seemingly side-step away from bone repair by first creating a cartilage intermediate and mesenchymal stem cells differentiating into chondrocytes. This may not be a drawback however, as cartilage intermediates and chondrocytes formed are avascular and do not need as many nutrients as osteoblasts, and are more likely to survive the process or bone regeneration.2,20 Further, it has even been suggested that using an endochondral approach to repair CMF defects by promoting a cartilage intermediate, along with neural crest-derived stem cells (from hair follicles, oral mucosa, dental pulp, among others), could prove a more promising approach to CMF defect repair.2 An understanding of a materials method of regenerating CMF defects, by intramembranous or endochondral ossification, could be useful for developing modifications to the material to enhance osteogenesis.
At the onset of injury, there are other cell types involved in repair beyond those in normal bone homeostasis represented in Fig. 1. Bone healing occurs in stages; for segmental defects such as CMF defects, this process can take several months to complete. To heal these defects, substantial bone or a bone-mimicking biomaterial needs to be added to the wound site to bridge the gap in missing bone and regenerate this space. In the first stage after surgical implantation of additional bone or biomaterial to the defect, a hematoma is formed and inflammation begins, transporting with it various immune cells and mesenchymal stem cells (Fig. 2). During this stage, bacteria can be easily introduced within the implanted material if not sterilized properly, or from surrounding patient skin and contamination of surgical tools. Neutrophils are the first immune system cell to migrate to the site of tissue damage and release antimicrobials to kill pathogens, as well as release cytokines to recruit other immune cell types and promote angiogenesis.21 Failure to regenerate bone can occur if pathogens cannot be cleared by neutrophils and can result in a bacterial biofilm which can be difficult to eliminate by the body and by antibiotics. This can result in persistent inflammatory stimuli as the body works to clear it, and often abscess formation, ultimately leading to chronic infection and the need for a subsequent surgery to remove infected tissue and restart the bone regeneration process.22
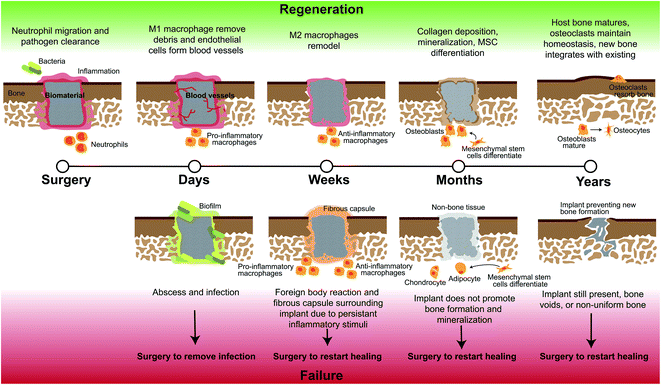 |
| Fig. 2 Stages of craniomaxillofacial bone defect regeneration with biomaterial implants and the possible routes of failure. Full regeneration of these defects can occur over the course of years and from the early to late stages of regeneration there are multiple instances of regeneration failure and when any of these failures occur, the biomaterial most likely will need to be removed and regeneration restarted with a new surgery and material. | |
Monocytes travel to the wound site from the bone marrow and can differentiate into osteoclasts to stimulate bone resorption or M0, unpolarized, macrophages, which can later differentiate into various phenotypes based on environmental cytokines and proteins.16 Macrophages activate in response damaged tissue signals, and during a healthy immune response, undifferentiated macrophages migrate to the wound site and polarize to the M1, or “pro-inflammatory,” phenotype in the early stages (1–7 days).23–25 The M1 phenotype is activated by interferon gamma (IFNγ), lipopolysaccharide (LPS), or tumor necrosis factor alpha (TNFα).23 M1 macrophages function to produce inducible nitric oxide synthase (iNOS), reactive oxygen species, and inflammatory cytokines,23 and are responsible for assisting in early blood vessel formation by VEGF production and removal of debris. After a few days and continuing for weeks, M1 macrophages shift in phenotype to M2 macrophages, also classified as “pro-healing” or “anti-inflammatory,” which can be induced by IL-4, IL-10, and IL-13 cytokines.23 M2 macrophages function to remodel the tissue, deposit new extracellular matrix, and secrete PDGF-BB to assist in late-stage blood vessel development.24,25 The M1 to M2 transition can occur over the course of weeks, and is important in avoiding persistent or chronic inflammation, which likely occurs in untreated CMF defects where M1 macrophages will persist, and can lead to a foreign body reaction and ultimate need for a secondary surgery.26,27 If these macrophages or neutrophils are still present after months, this can be classified as persistent inflammation and limited bone healing will occur, as they will continue to produce inflammatory cytokines; without neutrophil apoptosis, tissue damage can occur through continued release of factors meant for pathogen clearance.21 Additionally, in the case of implanted materials, a foreign body reaction will occur and if the body continues to react to the implant with inflammatory stimuli this can lead to macrophage fusion and surrounding the implant with fibrous tissue and inhibiting bone formation. After inflammation recedes during normal wound repair, mesenchymal stem cells differentiate and mature, and deposit matrix to form bone.28,29 Finally, secondary bone formation occurs by osteoclast-mediated bone resorption in order to create the anisotropic nature of bone and maintain healthy amounts of bone within the body.28
Current standards for repairing bone defects
The gold standard for repairing most CMF defects is via the use of bone grafts, and includes both allogenic and autologous sources of bone.
Autografts
Autografts use bone from a secondary site in the patient's own body to replace bone missing in the primary CMF wound site, creating the need for a minimum of two surgeries to attain the bone graft. The most common bone used is the iliac crest, and typically has success rates ranging from 70% to 95%.30 Removing bone from another area of the patient's body leads to drawbacks such as pain, vascular and nerve injury, bone fracture, and high chance of bone morbidity.1 Additionally, the large amount of bone necessary for CMF defect repair can limit the amount of bone usable in a patient's own body, and differences in patient health and age can lead to variable healing outcomes.31 Overall, autografts have the highest success rate in the clinic, attributed to osteogenic and other cell retention in the graft and a desired acute immune response to a material familiar to the body.1
Allografts
Allografts use bone commonly from a deceased donor, with cellular materials removed and bone pre-processed into demineralized bone matrix as blocks or particles before implantation.1 Pathogenic agents and genetic material must be removed prior to implantation to minimize disease transmission and a persistent inflammatory response, which includes heavy processing of the allograft. However, during this cleaning process, osteogenesis of the graft can be impacted as the extracellular matrix (ECM) and collagen can be removed, and this leads to variabilities in healing due to commercial supplier cleaning process differences.32,33 Drawbacks to allografts include high rates of infection even after sterilization due to foreign substances still remaining after processing, and a more vigorous approach to remove these leads to the bone being less osteogenic.34 The rate of success of allografts is lower than autografts, but avoids the limitations of a second invasive surgery and limited availability of autografts.35
The disadvantages associated with the use of autografts and allografts promote the research and development of tissue engineered biomaterials. Biomaterial approaches allow for patient-tailorable options as well as these typically being easier to modify, enabling changes in mechanics, bioactivity, and drug-loading to improve regeneration.
Biomaterials to repair orthopedic defects
Biomaterials are implants that can be discretely designed to optimize mechanics and biological signals to one day offer the same or better healing than autograft and allograft methods. The greatest advantage of biomaterials is their tailorable nature, allowing for researchers to change multiple properties and add various materials to optimize bone growth. Currently, autograft materials are still the gold standard for CMF defect repair due to highest successful outcomes, but the significant limitations of autografts promotes the discovery and creation of new biomaterials without these limitations.36–38
Biomaterials are fabricated from either polymers, metals, or ceramics, and often combinations of multiple material types. These are summarized in Table 1.
Table 1 Benefits and drawbacks of materials used for bone repair
Material |
Sub-class |
Benefits |
Drawbacks |
Novel developments |
Metals |
Stainless steel, titanium |
High load bearing |
• Permanent fixture |
• Surface coatings |
• Stress-shielding |
• Nanopatterned surfaces |
• Risk of infection |
|
• Secondary surgery to remove implant |
|
• Limited osseointegration |
|
Magnesium |
• High load bearing |
• Rapid dissolution of metal |
Addition of other metal ions |
• Biodegradable |
• Implant failure |
|
|
• Risk of infection |
|
Zinc |
• Biocompatbile |
• Low mechanical properties |
• Porous structures |
• Antibacterial |
• Releases large zinc ions harmful to cells |
• Calcium phosphate coatings |
Ceramics |
Bioglass |
• Bioactive |
• Brittle |
Metal doping |
• Osteoconductive |
• Low fracture toughness |
|
• Integration with host bone |
• Poor osteoinductivity |
|
• Antibacterial |
|
|
Calcium phosphates |
• Osteoinductive |
• Brittle |
• Metal doping |
• Resorbable |
• Slow resorption |
• Addition to polymers as coatings |
• Injectable as a cement, shapeable |
• Limited mechanical strength |
|
|
• Risk of infection |
|
Silica nanomaterials |
• Low cytotoxicity |
• Crystallinity impacts biocompatibility |
• Surface modifications |
• High porosity |
• Aggregation of nanoparticles |
• Combination with polymers |
• High mechanical strength |
• High concentrations can lead to particle setting and cytotoxic effects |
|
• Biocompatible |
• Concentration limits |
|
• Tunable pore size |
• Risk of infection |
|
• Drug delivery vehicles |
|
|
• Osteogenic |
|
|
• Promotes vasculature |
|
|
Polymers |
Polylactic acid (PLA) |
• Biocompatible |
• Acidic degradation products may cause inflammation |
• Coat with calcium phosphate |
• Biodegradable |
• Risk of infection |
• Blend with multiple polymers |
• Easily 3D-printed into specific shapes and porosities |
|
|
• Shorter degradation time than PCL (6 + months) |
|
|
• High mechanical properties |
|
|
Polycaprolactone (PCL) |
• Flexible |
• Low mechanical stiffness |
• Blend with multiple polymers |
• Hydrophobic |
• Long degradation times |
• Use different polymer conformations (star) |
• Biodegradable |
• Acidic degradation products |
|
• Biocompatible |
• High transition temperature for shape actuation |
|
• Easily 3D-printed into specific shapes and porosities |
• Risk of infection |
|
• Shape-memory fabrication |
|
|
Collagen |
• Tunable pore size |
• Low mechanical properties |
• Reinforce with stronger materials |
• Biocompatible |
• Disease transmission risk |
• Collagen derived from marine sources |
• Sequester growth factors easily |
• Need mineral to induce osteogenesis |
• Add calcium phosphate |
|
• Risk of infection |
|
Chitosan |
• Antibacterial |
• Poor mechanical properties |
• Reinforce with stronger materials |
• Anti-inflammatory |
• Low cell attachment |
• Modify fabrication (granular hydrogels) |
|
• Poor osteoconductivity |
|
|
• Need mineral to induce osteogenesis |
|
Metals
Metals have been classically used in CMF defect repair for permanent solutions to fill missing tissue. Metal implants generally can conduct heat, create difficulties with monitoring health via imaging systems, and their stiffness can cause stress-shielding.34 Additionally, most metals have a risk of corrosion and metal ion release, as well as mismatched mechanics compared to bone, which can lead to surrounding bone atrophy.39–41 Generally, metals are limited for use in permanent fixation for high loading applications, such as long bone fractures, as opposed to CMF defects. The non-degradable nature of metals also limits their use in pediatric patients due to facial deformities arising from restriction of the growing and developing skull and migration of the metal screws and plates during this process.42 The most commonly used metal in CMF defects are stainless steel and titanium-based alloys.43 Titanium is one of the strongest biomaterials used in bone repair, however, for non-load bearing CMF defects such as the skull, this high strength is unnecessary. Additionally, this material is a permanent fixture and has poor osseointegration. Recent developments in the surface modifications of titanium implants have demonstrated osteoinduction in vitro and in vivo by nanopatterning the surface of these 3D materials.44 Magnesium-based metal implants have strikingly different properties from titanium, as this metal will rapidly resorb by the body and has osteogenic effects similar to degradable biomaterials.43 Magnesium offers structural support (i.e. high mechanical stiffness), but rapidly corrodes in the body which can result in hyper-magnesia and voids in bone formation, and has no method of preventing implant infection and subsequent biofilm formation.43,45 Recent developments in magnesium alloys have combined this material with calcium and zinc to release these ions to the surroundings to enhance angiogenesis and osteogenesis, as well as combining with graphene to impart antimicrobial properties.45,46 Zinc has also been investigated in bone repair due to its biocompatible and antimicrobial properties.47 However, pure zinc has low mechanical properties and as it degrades releases large amounts of zinc ions to the surroundings, which are detrimental to cells.47 Recently, zinc alloys have been investigated, and altering the design of this material to include porosity has improved cell attachment and hydroxyapatite coatings have been added to further improve biocompatibility.47 The use of metals could prove a very promising approach if surface modifications and controlled release of metal ions are further investigated and to provide an osteogenic effect.
Ceramics
Ceramic or hydroxyapatite-based materials are the alternative of choice after autografts and allografts in the clinic.48 Although these are the preferred biomaterial for bone repair due to their biocompatibility and high mechanical properties, these materials are generally brittle and can have lengthy resorption times.49 Bioglass is the most commonly used ceramic for bone repair, containing calcium and phosphorous among other elements, but overall this material is generally less successful than autografts.36,37,50 To improve the mechanical properties of 45S5 bioglass, metal oxides have been doped into this material, as well as nanosilicates such as magnesium silicate, which has demonstrated improved osteogenic differentiation.51 Specifically, 3D bioglass scaffolds with this nanoclay were able to promote osteogenic differentiation of adipose-derived stem cells and cranial bone formation.52 Tricalcium phosphates and calcium phosphate cements have similar drawbacks and advantages as bioglass, with slow resorption, brittle properties, and a biocompatible nature.53 These can also be injectable, and like bioglass, have been doped with similar metals such as zinc and magnesium, and more recently been doped with manganese to improve osteogenesis due to its positive influence and involvement in bone formation.53 A more recent and promising ceramic material are mesoporous silicate nanoparticles, which have demonstrated high mechanical properties, osteogenic behavior, and have been used as drug carriers due to their porous nature.54 Most often these nanoparticles are combined with other materials to elute growth factors, but recently Kanniyappan et al. investigated the impact of various concentrations of pure mesoporous silicate nanoparticles on osteogenesis.55 Of note, high concentrations of these nanoparticles demonstrated settling and reduced viability of cells, however, at concentrations of 1 mg mL−1 these were osteogenic and promoted angiogenesis.55 Ceramic materials could prove very promising in combination with metals or other materials to impart improved strength and osteogenesis.
Polymers
Polymers used for tissue regeneration should be biodegradable and biocompatible, with special consideration of degradation byproducts for cytotoxic effects. Polymers offer advantages in large scale reproducibility and unique control over mechanical properties, degradation, and structure by manipulating polymer chains.56 Drawbacks to these include poor mechanical properties compared to bone and the possibility of host rejection and fibrous tissue formation due to released byproducts. Two of the most commonly used polymers are FDA approved polycaprolactone (PCL) and poly(lactic acid) (PLA), which can degrade in the body via hydrolysis, but their degradation byproducts are acidic, and in high enough quantities may damage cells.39,57,58 Both polymers are biodegradable and biocompatible, but PLA offers high mechanical strength and shorter degradation times, while PCL offers flexibility and hydrophobicity.59 Due to these disadvantages, PLA and PCL have been combined to create polymer blends to leverage the best qualities of both polymers to optimize degradation time and improve mechanical properties and flexibility of the resulting material.59 To improve the osteogenic response of PLA alone, hydroxyapatite coatings have been used to alleviate acidic byproduct release and increase bioactivity.60 PCL has also been investigated as a shape-memory polymer to improve fit of the implant with host bone defects, however, a high transition temperature was needed for shape actuation.61 Recent developments by the Grunlan Lab have further modified the PCL polymer with star architectures in order to lower this transition temperature and increase expansion pressure to fit against host bone.62 These types of polymers offer biocompatibility and easy structure modification by 3D-printing technologies and polymer composition allowing for a large realm of possibilities to tailor these materials for bone repair.
Other polymers derived from animals and insects, such as collagen and chitosan, have been used extensively to heal both hard and soft tissues. Collagen is the main organic constituent of bone and thus using collagen materials has found great success in bone and wound regeneration. Porous type I collagen scaffolds combined with glycosaminoglycans have been successfully used to repair tendon and skin, and the addition of calcium phosphate to these has resulted in bone repair.63–71 A benefit to using collagen scaffolds are their tunable pore size and orientation, their ability as high growth factor-retention sponges, and ease of incorporating additional materials during fabrication such as adding zinc particles.63,66,67,72–76 A drawback to these materials are their extremely mechanically weak nature, which are far from matching the mechanical properties of bone, and most collagen used in biomaterial applications is animal derived and there are concerns of disease transmission, specifically, bovine spongiform encephalopathy (BSE) and transmissible spongiform encephalopathy (TSE).77 To overcome these limitations, 3D-printed polymers have been incorporated into mineralized collagen scaffolds to create composite materials with moduli similar to the 3D-print material used, and salmon-derived collagen has been investigated as an alternative to bovine collagen to avoid religious concerns and disease transmission.78–80 Hydrogels have also been investigated as methods to repair bone due to their injectable nature and ability to release drugs to the surroundings. Hydrogel materials such as chitosan or alginate typically have low cell infiltration and vessel formation throughout due to slow degradation.81 Chitosan offers antibacterial and anti-inflammatory properties but hydrogels made of this have similarly weak mechanics to collagen and low cell attachment and osteoconductivity.82 Additional mineral can be added to chitosan hydrogels, similar to collagen scaffolds, to induce osteogenic responses, and furthermore, creation of granular hydrogels can enhance porosity and cell infiltration.81,82 Promising new approaches to improving hydrogels include incorporation of synthetic polymers and extracellular-derived matrices which include glycosaminoglycans and proteins beneficial for tissue repair. Recently, a pig-bone ECM was combined with polyethylene glycol diacrylate to lengthen degradation of the hydrogel and promote osteogenic proliferation.83 Natural polymer-based materials are biocompatible and with the addition of calcium phosphate mineral, can readily promote osteogenesis, and have a promising future when combined with other materials to increase mechanics and stability of these structures.
Composites
Metals, ceramics, and polymers all have their associated benefits and drawbacks for repairing bone defects, and thus recent biomaterial developments have focused on composite materials. This refers to the combination of two or more distinct materials to leverage the benefits of both materials, in the hopes of overcoming the separate material drawbacks. Many of the recent improvements made to metals, ceramics, and polymers have involved a combination of two or more of these materials together. Another example includes combination of ceramic microspheres in a chitosan matrix. Ceramic microsphere granules have been used to reduce the invasiveness of calcium phosphate ceramics but the porosity of these is very low due to the ability of these to aggregate.84 To create a more cohesive and porous material, chitosan and polyethylene glycol were combined with these ceramic microspheres to create a better injectable and mechanically stable implant.84 For example, while chitosan alone is anti-inflammatory it has low mechanical stiffness and calcium–phosphate ceramics are brittle with low porosity, its combination with chitosan can yield a composite with benefits of both to create a more stable material able to regenerate greater host bone with minor inflammation. Many novel materials developed currently, include hydroxyapatite coatings47,60 and metal or ceramics particles45,46,53–55,75 incorporated into polymeric base materials to increase mechanical stability and osteogenesis.79,82,85,86,89 Other unique promising approaches include bone-mimicking structural elements as well as composition, such as the use of Voronoi open-cell architectures to replicate the porosity and mechanical structure of cortical and cancellous bone,87 and 3D-printing haversian canals to better transport multiple cells and nutrients throughout the entire implant.88 Composites represent a new way to use existing materials to improve mechanics and biological performance, as well as avoid many of the drawbacks of these materials. Composite materials are likely to be most successful in the clinic in the future, and new developments using these materials will combine metals, ceramics, and polymers.
Strategies to address the challenges of repairing craniomaxillofacial defects
The low success rates of biomaterial solutions to repair CMF defects can be attributed the challenges associated with generalized wound healing and challenges that are specific to these types of defects. By addressing each of the challenges of CMF defects by biomaterial design and composition, this can improve the outcome of healing in the clinic, but failure to address even one factor may result in catastrophic failure of the implant. General properties of a biomaterial to address the challenges of CMF defect repair are outlined in Fig. 3.
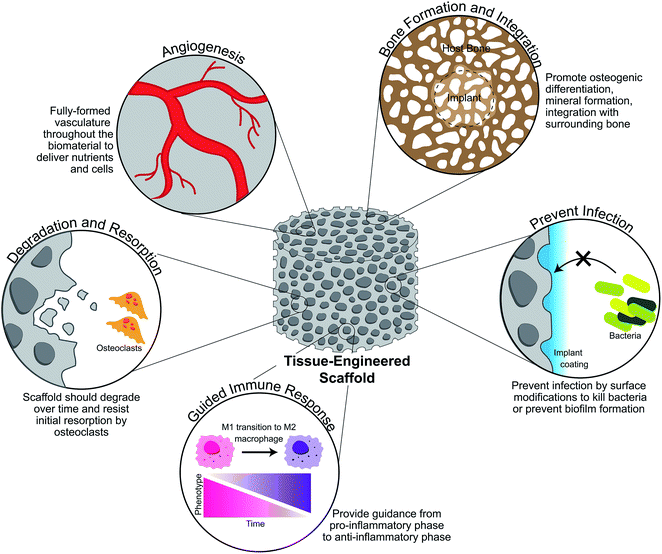 |
| Fig. 3 Ideal properties of a tissue-engineered scaffold for craniomaxillofacial defect repair. A scaffold should promote new and organized vasculature throughout the defect space in order to delivery nutrients and cells to the newly forming bone. It should also be designed to produce new bone and integrate well with the surrounding bone, doing so by degrading over time and resisting initial resorption by osteoclasts. Finally, a scaffold should prevent infection as chances of this are high in CMF defects, while also guiding the immune response to repair rather than persistent inflammation. | |
Biomaterial mechanics
The first step to the biomaterial implantation and bone regeneration process begins with the surgical handling and physical placement of the implant. As simple as this may sound, CMF defects are often irregular in size and shape, especially in the case of birth defects and battlefield injuries. To overcome this obstacle, many researchers have focused on using 3D-printing to create unique and patient-specific implants by scanning the skull with MRI or CT and converting the missing space from the scan into a 3D-print.90 While this makes for enough material to fit the defect space, additional consideration of the surgical handling of the implant is important. Ultimately, if a surgeon has difficulty handling the implant or placing it into the defect space, this will have downstream clinical use and application issues. This can be a problem with extremely stiff implants, which not only must be fabricated extremely precisely to fit within the defect, but also impart unfavorable mechanics to the tissue. Generally, stiffness has been attributed to increases in bone regeneration and many researchers have strived to create implants that can compare to the mechanical properties of bone. However, CMF defects represent an interesting challenge as they are non-load bearing and may not require implants that exactly match their natural properties.
The Young's modulus of cortical and cancellous bone ranges from 15–20 GPa and 0.1–2 GPa, respectively for longer bones, and the compressive modulus of sections of bone from the skull containing both of these regions is on the order of 0.36–5.6 GPa depending on direction of load.6,7 This high mechanical strength, even for cancellous bone, can be difficult to achieve with materials such as polymers, especially as these materials are needed to be porous to allow for cell penetration. Metals and ceramics may more easily approach these mechanics, but it is possible that such a high stiffness is not necessarily needed for bone repair as increases in moduli from 0.34 kPa to 3.9 kPa in crosslinked and non-crosslinked mineralized collagen scaffolds was enough to induce an increase in osteogenic differentiation.91 A factor of possible greater concern is the fit of the implant to the defect space. If an implant is too stiff, most commonly in metal materials, stress-shielding at the bone and metal contact can cause greater bone loss.92 Even will softer materials, if an implant is not mechanically stable and limited in motion, this can cause further damage. Outside the range of 28–150 μm of motion possible between the implant and host bone space can be defined as micromotion, which is undesirable.92 Micromotion can lead to fibrous tissue formation and growth surrounding the implant, ultimately limiting bone regeneration.92 To overcome this problem, many groups have focused on ‘shape-fitting’ implants, created from polymers which can be shaped into the defect space by a surgeon and based on temperature changes can set within the defect space.61,93 These types of materials avoid the issue of micromotion due to hardening within the defect space, but thermo-responsive properties may be limited to synthetic polymers and may not be applicable to metal and ceramic materials. An alternative method to apply shape-fitting properties outside of material composition is through structural modification, which may be applicable to a wider variety of material types. An example of this is using a design able to be conformally contracted by the user, and such a design has been implemented with PLA and used to create tight contact in cylindrical defects smaller than the design itself.79 Other labs and companies such as Dimension Inx (Chicago, IL, USA) have focused on the fabrication of biomaterials from sheets or ones that are not pre-cut to the patient's defect shape, allowing for the surgeon to cut and shape the biomaterial to their liking and fast processing of these materials by avoiding timely patient-tailoring of shape.94,95
In creating an implant that can be formed or manipulated by the surgeon, one can limit the possibility of micromotion that can occur through stiff materials. This not only improves handling, which is desired for clinical applications, but can improve healing as well. Future biomaterial developments for CMF defects in particular should focus on the mechanics of implants, not from the standpoint of matching the stiffness of bone, but to avoid any defect motion and creating materials that can be easily added to the defect space. By doing so, this first obstacle in repair and implantation can be overcome.
Bacterial infection
Bacteria are everywhere and the consequences of their presence in surgical implantation of biomaterials can be devastating. Sterilization of tools, surfaces, skin, and the implant itself are common first precautions to avoid their contamination of the wound, with antibiotics being administered during and after surgery to eliminate any bacteria that may have still been able to enter the wound. Even more concerning, these bacteria that enter the wound site may be antibiotic-resistant, such as the most common bacteria present in bone infections, Staphylococcus aureus, and its antibiotic-resistant strain, Methicillin-resistant Staphylococcus aureus (MRSA).96 Additionally, the chance of infection increases to as high as 50% with type III open wound surgeries or fixations, such as CMF defects, making these likely to become infected even with sterilization of equipment and antibiotic treatments.97,98 Treatment of infections is further complicated by the inability of many antibiotics to penetrate inflamed tissue, and if bacteria are left untreated, this can then cause chronic inflammation and implant failure.22,99 In particular, Staphylococcus aureus (S. aureus) acts to inhibit bone formation by invading osteoblasts and osteocytes and becoming internalized within these cells, protecting it from antibiotics and immune system clearance.97 Once inside osteoblasts, it can inhibit their ability to differentiate and cause apoptosis, which downstream prevents mineral deposition and new bone formation.97 Through this impact on osteoblasts, S. aureus favors osteoclastogenesis and bone resorption, due to an imbalance of osteoclasts and osteoblasts and thus leading to even less bone formation occurring.97 Other bacteria, such as Pseudomonas aeruginosa, will aggregate and form biofilms around an implant, protecting itself from the immune response and antibiotics by the formation of a resistant and protective film.100 Overall, if unable to be cleared by the body's own immune response and antibiotics, bacteria can infiltrate the implanted material and create abscesses and completely inhibit bone formation, leading to another surgery to remove this infected material and clean the wound site.97
To prevent bacterial infection current research has progressed towards developing antibiotic-free methods to eliminate the growing number of antibiotic-resistant bacteria. This can be explored through modifying the topography or composition of biomaterials. The topography of biomaterials can be modified by altering the micron- or nano-scale surface features during fabrication. To illicit bactericidal effects, nano-scale topographies are able to disrupt the bacterial membrane, while micron-scale features can be too large in some cases to have this same effect.101 Beyond scale, the pattern of the topography can affect the way bacteria adhere to a surface as well. Lines, pillars, hexagons and other patterns can inhibit biofilm formation, while pillars and needle-like patterns can kill bacteria on contact while keeping cells alive.101 Pillars and rod-like patterns disrupt bacterial membranes due to their small size and closer-spaced pillars can damage membranes better due to shear forces.102 Fabrication of nano-structured surfaces may be easiest to achieve with polymer and lithography approaches, thus, for materials that may have more difficulties with precise surface modifications, chemical and biological compositional changes may be preferred.
Additives or coatings on biomaterials offer alternatives to antibiotics for reducing bacterial adhesion or promoting bacterial death, and include antimicrobial peptides and enzymes, hydrophobic coatings, nanoparticles, natural materials, among other solutions.103 Antimicrobial peptides (AMPs) have shown effectiveness against Gram-negative and Gram-positive bacteria as well as viruses, due to their overall positive charge and hydrophobic residues, which disrupt the negatively charged bacterial cell wall.103,104 Novel developments in this field have included titanium implants containing titania nanotubes for on-demand delivery of AMPs in stimuli-responsive “boxes” which open to release AMPs under bacterial infection due to a drop in pH.105 This also includes other materials, such as collagen and chitosan scaffolds, loaded with polymeric microspheres containing peptides to eliminate bacterial growth through sustained release of these peptides.106,107 Enzymes can operate by interfering with bacterial adhesion or killing bacteria by hydrolysis of the cell wall and lysis of the bacteria.103 Mesoporous silica nanoparticles have been used as drug delivery vehicles and have been used to deliver levofloxacin, a drug which converts bacterial enzymes into bacteria-toxic enzymes, in response to heightened acid phosphatase levels which occur in bone infection and resorption.108 One common enzyme used to eliminate S. aureus in particular is lysostaphin, a specific anti-staphylococcal enzyme, which has been loaded into hydrogels for eradication of S. aureus infection and while regenerating bone.109,110 Altering the hydrophobicity of a material can prevent adhesion of bacteria and thus prevent accumulation and biofilm formation, but increasing hydrophobicity also prevents host cell attachment and infiltration of the implant to promote tissue regeneration.103 This method has been used by coating titanium implants with positively charged, hydrophobic silane molecules, which prevented bacterial attachment while demonstrating no cytotoxic impact on human dermal fibroblasts.111 Others have also developed thin PLA films containing magnesium particles to control the rate of degradation of this metal, and these films displayed hydrophobicity and resulting bacteriostatic behavior.112 One of the most common antimicrobial additives are metal particles, and specifically silver nanoparticles, which have been used in the food industry. Alternatively to silver, other metals such as gold, aluminum, copper, iron, magnesium, zinc, bismuth, cerium, and titanium have been also used as nanoparticles to combat bacterial infections.113 Zinc and silver nanoparticles in various ratios have been added to titanium implants for release of these factors over a minimum of 28 days to eliminate adherent and planktonic MRSA.114 Finally, natural additives have been explored recently as coatings or added compositions to biomaterials, such as honeys, chitosan and animal-derived products, algae and other plant by-products.115 Chitosan has been most recently used in combination with antibiotics as a material for controlled release of these to the surroundings, as pure chitosan implants have demonstrated little resistance to bacteria compared to antibiotic controls.116 However, loading these chitosan sponges with antibiotics can increase clearance of S. aureus more so than antibiotic application alone.117 Honey in particular has been of recent focus due to its low pH and hydrogen peroxide content attributing to its antibacterial properties, and has been incorporated into hydrogels and on the surface of materials as honey-needles to kill bacteria.118–120
Infection can occur during implantation of a biomaterial and remain unknown after surgery until it is too late, and the removal of the infected biomaterial is necessary. Additionally, antibiotics do not afford the security of infection prevention they once did, therefore design of implants for CMF defects must include antibacterial properties due to the high chance of infection. Whether incorporation of this be as a topographical or compositional design, there are many avenues to choose from to create antibacterial biomaterials.
Immune response
Another challenge to healing CMF defects is directing the immune response to repair. When a biomaterial is implanted into the body, the body can see this as a foreign substance and recruit macrophages to break it down or wall it off from the rest of the body. This foreign body reaction, if persistent, can result in a fibrous wall surrounding the implant and fibrous tissue blocking bone formation from occurring, thus resulting in implant failure. To avoid this, a large body of research has focused on the response of macrophages to implants. As stated previously, M1 and M2 macrophages transition to heal the wound successfully, but persistence of these and their stimuli can lead to fibrous tissue formation and chronic inflammation, and ultimate failure of healing. By designing materials to interact with the immune response to guide in repair and transition eventually out of an inflammatory reaction, we can create more successful healing outcomes.
There are various properties of a biomaterial that can affect the way macrophages and other immune cells react to its implantation. A few of these that have shown significant effect are the pore size and shape, degradation byproducts, and shape and topography of the implant. Previous work by Sussman et al. has demonstrated that a pore size of 34 μm can influence macrophages towards a pro-inflammatory phenotype, with 63% of macrophages expressing M1 markers and 81% reduction in M2 markers.121 This pore size also led to an increase in myofibroblasts, most likely due to an increase in M1 response, but non-porous materials had thicker fibrotic capsule resulting from a foreign body reaction and less vascularization.121 Studies by Madden et al. implanted porous materials for cardiac regeneration and demonstrated that pore sizes above 45 μm in diameter resulted in organized fibrotic tissue, and they discovered a pore size from 30–40 μm promoted a M2-like response, reducing fibrosis and increasing angiogenesis.122 Not only does the pore size affect macrophage polarization, but shape of pores also impacts this, as work by McWhorter et al. demonstrated that micropatterning a surface to cause macrophage elongation shifts the phenotype towards M2 and enhances M2 cytokine effects.123 Careful consideration must be made on choosing a biomaterial for bone regeneration in the case of degradation byproducts, as many of these can by cytotoxic in high quantities. Generally, particles from wear of implants and degradation by hydrolysis can cause production of pro-inflammatory cytokines, with an example of this are poly(lactic-acid)-based biomaterials, which have been shown to cause an inflammatory response.124,125 This inflammatory response can be attributed to large releases of the degradation byproducts, specifically acidic lactic acid, and small PLA particles (<2 μm) can induce a foreign body response, by persistence of M1 macrophages, and bone resorption.126 Additionally, the large-scale size and shape of the implanted material can illicit an inflammatory response. Thicker materials have been shown to illicit a greater foreign body response and fibrotic tissue, and a greater surface area as well as sharp and angular shapes are more likely to induce a foreign body response and M1 phenotype.127,128 Alternatively, growth factors and other molecules can be added to the surface of materials to facilitate the M1 to M2 transition to prevent chronic inflammation. Some examples of this include coatings that release IL-4 from polypropylene meshes to promote M2 responses,129 early release of IFNγ and then later release of strontium ions to force an early M1 and later M2 phenotype transition in glass composite scaffolds,130 and scaffolds containing bioactive anti-inflammatory nanocapsules which block M1 inflammatory cytokines while promoting M2 phenotypes to improve bone repair.131 Overall, more care must be taken in the surface and whole design of implants, as pore size, shape, degradation and released products, and material thickness can all influence the response of macrophages and if not designed correctly, can elicit a foreign body response and fibrotic capsule surrounding the implant.
Balancing multiple cell types and interactions
After the immune response dwindles, formation of bone can begin with collagen and mineral deposition. However, there are multiple cell types involved in bone regeneration outside of the immune response, and their interactions must be balanced and promoted in a way that allows them to use the implant for repair. Such cells involved in the regeneration process and bone homeostasis are mesenchymal stem cells, osteoblasts, osteocytes, osteoclasts, pericytes, and endothelial cells. By designing an implant to promote these cells to create healthy bone tissue, one can have a more successful outcome.
Many researchers have studied the effect of osteoblasts on biomaterials for bone, and metals, ceramics, and polymer materials have all demonstrated their ability to work well with osteoblasts.132 Without osteoblasts, new bone formation could not occur, but research should also focus on the precursor to these cells: mesenchymal stem cells. MSCs migrate the wound site and depending on the biomaterial characteristics this can determine the fate of these cells, as they can differentiate into many other lineages besides bone. Additionally, osteoblasts should eventually mature to osteocytes and maintain healthy bone once regenerated. Osteoclasts function to maintain homeostasis in fully-formed bone, but careful consideration must be made to not promote the actions of these cell types early on and cause unwanted resorption of the implant. Finally, endothelial cells and pericytes form vasculature throughout the material to deliver nutrients and continue to supply cells to the wound. Promoting angiogenesis and bone formation while limiting bone resorption can be directed by material composition, stiffness, and pore structure and size.
The composition and structure of biomaterial implants should be as closely related to the natural composition of bone as possible, including a combination of type I collagen and hydroxyapatite mineral.133 The mineral and glycosaminoglycan content within a material alone can have dramatic effects on multiple cell fates. Studies using mineralized collagen scaffolds compared to non-mineralized collagen variants have demonstrated significantly more bone formed in rabbit calvarial defects using mineralized scaffolds.134 Not only does mineral within a biomaterial act to facilitate further mineral deposition by osteoblasts, but also limits bone resorption, as calcium ion signaling may improve secretion of OPG by mesenchymal stem cells and limit osteoclastogenesis.133,135 This has been further demonstrated by the ability of mineralized collagen scaffolds to promote greater OPG release by MSCs and less osteoclast resorptive activity than non-mineralized collagen counterparts.136,137 Additionally, glycosaminoglycans are important constituents of healthy bone and specifically glycosaminoglycans chondroitin-6-sulfate and heparin sulfate have been shown to promote mineral formation in mineralized collagen scaffolds.138 Glycosaminoglycans have dramatic effects on other cells and processes such as angiogenesis and inflammation. Studies using chondroitin sulfate have demonstrated an inhibitory effect of this glycosaminoglycan on monocyte migration in vitro and thus a potential anti-angiogenic effect in vivo.139,140 Additionally, chondroitin sulfate and heparin sulfate have been known to have anti-inflammatory effects, and heparin sulfate has also been shown to demonstrate enhanced osteoclastogenesis.141–144
The stiffness and porosity of a substrate can also act to shift mesenchymal stem cell fate and it is well observed that a stiffer material will influence MSCs towards differentiation into osteoblasts.145 Stiffness not only affects mesenchymal stem cell differentiation, but also angiogenesis, with stiffer materials exhibiting greater angiogenesis in vivo, with this attributed to endothelial cells spreading more on stiffer substrates.146 Electrospinning has been used to study the effect of MSC differentiation due to fiber alignment, and stem cells seeded on aligned substrates promoted osteogenic gene expression over randomly oriented structures.147 This has also held true for anisotropic pores in mineralized collagen scaffolds, where alignment caused an increase in osteogenic gene expression and mineralization.138 This alignment may also have beneficial effects in directing vessel network formation through channel-like materials and providing guidance for angiogenesis.148 Pore size and shape can effect multiple cell types, and thus there is some speculation on the best pore size for enhancing osteogenesis due to multiple cell interactions. It is generally thought that for MSC infiltration and differentiation into osteoblasts pores should range from 50–200 μm in diameter. However, some materials on the order of 1 mm pore diameters have demonstrate bone regeneration, but pores smaller than 50 μm fail to produce mineral.145 Additionally, pore sizes on the larger scale are typically better for blood vessel formation, but pore sizes greater than 400 μm have demonstrated no improvement in this.149 One must also consider pore spacing, as blood vessels in normal bone are no more than 300 μm apart to continue to deliver nutrients.149
Additional materials outside of those naturally found in bone can be added to biomaterials to enhance multiple cell types, such as metal particles. As stated previously, metal particles can be beneficial as antimicrobial additives, and some metal particles have even demonstrated improving bone formation. Incorporation of zinc nanoparticles on mineralized collagen scaffolds induced greater MSC osteogenesis and mineral formation, and magnesium ions have demonstrated the ability to induce MSCs to osteoblasts.75,150 A variety of nanoparticles including gold and silver have been shown to enhance angiogenesis, possibly through the modulation of reactive oxygen species.151–153 When testing the ability of biomaterials to regenerate bone, one can make changes in multiple properties, but the behavior of cells important for bone formation, bone resorption, vascularization, and the immune response need to be studied in order to more accurately predict the outcomes in vivo or in clinical trials, as osteoblasts are not the only cell type that instruct healthy bone formation.
Regenerative healing
The final design criteria of a bone regenerative biomaterial is the full regeneration of the defect space. This design decision is based on the material properties, mainly the degradation and resorption of the implant. One main criteria for a regenerative material is that the host bone regenerate in the defect space, so ultimately this leaves out the use of metals, as these are permanent implants and may integrate with surrounding host bone, but will never be replaced by bone. This is not to say that metal nanoparticles cannot be used to achieve bone regeneration, but metal as a high-volume replacement of the missing tissue will not cause regeneration due to the body's inability to break down this material. Beyond metals, careful care must be exercised when choosing ceramics or polymers as the biomaterial main constituent, especially as polymer degradation times can be easily manipulated.
Ideally, if an implant has not been hindered by the many challenges of early healing then bone regeneration will start to occur within the defect space and within the implant. For full regeneration this means that the degradation of the material must match the rate of new bone formation. If these are not balanced then the material to support bone regeneration may degrade before it can provide essential ingredients for bone repair and leave voids in the defect space, or conversely, the material may remain for too long and inhibit host bone formation. This can be avoided by choosing a material with a degradation time that matches new bone formation and even the thickness of the material. The thicker a polymer or other material leads to a lengthier time for cells and hydrolysis to degrade this material. Typically, it is thought that craniomaxillofacial defects with implants will regenerate bone within 3–6 months after biomaterial implantation if healing occurs healthily.6 Polymers can be specifically designed to degrade slower or more quickly by altering the chemistry and composition, as PCL polymers typically can take over 2 years to degrade, PLA can take over 6 months, and PLGA can take less than 6 months.57,154 To overcome this, chemical changes can be made to the polymer to change its response to temperature, hydrolysis, pH, and other factors, which may help it to degrade faster during bone regeneration.155 Factors outside of materials chemistry have been demonstrated to help in degradation and bone formation, specifically mechanical stimuli has been shown to synchronize degradation and bone formation in calcium sulfate cements for long bone repair.156 However, the application of mechanical stimuli to craniofacial bones may be more difficult as they are not usually under load-bearing conditions.
An additional issue with regenerative biomaterials that may lead incomplete bridging of the bone defect space or non-uniform bone formation due to incomplete cell penetration of the implant. This can be controlled once again by scaffold architecture and porosity. Work by the Wagoner Johnson group at the University of Illinois has demonstrated that microporous hydroxyapatite-containing BCP scaffolds had more uniform bone formation than scaffolds without these pores.157 Additionally, they found micro-porosity effected trabecular thickness and the distance between struts in their 3D-printed scaffolds only effected this thickness at the periphery of the scaffold.157 This work as well as work by Wu et al. have demonstrated that 3D-printing can be used to effectively study and optimize the pore size for bone growth within the center of implants.158
Summary of design principles for next-generation implants to improve craniomaxillofacial bone regeneration
There are many challenges associated with CMF defect repair and implants will face multiple obstacles before successful outcomes, outlined in Table 2. Bulk implant mechanical properties can govern surgical handling and ill-fitting implants can lead to a fibrous encapsulation. Increasing the stiffness of implants increases bone formation by osteoblasts as well as endothelial cell spreading which enhances angiogenesis. The porosity and microstructure of implants can be used to inhibit bacterial attachment, as well as promote M1 or M2-like macrophage response and cell penetration throughout the entire implant. However, this porosity can range from very small pores for promoting pro-healing macrophage phenotype, to being large enough to allow for cell penetration throughout the implant by MSCs, endothelial cells, and osteoblasts. Future studies must include the consideration of the impact of multiple cell types on the pore size and structure, as one pore size may be beneficial for osteogenesis but may promote a pro-inflammatory response. Finally, the composition of the implant plays a very important role in its ability to kill bacteria, promote osteogenesis, degrade during bone formation, and elicit a pro-healing immune response. Biomaterial design principles that focus on addressing the challenges at the many stages of healing are likely to have a more successful clinical outcome for CMF defect repair.
Table 2 Biomaterial modification strategies to address the challenges of CMF defect repair
Challenge |
Ideal properties |
Methods to address |
Ref. |
Mechanics |
Surgical handling |
Easy for surgeons to add to defect |
• 3D-printing exact defect shape |
61, 90, 93–95 |
• Shapeable by surgeon (i.e. putty) |
• Trimmable material (i.e. sheet) |
Stiffness |
Should not be stiffer than bone to avoid stress-shielding and not too soft to avoid material collapse |
• Avoid stiff metal materials |
78 and 91 |
• Create composite structures to increase stiffness of soft materials |
• Cross-linking to add stiffness |
Micromotion |
Limit to 28–150 μm of motion or else fibrosis will occur |
• Design implant with shape-fitting properties |
61, 79 and 93 |
![[thin space (1/6-em)]](https://www.rsc.org/images/entities/char_2009.gif) |
Bacterial infection |
Infection |
Killing bacteria or preventing bacterial adhesion to implant surface without antibiotics |
• Nano-scale surface topography kills bacteria (i.e. pillars or unique patterns) |
101–104, 113 and 115 |
• Compositional changes can kill bacteria or prevent attachment: |
• Antimicrobial peptides and enzymes |
• Hydrophobic coatings |
• Metal nanoparticles |
• Natural materials (i.e. honey, chitosan) |
![[thin space (1/6-em)]](https://www.rsc.org/images/entities/char_2009.gif) |
Immune response |
Macrophage phenotype |
M1 to M2 transition over weeks |
• Porous material facilitates healing, >30 μm pore size to promote M2 |
121 and 123 |
• Patterned surfaces or anisotropic pores promote macrophage elongation and M2 phenotype |
Foreign body response (FBR) |
Avoid material causing FBR |
• Degradation byproducts should not be cytotoxic or in high quantities |
124–128 |
• Particles sizes <2 μm can cause FBR and bone resorption |
• Avoid thick, hard to degrade materials |
• Avoid designing materials with points or sharp edges |
![[thin space (1/6-em)]](https://www.rsc.org/images/entities/char_2009.gif) |
Balancing multiple cell types |
Mesenchymal stem cells, osteoblasts, and osteocytes |
Osteogenesis and differentiation to the bone lineage |
• Metal particles such as zinc and magnesium can induce osteogenesis |
75, 134, 138, 145 and 150 |
• Pore sizes > 50 μm can induce osteogenesis |
• Aligned fibers and pores promote bone formation over random orientations |
• Increasing stiffness increases osteogenesis |
• Mineral (Ca, P) promotes MSC differentiation and osteogenesis |
• Glycosaminoglycans (i.e. Chondroitin-6-sulfate, heparin sulfate) induce osteogenesis |
Osteoclasts |
Limit early resorptive activity of implant |
• Calcium enhances OPG production to block osteoclastogenesis |
133 and 136 |
Pericytes and endothelial cells |
Promote angiogenesis and fully formed and functional vasculature |
• Stiffer materials encourage angiogenesis and endothelial cell spreading |
146, 148 and 149 |
• Aligned or channel-like pores can guide vessel formation |
• Larger pores are better at promoting angiogenesis |
![[thin space (1/6-em)]](https://www.rsc.org/images/entities/char_2009.gif) |
Regenerative healing |
Host bone regeneration |
New bone should form throughout the material without voids |
• Micro-scale porosity enhances bone formation throughout implants |
157 and 158 |
• Metals do not allow for new bone formation |
Material degradation |
Material degradation should match host bone regeneration |
• Thinner materials allow for quicker degradation |
6, 155 and 156 |
• Ideally a material should degrade within 3–6 months for CMF defect repair |
• Polymer chemistry can be modified to hasten degradation by pH changes, temperature, and hydrolysis |
• Mechanical stimuli can help to balance degradation and regeneration |
Author contributions
Marley Dewey contributed to the conceptualization, visualization, and writing – original draft of this manuscript. Brendan Harley contributed to supervision and writing – review & editing of this manuscript.
Conflicts of interest
There are no conflicts of interest to declare.
Acknowledgements
The authors would like to acknowledge the Carl R. Woese Institute for Genomic Biology, and the Chemical and Biomolecular Engineering Department located at the University of Illinois at Urbana-Champaign. The authors would also like to members of the Harley Lab (UIUC) for the assistance editing this review. Research reported in this publication supported by the National Institute of Dental and Craniofacial Research of the National Institutes of Health under Award Number R21 DE026582 as well as funding provided by the NSF Graduate Research Fellowship DGE-1144245 (MD). The content is solely the responsibility of the authors and does not necessarily represent the official views of the NIH or NSF.
References
- M. Elsalanty and D. Genecov, Bone Grafts in Craniofacial Surgery, Craniomaxillofac Trauma Reconstr., 2009, 2, 125–134 CrossRef PubMed.
- E. C. Kruijt Spanjer, B. GKP, I. E. M. van Hooijdonk, A. J. W. P. Rosenberg and D. Gawlitta, Taking the endochondral route to craniomaxillofacial bone regeneration: a logical approach?, J Craniomaxillofac Surg., 2017, 45, 1099–1106 CrossRef PubMed.
- P. R. Brown Baer, J. C. Wenke, S. J. Thomas and C. R. Hale, Investigation of severe craniomaxillofacial battle injuries sustained by u.s. Service members: a case series, Craniomaxillofac Trauma Reconstr., 2012, 5(4), 243–252 CrossRef PubMed.
- A. D. P. Bankoff. Biomechanical Characteristics of the Bone. in Human Musculoskeletal Biomechanics, ed. T. Goswami, InTech, 2012 Search PubMed.
- B. Quang, L. Id, V. Nurcombe, S. M. Cool, C. A. V. Blitterswijk and J. D. Boer, et al., The Components of Bone and What They Can Teach Us about Regeneration, Materials, 2018, 11, 1–16 Search PubMed.
- S. Bose, M. Roy and A. Bandyopadhyay, Recent advances in bone tissue engineering scaffolds, Trends Biotechnol., 2012, 30, 546–554 CrossRef CAS PubMed.
- J. H. McElhaney, J. L. Fogle, J. W. Melvin, R. R. Haynes, V. L. Roberts and N. M. Alem, Mechanical properties of cranial bone, J. Biomech., 1970, 3(5), 495–511 CrossRef CAS PubMed.
- H. A. M. Mahinda and O. P. Murty, Variability in thickness of human skull bones and sternum – an autopsy experience, J. Forensic Med. Toxicol., 2009, 26(2), 26–31 Search PubMed.
- J. H. C. Lee, B. Ondruschka, L. Falland-Cheung, M. Scholze, N. Hammer and D. C. Tong, et al., An Investigation on the Correlation between the Mechanical Properties of Human Skull Bone, Its Geometry, Microarchitectural Properties, and Water Content, J. Healthc. Eng., 2019, 2019, 6515797 Search PubMed.
- J. M. Aldrige and J. R. Urbaniak, Avascular necrosis of the femoral head: Role of vascularized bone grafts, Orthop. Clin. N. Am., 2007, 38, 13–22 CrossRef PubMed.
- S. P. Bruder, N. Jaiswal, N. S. Ricalton, J. D. Mosca, K. H. Kraus and S. Kadiyala, Mesenchymal Stem Cells in Osteobiology and Applied Bone Regeneration, Clin. Orthop. Relat. Res., 1996, 355S, S247–S56 CrossRef PubMed.
- X. Wang, Y. Wang, W. Gou, Q. Lu, J. Peng and S. Lu, Role of mesenchymal stem cells in bone regeneration and fracture repair: a review, Int Orthop., 2013, 37(12), 2491–2498 CrossRef PubMed.
- E. M. Aarden, P. J. Nijweide and E. H. Burger, Function of osteocytes in bone, J. Cell. Biochem., 1994, 55(3), 287–299 CrossRef CAS PubMed.
- G. Bergers and S. Song, The role of pericytes in blood-vessel formation and maintenance, Neuro-Oncology, 2005, 7(4), 452–464 CrossRef CAS PubMed.
- T. Tian, T. Zhang, Y. Lin and X. Cai, Vascularization in Craniofacial Bone Tissue Engineering, J. Dent. Res., 2018, 97, 969–976 CrossRef CAS PubMed.
- S. L. Teitelbaum, Osteoclasts: What Do they Do and How Do They Do It?, Am. J. Pathol., 2007, 170(2), 427–435 CrossRef CAS PubMed.
- M. Che, R. D. Bonfil, R. Fridman, X. Deng, Z. Dong and J. C. Trindade Filho, et al., Matrix Metalloproteinase Activity and Osteoclasts in Experimental Prostate Cancer Bone Metastasis Tissue, Am. J. Pathol., 2011, 166, 1173–1186 Search PubMed.
- C. K. Scott and J. A. Hightower, The matrix of endochondral bone differs from the matrix of intramembranous bone, Calcif. Tissue Int., 1991, 49, 349–354 CrossRef CAS PubMed.
- E. Thompson, A. Matsiko, E. Farrell, D. J. Kelly and F. O’Brien, Recapitulating endochondral ossification: a promising route to in vivo bone regeneration, J. Tissue Eng. Regener. Med., 2015, 9, 889–902 CrossRef CAS PubMed.
- D. Gawlitta, E. Farrell, J. Malda, L. B. Creemers, J. Alblas and W. J. A. Dhert, Modulating Endochondral Ossification of Multipotent Stromal Cells for Bone Regeneration, Tissue Eng., Part B, 2010, 16(4), 385–395 CrossRef PubMed.
- T. A. Wilgus, S. Roy and J. C. McDaniel, Neutrophils and Wound Repair: Positive Actions and Negative Reactions, Adv. Wound Care, 2013, 2(7), 379–388 CrossRef PubMed.
- M. V. Thomas and D. A. Puleo, Infection, inflammation, and bone regeneration: a paradoxical relationship, J. Dent. Res., 2011, 90(9), 1052–1061 CrossRef CAS PubMed.
- B. N. Brown, B. D. Ratner, S. B. Goodman, S. Amar and S. F. Badylak, Macrophage polarization: an opportunity for improved outcomes in biomaterials and regenerative medicine, Biomaterials, 2012, 33, 3792–3802 CrossRef CAS PubMed.
- K. L. Spiller, D. O. Freytes and G. Vunjak-Novakovic, Macrophages Modulate Engineered Human Tissues for Enhanced Vascularization and Healing, Ann. Biomed. Eng., 2015, 43, 616–627 CrossRef PubMed.
- K. L. Spiller, S. Nassiri, C. E. Witherel, R. R. Anfang, J. Ng and K. R. Nakazawa, et al., Sequential delivery of immunomodulatory cytokines to facilitate the M1-to-M2 transition of macrophages and enhance vascularization of bone scaffolds, Biomaterials, 2015, 37, 194–207 CrossRef CAS PubMed.
- Y. K. Kim, E. Y. Chen and W. F. Liu, Biomolecular strategies to modulate the macrophage response to implanted materials, J. Mater. Chem. B, 2016, 4, 1600–1609 RSC.
- E. Gibon, L. Y. Lu, K. Nathan and S. B. Goodman, Inflammation, ageing, and bone regeneration, J. Orthop. Translat., 2017, 10, 28–35 CrossRef PubMed.
- C. M. Runyan and K. S. Gabrick, Biology of bone formation, fracture healing, and distraction osteogenesis, Journal of Craniofacial Surgery, 2017, 28, 1380–1389 CrossRef PubMed.
- R. A. Hortensius and B. A. C. Harley, Naturally derived biomaterials for addressing inflammation in tissue regeneration, Exp. Biol. Med., 2016, 241, 1015–1024 CrossRef CAS PubMed.
- M. Pogrel, S. Podlesh, J. Anthony and J. Alexander, A comparison of vascularized and nonvascularized bone grafts for reconstruction of mandibular continuity defects, J Oral Maxillofac. Surg., 1997, 55, 1200–1206 CrossRef CAS.
- A. Depeyre, S. Touzet-Roumazeille, L. Lauwers, G. Raoul and J. Ferri, Retrospective evaluation of 211 patients with maxillofacial reconstruction using parietal bone graft for implants insertion, J Craniomaxillofac Surg., 2016, 44, 1162–1169 CrossRef PubMed.
- S. Ghanaati, M. Barbeck, P. Booms, J. Lorenz, C. J. Kirkpatrick and R. A. Sader, Potential lack of “standardized” processing techniques for production of allogeneic and xenogeneic bone blocks for application in humans, Acta Biomater., 2014, 10, 3557–3562 CrossRef CAS PubMed.
- H. Bae, L. Zhao, L. Kanim, P. Wong, R. Delamarter and E. Dawson, Intervariability and Intravariability of Bone Morphogenetic Proteins in Commercially Available Demineralized Bone Matrix Products, Spine, 2006, 31, 1299–1306 CrossRef PubMed.
- B. Abuzayed, S. Aydin, S. Aydin, B. Kucukyuruk and G. Sanus, Cranioplasty: review of materials and techniques, J. Neurosci. Rural. Pract., 2011, 2, 162 CrossRef PubMed.
- K. Nelson, T. Fretwurst, A. Stricker, T. Steinberg, M. Wein and A. Spanou, Comparison of four different allogeneic bone grafts for alveolar ridge reconstruction: a preliminary histologic and biochemical analysis, Oral Surg. Oral Med. Oral Pathol. Oral Radiol., 2014, 118, 424–431 CrossRef PubMed.
- A. M. Tatara, G. L. Koons, E. Watson, T. C. Piepergerdes and S. R. Shah, Biomaterials-aided mandibular reconstruction using in vivo bioreactors, Proc. Natl. Acad. Sci. U. S. A., 2019, 116, 6954–6963 CrossRef CAS PubMed.
- N. Broggini, D. D. Bosshardt, S. S. Jensen, M. M. Bornstein, C.-c Wang and D. Buser. Bone healing around nanocrystalline hydroxyapatite, deproteinized bovine bone mineral , biphasic calcium phosphate , and autogenous bone in mandibular bone defects, 2014, pp. 1478–1487 Search PubMed.
- W. Wang and K. W. K. Yeung, Bone grafts and biomaterials substitutes for bone defect repair: a review, Bioact. Mater., 2017, 2, 224–247 CrossRef PubMed.
- T. Gredes, F. Kunath, T. Gedrange and C. Kunert-Keil, Bone Regeneration after Treatment with Covering Materials Composed of Flax Fibers and Biodegradable Plastics: A Histological Study in Rats, BioMed Res. Int., 2016, 2016, 1–8 CrossRef PubMed.
- S. Radzi, G. Cowin and B. Schmutz, Metal artifacts from titanium and steel screws in CT, 1.5T and 3T MR images of the tibial Pilon: a quantitative assessment in 3D, Quant. Imaging. Med. Surg., 2014, 4, 163–172 Search PubMed.
- T. Terjesen, A. Nordby and V. Arnulf, Bone atrophy after plate fixation: compute tomography of femoral shaft fractures, Acta Orthop. Scand., 1985, 56, 416–418 CrossRef CAS PubMed.
- T. E. Crist, P. J. Mathew, E. L. Plotsker, A. C. Sevilla and S. R. Thaller, Biomaterials in Craniomaxillofacial Reconstruction: Past, Present, and Future, J. Craniofac. Surg., 2021, 32(2), 535–540 CrossRef PubMed.
- K. Alvarez and H. Nakajima, Metallic scaffolds for bone regeneration, Materials, 2009, 2, 790–832 CrossRef CAS.
- A. I. M. Greer, V. Goriainov, J. Kanczler, C. R. M. Black, L.-A. Turner and R. M. D. Meek, et al., Nanopatterned Titanium Implants Accelerate Bone Formation In Vivo, ACS Appl. Mater. Interfaces, 2020, 12(30), 33541–33549 CrossRef CAS PubMed.
- N. Safari, N. Golafshan, M. Kharaziha, M. Reza Toroghinejad, L. Utomo and J. Malda, et al., Stable and Antibacterial Magnesium–Graphene Nanocomposite-Based Implants for Bone Repair, ACS Biomater. Sci. Eng., 2020, 6(11), 6253–6262 CrossRef CAS PubMed.
- H. S. Han, I. Jun, H. K. Seok, K. S. Lee, K. Lee and F. Witte, et al., Biodegradable Magnesium Alloys Promote Angio-Osteogenesis to Enhance Bone Repair, Adv. Sci., 2020, 7(15), 1–12 Search PubMed.
- Y. Zhuang, Q. Liu, G. Jia, H. Li, G. Yuan and H. Yu, A Biomimetic Zinc Alloy Scaffold Coated with Brushite for Enhanced Cranial Bone Regeneration, ACS Biomater. Sci. Eng., 2021, 7(3), 893–903 CrossRef CAS.
- M. Bohner, Physical and chemical aspects of calcium phosphates used in spinal surgery, Eur. Spine J., 2001, 10, S114–S21 CrossRef PubMed.
- S. Scaglione, R. Quarto and P. Giannoni, Stem cells and tissue scaffolds for bone repair, Cell. Response Biomater., 2008, 291–312 Search PubMed.
- V. Athanasiou, D. Papachristou, A. Panagopoulos, A. Saridis, C. Scopa and P. Megas, Histological comparison of autograft, allograft-DBM, xenograft, and synthetic grafts in a trabecular bone defect: an experimental study in rabbits, Med. Sci. Monit., 2010, 16, BR24–31 Search PubMed.
- A. Hoppe, N. S. Güldal and A. R. Boccaccini, A review of the biological response to ionic dissolution products from bioactive glasses and glass–ceramics, Biomaterials, 2011, 32(11), 2757–2774 CrossRef CAS PubMed.
- X. Zheng, X. Zhang, Y. Wang, Y. Liu, Y. Pan and Y. Li, et al., Hypoxia-mimicking 3D bioglass-nanoclay scaffolds promote endogenous bone regeneration, Bioact. Mater., 2021, 6(10), 3485–3495 CrossRef CAS PubMed.
- T. Wu, H. Shi, Y. Liang, T. Lu, Z. Lin and J. Ye, Improving osteogenesis of calcium phosphate bone cement by incorporating with manganese doped β-tricalcium phosphate, Mater. Sci. Eng., C, 2020, 109, 110481 CrossRef CAS.
- R. Eivazzadeh-Keihan, K. K. Chenab, R. Taheri-Ledari, J. Mosafer, S. M. Hashemi and A. Mokhtarzadeh, et al.,
Recent advances in the application of mesoporous silica-based nanomaterials for bone tissue engineering, Mater. Sci. Eng., C, 2020, 107, 110267 CrossRef CAS.
- H. Kanniyappan, M. Venkatesan, J. Panji, M. Ramasamy and V. Muthuvijayan, Evaluating the inherent osteogenic and angiogenic potential of mesoporous silica nanoparticles to augment vascularized bone tissue formation, Microporous Mesoporous Mater., 2021, 311, 110687 CrossRef CAS.
- X. Liu and P. Ma, Polymeric Scaffolds for Bone Tissue Engineering, Ann. Biomed. Eng., 2004, 32, 477–486 CrossRef PubMed.
- K. Athanasiou, G. Niederauer and C. M. Agrawal, Sterilization, toxicity, biocompatibility and clinical applications of polylactic acid/polyglycolic acid copolymers, Biomaterials, 1996, 17, 93–102 CrossRef CAS PubMed.
- K. Athanasiou, C. Agrawal, F. Barber and S. Burkhart, Orthopaedic applications for PLA-PGA biodegradable polymers, Arthroscopy, 1998, 14, 726–737 CrossRef CAS.
- Q. Yao, J. G. L. Cosme, T. Xu, J. M. Miszuk, P. H. S. Picciani and H. Fong, et al., Three dimensional electrospun PCL/PLA blend nanofibrous scaffolds with significantly improved stem cells osteogenic differentiation and cranial bone formation, Biomaterials, 2017, 115, 115–127 CrossRef CAS.
- B. Zhang, L. Wang, P. Song, X. Pei, H. Sun and L. Wu, et al., 3D printed bone tissue regenerative PLA/HA scaffolds with comprehensive performance optimizations, Mater. Des., 2021, 201, 109490 CrossRef CAS.
- D. Zhang, O. J. George, K. M. Petersen, A. C. Jimenez-Vergara, M. S. Hahn and M. A. Grunlan, A bioactive “self-fitting” shape memory polymer scaffold with potential to treat cranio-maxillo facial bone defects, Acta Biomater., 2014, 10, 4597–4605 CrossRef CAS PubMed.
- M. R. Pfau, K. G. McKinzey, A. A. Roth, L. M. Graul, D. J. Maitland and M. A. Grunlan, Shape memory polymer (SMP) scaffolds with improved self-fitting properties, J. Mater. Chem. B, 2021, 9, 3826–3837 RSC.
- S. Caliari, W. Grier, D. Weisgerber, Z. Mahmassani, M. Boppart and B. Harley, Collagen scaffolds incorporating coincident gradations of instructive structural and biochemical cues for osteotendinous junction engineering, Adv. Healthcare Mater., 2015, 4, 831–837 CrossRef CAS PubMed.
- A. Gaspar, L. Moldovan, D. Constantin, A. M. Stanciuc, P. M. Sarbu Boeti and I. C. Efrimescu, Collagen-based scaffolds for skin tissue engineering, J Med Life., 2011, 4(2), 172–177 CAS.
- A. Getgood, S. Kew, R. Brooks, H. Aberman, T. Simon and A. Lynn, et al., Evaluation of early-stage osteochondral defect repair using a biphasic scaffold based on a collagen–glycosaminoglycan biopolymer in a caprine model, The Knee, 2012, 19, 422–430 CrossRef PubMed.
- S. R. Caliari and B. A. C. Harley, Structural and biochemical modification of a collagen scaffold to selectively enhance MSC tenogenic, chondrogenic, and osteogenic differentiation, Adv. Healthcare Mater., 2014, 3, 1086–1096 CrossRef CAS PubMed.
- C. M. Murphy and F. J. O'Brien, Understanding the effect of mean pore size on cell activity in collagen-glycosaminoglycan scaffolds, Cell Adhes. Migr., 2010, 4, 377–381 CrossRef PubMed.
- R. A. Hortensius and B. A. C. Harley, The use of bioinspired alterations in the glycosaminoglycan content of collagen-GAG scaffolds to regulate cell activity, Biomaterials, 2013, 34, 7645–7652 CrossRef CAS PubMed.
- B. P. Kanungo, E. Silva, K. V. Vliet and L. J. Gibson, Characterization of mineralized collagen-glycosaminoglycan scaffolds for bone regeneration, Acta Biomater., 2008, 4, 490–503 CrossRef CAS PubMed.
- B. A. Harley, A. K. Lynn, Z. Wissner-Gross, W. Bonfield, I. V. Yannas and L. J. Gibson, Design of a multiphase osteochondral scaffold. II. Fabrication of a mineralized collagen–glycosaminoglycan scaffold, J. Biomed. Mater. Res., Part A, 2010, 92, 1066–1077 Search PubMed.
- A. Al-Munajjed, J. Gleeson and F. O'Brien, Development of a collagen calcium-phosphate scaffold as a novel bone graft substitute, Stud. Health Technol. Inform., 2008, 133, 11–20 Search PubMed.
- F. J. O'Brien, B. A. Harley, I. V. Yannas and L. J. Gibson, The effect of pore size on cell adhesion in collagen-GAG scaffolds, Biomaterials, 2005, 26, 433–441 CrossRef.
- F. J. O'Brien, B. A. Harley, I. V. Yannas and L. Gibson, Influence of freezing rate on pore structure in freeze-dried collagen-GAG scaffolds, Biomaterials, 2004, 25, 1077–1086 CrossRef.
- W. K. Grier, H. Sun, R. A. Chang, M. D. Ramsey and B. A. C. Harley, The influence of cyclic tensile strain on multi-compartment collagen-GAG scaffolds for tendon-bone junction repair, Connect. Tissue Res., 2019, 60, 530–543 CrossRef CAS PubMed.
- A. S. Tiffany, D. L. Gray, T. J. Woods, K. Subedi and B. A. C. Harley, The inclusion of zinc into mineralized collagen scaffolds for craniofacial bone repair applications, Acta Biomater., 2019, 93, 86–96 CrossRef CAS PubMed.
- A. S. Tiffany, M. J. Dewey and B. A. C. Harley, Sequential sequestrations increase the incorporation and retention of multiple growth factors in mineralized collagen scaffolds, RSC Adv., 2020, 10(45), 26982–26996 RSC.
- E. Song, S. Yeon Kim, T. Chun, H.-J. Byun and Y. M. Lee, Collagen scaffolds derived from a marine source and their biocompatibility, Biomaterials, 2006, 27(15), 2951–2961 CrossRef CAS PubMed.
- D. W. Weisgerber, K. Erning, C. L. Flanagan, S. J. Hollister and B. A. C. Harley, Evaluation of multi-scale mineralized collagen–polycaprolactone composites for bone tissue engineering, J. Mech. Behav. Biomed. Mater., 2016, 61, 318–327 CrossRef CAS PubMed.
- M. J. Dewey, E. M. Johnson, D. W. Weisgerber, M. B. Wheeler and B. A. C. Harley, Shape-fitting collagen–PLA composite promotes osteogenic differentiation of porcine adipose stem cells, J. Mech. Behav. Biomed. Mater., 2019, 95, 21–33 CrossRef CAS PubMed.
- B. Hoyer, A. Bernhardt, S. Heinemann, I. Stachel, M. Meyer and M. Gelinsky, Biomimetically mineralized salmon collagen scaffolds for application in bone tissue engineering, Biomacromolecules, 2012, 13, 1059–1066 CrossRef CAS PubMed.
- T. H. Qazi and J. A. Burdick, Granular hydrogels for endogenous tissue repair, Biomaterials and Biosystems, 2021, 1, 100008 CrossRef.
- X. Zhang, Y. He, P. Huang, G. Jiang, M. Zhang and F. Yu, et al., A novel mineralized high strength hydrogel for enhancing cell adhesion and promoting skull bone regeneration in situ, Composites, Part B, 2020, 197, 108183 CrossRef CAS.
- F. Obregon-Miano, A. Fathi, C. Rathsam, I. Sandoval, F. Deheghani and A. Spahr, Injectable porcine bone demineralized and digested extracellular matrix—PEGDA hydrogel blend for bone regeneration, J. Mater. Sci.: Mater. Med., 2020, 31(2), 21 CrossRef CAS PubMed.
- D. B. Lima, M. A. A. de Souza, G. G. de Lima, E. P. Ferreira Souto, H. M. L. Oliveira and M. V. L. Fook, et al., Injectable bone substitute based on chitosan with polyethylene glycol polymeric solution and biphasic calcium phosphate microspheres, Carbohydr. Polym., 2020, 245, 116575 CrossRef CAS PubMed.
- M. J. Dewey, A. V. Nosatov, K. Subedi, R. Shah, A. Jakus and B. A. C. Harley, Inclusion of a 3D-printed Hyperelastic Bone mesh improves mechanical and osteogenic performance of a mineralized collagen scaffold, Acta Biomater., 2021, 121, 224–236 CrossRef CAS.
- D. Weisgerber, D. Milner, H. Lopez-Lake, M. Rubessa, S. Lotti and K. Polkoff, et al., A mineralized collagen-polycaprolactone composite promotes healing of a porcine mandibular ramus defect, Tissue Eng., Part A, 2017, 0, 1–12 Search PubMed.
- S. Gomez, M. D. Vlad, J. Lopez and E. Fernandez, Design and properties of 3D scaffolds for bone tissue engineering, Acta Biomater., 2016, 42, 341–350 CrossRef CAS PubMed.
- M. Zhang, R. Lin, X. Wang, J. Xue, C. Deng and C. Feng, et al., 3D printing of Haversian bone–mimicking scaffolds for multicellular delivery in bone regeneration, Sci. Adv., 2020, 6(12), eaaz6725 CrossRef CAS PubMed.
- J. A. Killion, S. Kehoe, L. M. Geever, D. M. Devine, E. Sheehan and D. Boyd, et al., Hydrogel/bioactive glass composites for bone regeneration applications: Synthesis and characterisation, Mater. Sci. Eng., C, 2013, 33(7), 4203–4212 CrossRef CAS PubMed.
- A. Haleem and M. Javaid, Role of CT and MRI in the design and development of orthopaedic model using additive manufacturing, J. Clin. Orthop. Trauma., 2018, 9(3), 213–217 CrossRef PubMed.
- Q. Zhou, S. Lyu, A. Bertrand, A. Hu, C. Chan and X. Ren, et al., Stiffness of Nanoparticulate Mineralized Collagen Scaffolds Triggers Osteogenesis via Mechanotransduction and Canonical Wnt Signaling, Macromol Biosci., 2021, 21(3), e2000370 CrossRef PubMed.
- M. J. Cross, G. J. Roger and J. Spycher, Cementless fixation techniques and challenges in joint replacement. in Joint Replacement Technology, ed. P. A. Revell, Woodhead Publishing Limited, Cambridge, UK 2 edn, 2014,pp. 186–211 Search PubMed.
- L. N. Nail, D. Zhang, J. L. Reinhard and M. A. Grunlan, Fabrication of a Bioactive, PCL-based “Self-fitting” Shape Memory Polymer Scaffold, J. Visualized Exp., 2015, 104, e52981 Search PubMed.
- Yu-H. Huang, A. E. Jakus, S. W. Jordan, Z. Dumanian, K. Parker and L. Zhao, et al., Three-Dimensionally Printed Hyperelastic Bone Scaffolds Accelerate Bone Regeneration in Critical-Size Calvarial Bone Defects, Plast. Reconstr. Surg., 2019, 1397–1407 CrossRef CAS PubMed.
- A. E. Jakus, A. L. Rutz, S. W. Jordan, A. Kannan, S. M. Mitchell and C. Yun, et al., Hyperelastic “bone”: a highly versatile, growth factor–free, osteoregenerative, scalable, and surgically friendly biomaterial, Sci. Transl. Med., 2016, 8(358), 358ra127 CrossRef PubMed.
- R. Prabhoo, R. Chaddha, R. Iyer, A. Mehra, J. Ahdal and R. Jain, Overview of methicillin resistant Staphylococcus aureus mediated bone and joint infections in India, Orthop. Rev., 2019, 11(2), 8070 Search PubMed.
- J. Josse, F. Velard and S. Gangloff, Staphylococcus aureus vs. Osteoblast: Relationship and Consequences in Osteomyelitis, Front. Cell. Infect. Microbiol., 2015, 5(85), 1–17 Search PubMed.
- A. Trampuz and W. Zimmerli, Diagnosis and treatment of implant-associated septic arthritis and osteomyelitis, Curr. Infect. Dis. Rep., 2008, 10, 394–403 CrossRef.
- F. L. Luthje, S. A. Blirup-Plum, N. S. Moller, P. M. H. Heegaard, H. E. Jensen and K. Kirketerp-Moller, et al., The host response to bacterial bone infection involves a local upregulation of several acute phase proteins, Immunobiology, 2020, 225(3), 1–10 CrossRef PubMed.
- T. Bjarnsholt, The role of bacterial biofilms in chronic infections, APMIS, Suppl., 2013,(136), 1–51 Search PubMed.
- S. W. Lee, K. S. Phillips, H. Gu, M. Kazemzadeh-Narbat and D. Ren, How microbes read the map: Effects of implant topography on bacterial adhesion and biofilm formation, Biomaterials, 2021, 268, 120595 CrossRef CAS.
- M. N. Dickson, E. I. Liang, L. A. Rodriguez, N. Vollereaux and A. F. Yee, Nanopatterned polymer surfaces with bactericidal properties, Biointerphases, 2015, 10(2), 021010 CrossRef PubMed.
- J. J. Swartjes, P. K. Sharma, T. G. van Kooten, H. C. van der Mei, M. Mahmoudi and H. J. Busscher, et al., Current Developments in Antimicrobial Surface Coatings for Biomedical Applications, Curr. Med. Chem., 2015, 22(18), 2116–2129 CrossRef CAS PubMed.
- R. E. Hancock and H. G. Sahl, Antimicrobial and host-defense peptides as new anti-infective
therapeutic strategies, Nat. Biotechnol., 2006, 24(12), 1551–1557 CrossRef CAS PubMed.
- J. Chen, X. Shi, Y. Zhu, Y. Chen, M. Gao and H. Gao, et al., On-demand storage and release of antimicrobial peptides using Pandora's box-like nanotubes gated with a bacterial infection-responsive polymer, Theranostics, 2020, 10(1), 109–122 CrossRef CAS PubMed.
- Y. He, Y. Jin, X. Ying, Q. Wu, S. Yao and Y. Li, et al., Development of an antimicrobial peptide-loaded mineralized collagen bone scaffold for infective bone defect repair, Regener. Biomater., 2020, 7(5), 515–525 CrossRef CAS PubMed.
- Y. Li, R. Na, X. Wang, H. Liu, L. Zhao and X. Sun, et al., Fabrication of Antimicrobial Peptide-Loaded PLGA/Chitosan Composite Microspheres for Long-Acting Bacterial Resistance, Molecules, 2017, 22(10), 1637 CrossRef PubMed.
- L. Polo, N. Gómez-Cerezo, A. García-Fernández, E. Aznar, J. L. Vivancos and D. Arcos, et al., Mesoporous Bioactive Glasses Equipped with Stimuli-Responsive Molecular Gates for Controlled Delivery of Levofloxacin against Bacteria, Chem.–Eur. J., 2018, 24(71), 18944–18951 CrossRef CAS PubMed.
- C. T. Johnson, M. C. P. Sok, K. E. Martin, P. P. Kalelkar, J. D. Caplin and E. A. Botchwey, et al., Lysostaphin and BMP-2 co-delivery reduces S. aureus infection and regenerates critical-sized segmental bone defects, Sci. Adv., 2019, 5(5), eaaw1228 CrossRef CAS PubMed.
- J. K. Kumar, Lysostaphin: an antistaphylococcal agent, Appl. Microbiol. Biotechnol., 2008, 80(4), 555–561 CrossRef CAS PubMed.
- J. Shen, P. Gao, S. Han, R. Y. T. Kao, S. Wu and X. Liu, et al., A tailored positively-charged hydrophobic surface reduces the risk of implant associated infections, Acta Biomater., 2020, 114, 421–430 CrossRef CAS PubMed.
- V. Luque-Agudo, D. Romero-Guzmán, M. Fernández-Grajera, M. L. González-Martín and A. M. Gallardo-Moreno, Aging of Solvent-Casting PLA-Mg Hydrophobic Films: Impact on Bacterial Adhesion and Viability, Coatings, 2019, 9(12), 814 CrossRef CAS.
- Y. N. Slavin, J. Asnis, U. O. Häfeli and H. Bach, Metal nanoparticles: understanding the mechanisms behind antibacterial activity, J Nanobiotechnology, 2017, 15(1), 65 CrossRef PubMed.
- I. A. J. van Hengel, N. E. Putra, M. W. A. M. Tierolf, M. Minneboo, A. C. Fluit and L. E. Fratila-Apachitei, et al., Biofunctionalization of selective laser melted porous titanium using silver and zinc nanoparticles to prevent infections by antibiotic-resistant bacteria, Acta Biomater., 2020, 107, 325–337 CrossRef CAS PubMed.
- R. Gyawali and S. A. Ibrahim, Natural products as antimicrobial agents, Food Control, 2014, 46, 412–429 CrossRef CAS.
- S. I. Kobata, L. E. M. Teixeira, S. O. A. Fernandes, A. A. G. Faraco, P. V. T. Vidigal and I. Dd Araújo, Prevention of bone infection after open fracture using a chitosan with ciprofloxacin implant in animal model, Acta Cir. Bras., 2020, 35(8), e202000803 CrossRef PubMed.
- L. R. Boles, R. Awais, K. E. Beenken, M. S. Smeltzer, W. O. Haggard and A. J. Jessica, Local Delivery of Amikacin and Vancomycin from Chitosan Sponges Prevent Polymicrobial Implant-Associated Biofilm, Mil. Med., 2018, 183(1), 459–465 CrossRef PubMed.
- G. H. Frydman, D. Olaleye, D. Annamalai, K. Layne, I. Yang and H. M. A. Kaafarani, et al., Manuka honey microneedles for enhanced wound healing and the prevention and/or treatment of Methicillin-resistant Staphylococcus aureus (MRSA) surgical site infection, Sci. Rep., 2020, 10(13229), 1–11 Search PubMed.
- K. R. Hixon, T. Lu, M. N. Carletta, S. H. McBride-Gagyi, B. E. Janowiak and S. A. Sell, A preliminary in vitro evaluation of the bioactive potential of cryogel scaffolds incorporated with Manuka honey for the treatment of chronic bone infections, J. Biomed. Mater. Res., Part B, 2018, 106(5), 1918–1933 CrossRef CAS PubMed.
- R. F. El-Kased, R. I. Amer, D. Attia and M. M. Elmazar, Honey-based hydrogel: in vitro and comparative in vivo evaluation for burn wound healing, Sci. Rep., 2017, 7(1), 9692 CrossRef PubMed , available from: http://europepmc.org/abstract/MED/28851905, https://doi.org/10.1038/s41598-017-08771-8, https://europepmc.org/articles/PMC5575255, https://europepmc.org/articles/PMC5575255?pdf=render.
- E. Sussman, M. Halpin, J. Muster, R. Moon and B. Ratner, Porous Implants Modulate Healing and Induce Shifts in Local Macrophage Polarization in the Foreign Body Reaction, Ann. Biomed. Eng., 2014, 42, 1508–1516 CrossRef PubMed.
- L. R. Madden, D. J. Mortisen, E. M. Sussman, S. K. Dupras, J. A. Fugate and J. L. Cuy, Proangiogenic scaffolds as functional templates for cardiac tissue engineering, Proc. Natl. Acad. Sci. U. S. A., 2010, 107, 15211–15216 CrossRef CAS PubMed.
- W. F. Liu, T. Wang, P. Nguyen, F. Y. McWhorter and T. Chung, Modulation of macrophage phenotype by cell shape, Proc. Natl. Acad. Sci. U. S. A., 2013, 110, 17253–17258 CrossRef PubMed.
- K. S. Stankevich, A. Gudima, V. D. Filimonov, H. Klüter, E. M. Mamontova and S. I. Tverdokhlebov, et al., Surface modification of biomaterials based on high-molecular polylactic acid and their effect on inflammatory reactions of primary human monocyte-derived macrophages: perspective for personalized therapy, Mater. Sci. Eng., C, 2015, 51, 117–126 CrossRef CAS PubMed.
- G. Vallés, P. González-Melendi, J. L. González-Carrasco, L. Saldaña, E. Sánchez-Sabaté and L. Munuera, et al., Differential inflammatory macrophage response to rutile and titanium particles, Biomaterials, 2006, 27(30), 5199–5211 CrossRef PubMed.
- J. Suganuma and H. Alexander, Biological response of intramedullary bone to poly-L-lactic acid, J. Appl. Biomater., 1993, 4(1), 13–27 CrossRef CAS.
- O. Veiseh, J. C. Doloff, M. Ma, A. J. Vegas, H. H. Tam and A. R. Bader, et al., Size- and shape-dependent foreign body immune response to materials implanted in rodents and non-human primates, Nat. Mater., 2015, 14(6), 643–651 CrossRef CAS PubMed.
- N. L. Davison, F. Barrère-de Groot and D. W. Grijpma, Degradation of Biomaterials, in Tissue Engineering, ed. C. A. V. Blitterswijk and J. D. Boer, Academic Press. United States, 2 edn, 2015. pp. 177–215 Search PubMed.
- D. Hachim, S. T. LoPresti, C. C. Yates and B. N. Brown, Shifts in macrophage phenotype at the biomaterial interface via IL-4 eluting coatings are associated with improved implant integration, Biomaterials, 2017, 112, 95–107 CrossRef CAS PubMed.
- M. Luo, F. Zhao, L. Liu, Z. Yang, T. Tian and X. Chen, et al., IFN-γ/SrBG composite scaffolds promote osteogenesis by sequential regulation of macrophages from M1 to M2, J. Mater. Chem. B, 2021, 9(7), 1867–1876 RSC.
- C. Yin, Q. Zhao, W. Li, Z. Zhao, J. Wang and T. Deng, et al., Biomimetic anti-inflammatory nano-capsule serves as a cytokine blocker and M2 polarization inducer for bone tissue repair, Acta Biomater., 2020, 102, 416–426 CrossRef CAS PubMed.
- A. Amini, C. Laurencin and S. Nukavarapu, Bone Tissue Engineering: Recent Advances and Challenges, Crit. Rev. Biomed. Eng., 2012, 40(5), 363–408 CrossRef PubMed.
- X. Wu, K. Walsh, B. L. Hoff and G. Camci-Unal, Mineralization of Biomaterials for Bone Tissue Engineering, Bioengineering, 2020, 7(4), 132 CrossRef CAS PubMed.
- X. Ren, V. Tu, D. Bischoff, D. Weisgerber, M. Lewis and D. Yamaguchi, et al., Nanoparticulate mineralized collagen scaffolds induce in vivo bone regeneration independent of progenitor cell loading or exogenous growth factor stimulation, Biomaterials, 2016, 89, 67–78 CrossRef CAS PubMed.
- J. J. Bergh, Y. Xu and M. C. Farach-Carson, Osteoprotegerin expression and secretion are regulated by calcium influx through the L-type voltage-sensitive calcium channel, Endocrinology, 2004, 145(1), 426–436 CrossRef CAS PubMed.
- X. Ren, Q. Zhou, D. Foulad, A. S. Tiffany, M. J. Dewey and D. Bischoff, et al., Osteoprotegerin reduces osteoclast resorption activity without affecting osteogenesis on nanoparticulate mineralized collagen scaffolds, Sci. Adv., 2019, 5, 1–12 Search PubMed.
- X. Ren, Q. Zhou, D. Foulad, M. J. Dewey, D. Bischoff and T. A. Miller, et al., Nanoparticulate mineralized collagen glycosaminoglycan materials directly and indirectly inhibit osteoclastogenesis and osteoclast activation, J. Tissue Eng. Regener. Med., 2019, 13(5), 823–834 CrossRef CAS PubMed.
- M. J. Dewey, A. V. Nosatov, K. Subedi and B. Harley, Anisotropic mineralized collagen scaffolds accelerate osteogenic response in a glycosaminoglycan-dependent fashion, RSC Adv., 2020, 10(26), 15629–15641 RSC.
- Y. Liu, H. Yang, K. Otaka, H. Takatsuki and A. Sakanishi, Effects of vascular endothelial growth factor (VEGF) and chondroitin sulfate A on human monocytic THP-1 cell migration, Colloids Surf., B, 2005, 43(3), 216–220 CrossRef CAS PubMed.
- C. Lambert, M. Mathy-Hartert, J.-E. Dubuc, E. Montell, J. Vergés and C. Munaut, et al., Characterization of synovial angiogenesis in osteoarthritis patients and its modulation by chondroitin sulfate, Arthritis Res. Ther., 2012, 14(2), R58 CrossRef CAS PubMed.
- M. Vallières and P. Souich, Modulation of inflammation by chondroitin sulfate, Osteoarthritis Cartilage, 2010, 18, 18–23 CrossRef PubMed.
- A. G. García, J. Vergés and E. Montell, Immunomodulatory and anti-inflammatory effects of chondroitin sulphate, J. Cell. Mol. Med., 2009, 13, 1451–1463 CrossRef PubMed.
- A. Irie, M. Takami, H. Kubo, N. Sekino-Suzuki, K. Kasahara and Y. Sanai, Heparin enhances osteoclastic bone resorption by inhibiting osteoprotegerin activity, Bone, 2007, 41(2), 165–174 CrossRef CAS PubMed.
- S. Mousavi, M. Moradi, T. Khorshidahmad and M. Motamedi, Anti-Inflammatory Effects of Heparin and Its Derivatives : A Systematic Review, Adv. Pharmacol. Sci., 2015, 2015, 1–14 Search PubMed.
- J. K. Leach and J. Whitehead, Materials-Directed Differentiation of Mesenchymal Stem Cells for Tissue Engineering and Regeneration, ACS Biomater. Sci. Eng., 2018, 4(4), 1115–1127 CrossRef CAS PubMed.
- T. Yeung, P. C. Georges, L. A. Flanagan, B. Marg, M. Ortiz and M. Funaki, et al., Effects of substrate stiffness on cell morphology, cytoskeletal structure, and adhesion, Cell Motil. Cytoskeleton, 2005, 60(1), 24–34 CrossRef PubMed.
- H. Chen, Y. Qian, Y. Xia, G. Chen, Y. Dai and N. Li, et al., Enhanced Osteogenesis of ADSCs by the Synergistic Effect of Aligned Fibers Containing Collagen I, ACS Appl. Mater. Interfaces, 2016, 8(43), 29289–29297 CrossRef CAS PubMed.
- A. Klaumünzer, H. Leemhuis, K. Schmidt-Bleek, S. Schreivogel, A. Woloszyk and G. Korus, et al., A biomaterial with a channel-like pore architecture induces endochondral healing of bone defects, Nat. Commun., 2018, 9, 4430 CrossRef PubMed.
- A. Marrella, T. Y. Lee, D. H. Lee, S. Karuthedom, D. Syla and A. Chawla, et al., Engineering vascularized and innervated bone biomaterials for improved skeletal tissue regeneration, Mater. Today, 2018, 21(4), 362–376 CrossRef CAS PubMed.
- C. C. Hung, A. Chaya, K. Liu, K. Verdelis and C. Sfeir, The role of magnesium ions in bone regeneration involves the canonical Wnt signaling pathway, Acta Biomater., 2019, 98, 246–255 CrossRef CAS PubMed.
- A. K. Barui, S. K. Nethi, S. Haque, P. Basuthakur and C. R. Patra, Recent Development of Metal Nanoparticles for Angiogenesis Study and Their Therapeutic Applications, ACS Appl. Bio Mater., 2019, 2(12), 5492–5511 CrossRef CAS.
- A. K. González-Palomo, K. Saldaña-Villanueva, J. D. Cortés-García, J. C. Fernández-Macias, K. B. Méndez-Rodríguez and P. Maldonado, Effect of silver nanoparticles (AgNPs) exposure on microRNA expression and global DNA methylation in endothelial cells EA.hy926, Environ. Toxicol. Pharmacol., 2021, 81, 103543 CrossRef PubMed.
- S. K. Nethi, S. Mukherjee, V. Veeriah, A. K. Barui, S. Chatterjee and C. R. Patra, Bioconjugated gold nanoparticles accelerate the growth of new blood vessels through redox signaling, Chem. Commun., 2014, 50(92), 14367–14370 RSC.
- M. A. Woodruff and D. W. Hutmacher, The return of a forgotten polymer – polycaprolactone in the 21st century, Prog. Polym. Sci., 2010, 35, 1217–1256 CrossRef CAS.
- S. Deshayes and A. M. Kasko, Polymeric biomaterials with engineered degradation, J. Polym. Sci., Part A: Polym. Chem., 2013, 51(17), 3531–3566 CrossRef CAS.
- J. Zhang, L. Wang, W. Zhang, M. Zhang and Z.-P. Luo, Synchronization of calcium sulphate cement degradation and new bone formation is improved by external mechanical regulation, J. Orthop. Res., 2015, 33(5), 685–691 CrossRef CAS PubMed.
- L. E. Rustom, T. Boudou, B. W. Nemke, Y. Lu, D. J. Hoelzle and M. D. Markel, et al., Multiscale Porosity Directs Bone Regeneration in Biphasic Calcium Phosphate Scaffolds, ACS Biomater. Sci. Eng., 2017, 3(11), 2768–2778 CrossRef CAS PubMed.
- R. Wu, Y. Li, M. Shen, X. Yang, L. Zhang and X. Ke, et al., Bone tissue regeneration: the role of finely tuned pore architecture of bioactive scaffolds before clinical translation, Bioact. Mater., 2021, 6(5), 1242–1254 CrossRef CAS PubMed.
|
This journal is © The Royal Society of Chemistry 2021 |
Click here to see how this site uses Cookies. View our privacy policy here.