DOI:
10.1039/D1RA02379A
(Paper)
RSC Adv., 2021,
11, 19258-19264
Meteorite-catalyzed intermolecular trans-glycosylation produces nucleosides under proton beam irradiation†
Received
25th March 2021
, Accepted 14th May 2021
First published on 27th May 2021
Abstract
Di-glycosylated adenines act as glycosyl donors in the intermolecular trans-glycosylation of pyrimidine nucleobases under proton beam irradiation conditions. Formamide and chondrite meteorite NWA 1465 increased the yield and the selectivity of the reaction. The glycosyl transfer process was highly regioselective in yielding canonical N1-pyrimidine nucleosides, the natural β-anomers prevailing in the presence of formamide and NWA 1465. These data highlight the possible role of intermolecular trans-glycosylation in the prebiotic formation of purine and pyrimidine nucleosides, avoiding the occurrence of independent synthetic pathways.
Introduction
The formation of the glycosyl bond between a sugar and nucleobase is a critical process in the prebiotic origin of nucleosides,1 since both reagents are thermodynamically stable compounds and no further stabilization occurs upon the linkage, especially in the case of aqueous medium.2 In addition, nucleosides lack of the n–σ* hyper-conjugation effect present in the free sugar.3 Multi-steps prebiotic syntheses of nucleosides have been reported,4 encompassing the construction of the sugar on the nucleobase, as in the case of the formamido-pyrimidine chemistry5–9 or, in alternative, the building of the nucleobase on pre-formed amino-sugar (oxazoline chemistry).10–12 These syntheses yield nucleosides in high regio- and stereoselectivity but suffer from the disadvantages of a large number of reaction steps involving protection and de-protection procedures. As an alternative, the one-step synthesis of nucleosides involves the direct formation of the glycosyl bond between the nucleobase and the sugar from pre-formed substrates,13,14 and generated in situ reagents. Example of this latter procedure is the synthesis of three ribonucleosides (adenosine, uridine and cytidine) and one 2′-deoxy ribonucleoside (thymidine) by proton beam irradiation of formamide and meteorites, mimicking the solar wind conditions.15 The efficacy of formamide in the synthesis of biomolecules is evident in a large panel of physical–chemical conditions fueled by mineral catalysis and different energy sources, as reviewed.16–21 In addition, formamide is the optimal solvent for the thiolysis step in the oxazoline chemistry,12 as well as the effective formylating agent in the formamido-pyrimidine procedure.22 The formation of the glycosyl bond under proton beam irradiation occurred by a radical mechanism,23 the observed β-stereoselectivity being due to the interaction of the sugar with the surface of the mineral.12 In this latter case, di-glycosylated adenines 1 were detected as a mixture of the corresponding pyranose (p) and furanose (f) isomers, having β- and α-configuration at the anomeric position, namely N6-(2-deoxy-D-ribopyranosyl)-2′-deoxyadenosine(1pα/β), and N6-(2-deoxy-D-ribofuranosyl)-2′-deoxyadenosine(1fα/β) (Fig. 1).13,24,25 The phosphorylation of adenosine to corresponding nucleotides, including reactive cyclic adenosine monophosphates,26 was also reported in similar conditions.27 Remarkably, di-glycosylated adenines have been identified as a “remnant” structural motif in damaged DNA sites (e.g. abasic sites), where the 2′-deoxyadenosine residue of one helix links the opposite site of the double helix (Fig. 2).28
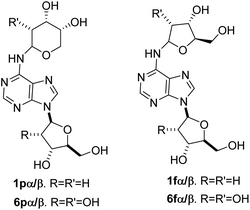 |
| Fig. 1 N6-(2-Deoxy-D-ribopyranosyl)-2′-deoxyadenosine 1pα/β, N6-(2-deoxy-D-ribofuranosyl)-2′-deoxyadenosine 1fα/β, N6-(D-ribopyranosyl)-2′-deoxyadenosine 6pα/β, N6-(D-ribofuranosyl)-2′-deoxyadenosine 6fα/β. | |
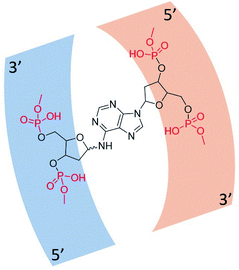 |
| Fig. 2 Structural motif in damaged DNA sites. N6-(2′-deoxy-D-ribofuranosyl)-2′-deoxyadenosine (black) was selected as a representative case of the four possible isomeric forms of the sugar bonded at the N6-exocyclic position of the adenosine residue.29 The sugar-phosphate backbone is reported in a red code. | |
The DNA cross-links are stable at neutral pH and room temperature, but they decompose at relatively high temperature (half-life, 65 days at 37 °C) to yield 2′-deoxyadenosine with release of the N6-glycosyl moiety.29 This implies that di-glycosylated adenines are potential reagents for intermolecular trans-glycosylation processes,30–33 during which the starting nucleoside can perform as the donor of the glycosyl residue when the appropriate nucleobase is provided.34 This process is a valuable entry for the synthesis of a large panel of nucleosides, favoring the easy conversion of purine nucleosides into the pyrimidine counterpart (and vice versa).35
Trans-glycosylation is also relevant in the prebiotic scenario, avoiding independent synthetic for the formation of purine and pyrimidine nucleosides.36 Chemically-driven trans-glycosylation occurs at elevated temperature, or in the presence of heavy metals, usually starting from protected substrates in multi-step conditions.37 Herein, we report the one-pot glycosylation of un-protected pyrimidine nucleobases by the selective intermolecular transfer of the N6-glycosyl moiety from di-glycosylated adenines under proton beam irradiation. The reaction works at low temperature in solid state condition or, in alternative, in formamide and in the presence of the chondrite meteorite Northwest Africa NWA 1465. The complete set of pyrimidine nucleosides in RNA molecules was obtained, the yield and regio- and stereoselectivity of the glycosylation being increased in the presence of formamide and NWA 1465. The reported experimental conditions are similar to those effective during the one-pot synthesis of nucleobases and sugars from a chemical precursor as simple as formamide, furnishing a robust framework for the prebiotic formation of nucleic acid precursors.16
Results and discussion
Di-glycosylated adenines were prepared as previously described.28,29 Briefly, 2′-deoxyadenosine 4 (0.57 mmol) and 2-deoxy-D-ribose (2.62 mmol) were dissolved in glacial acetic acid and methanol (1
:
3 v/v) and the reaction stirred at 40 °C for 72 h to afford 1 in 50% total yield as a mixture of the four corresponding α/β-pyranose and furanose isomers, compounds 1pα, 1pβ, 1fα, and 1fβ, respectively. The relative percentage of the four isomers (Table SI-1†), their synthesis, NMR data and UHPLC-MS analyses are reported in ESI SI #1.† Data were in accordance with those reported in the literature.29
Three types of experiments were performed for the trans-glycosylation of thymine 2 with 1 under proton beam irradiation. One of the experiments was performed in dry-film condition starting from a solid layer mixture of 1 (0.1 mmol) and 2 (0.1 mmol) (condition A).
A second was performed in previous experimental conditions in the presence of formamide (NH2CHO, 1.0 mL) (condition B), while the third experiment was different from the second only for the presence of NWA 1465 (3.6 mg; 10% in weight with respect to 1) (condition C). NWA 1465 is a meteorite representative of the chondrite family38 (preparation of powdered sample, elemental composition and cosmo-origin data of NWA 1465 are in SI #2†) which was previously applied in the synthesis of adenosine derivatives from adenine and sugar in the presence of formamide.13 The samples were irradiated with 170 MeV proton beam for 3.0 min at 243 K (the proton field was bounded to 10 × 10 cm2 by the collimator system). The averaged linear energy transfer (LET), representing the distribution of the energy in the sample with respect to the track of the proton beam, was kept at 0.57 keV μm−1, while the total radiation absorbed dose was 6.0 Gy (Scheme 1). The UHPLC-MS analyses of the reactions are reported in Fig. 3 (panels A–C). The reactions were purified by semi-preparative HPLC and the reaction products characterized by NMR and MS analyses by comparison with data previously reported.39 The yield of reaction products was calculated as percentage of the isolated nucleoside (mmol) with respect to converted 1. 1-(β-D-2′-deoxyribopyranosyl)thymine 3pβ, thymidine 3fβ, 1-(α-D-2′-deoxyribopyranosyl) thymine 3pα, and 1-(α-D-2′-deoxyribofuranosyl) thymine 3fα were detected in low but appreciable amount (the total yield of 3 is reported in Table 1, entry 1), besides to 2′-deoxyadenosine 4, adenine 5 and unreacted 1. The relative percentage of 3pβ, 3fβ, 3pα, and 3fα is reported in Table SI-1.† Adenine 5 was probably formed by partial degradation of adenosine. The mass to charge (m/z) ratio values and relative MS peak abundances of products are in SI #3,† while the original m/z fragmentation spectra are in SI #4.† Compounds 3pβ and thymidine were obtained as the major isomers (Table SI-1†), 3pβ being isolated in the highest yield (the β/α ratio value of isolated anomers is reported in Table 1). The prevalence of the pyranose form is in accordance with data previously reported for the glycosylation of pyrimidine nucleobases with unprotected ribose.40 The possible role of pyranose nucleosides and of other sugar analogues in the prebiotic origin of nucleic acids has been reported and discussed.41 The transfer of the glycosyl moiety from 1 to 2 selectively involved the N6-glycosyl group as a donor and proceeded with high regioselectivity, the N1-glycosylated pyrimidine (thermodynamic product) being the only detected regioisomer. A similar selectivity was reported during the glycosylation of 2 with ribose in the presence of 1,8-diazabicyclo[5.4.0]undec-7-ene (DBU), diisopropyl azodicarboxylate (DIAD), and tri-n-butylphosphine [P(n-Bu)3],41 as a consequence of the higher nucleophilicity of the N1 nitrogen atom in the pyrimidine ring.42 In addition, N-glycosylates are thermodynamically stable products with respect to O-glycosylate counterpart during the trans-glycosylation of pyrimidine nucleobases.43 The glycosyl transfer from 1 probably occurred through the formation of a sugar open-ring imine intermediate at the N6-anomeric position of the donor molecule.29 Note that the regioselectivity was different from that of metal catalyzed conditions, in which case the N3-isomer largely predominate, as a consequence of the formation of the metal σ-complex in the N1-position of the heterocycle ring (e.g. HgX and SnX4 complexes).44–46 Better results were obtained in condition B, in which case 3 was isolated in 61% total yield with a preferred β-stereochemistry (Table 1, entry 2), 3pβ and thymidine being again the major isomers (Table SI-1†). The increase of the yield of 3 was probably due to the high solubilizing effect of formamide.13 Other possible products derived from the formamide condensation pathway15 were not detected in our experimental conditions since they are expected to be synthesized in a negligible amount (μg scale) with respect to that of nucleosides (mg scale). NWA 1465 (condition C) further improved the yield of 3, showing the highest stereoselectivity in the synthesis of the β-anomer (Table 1, entry 3; Table SI-1†).
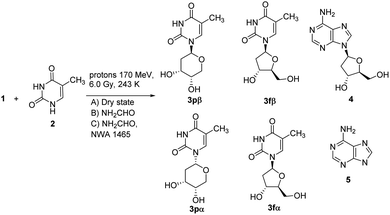 |
| Scheme 1 Synthesis of nucleoside 3 from 1 and thymine 2. | |
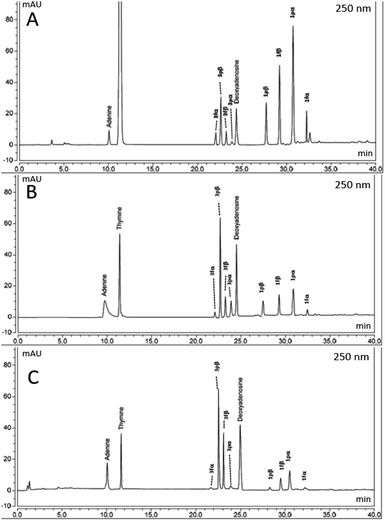 |
| Fig. 3 UHPLC analyses of the reaction between 1 and thymine 2 under different experimental conditions. Panel A: dry-film condition (condition A). Panel B: formamide (condition B). Panel C: formamide + NWA 1465 (condition C). Spectra were recorded at 250 nm. | |
Table 1 Intermolecular trans-glycosylation of nucleobases 2, and 7–8 by di-glycosylated adenines 1 and 6 under proton beam irradiationa
Entry |
Method |
Di-glycosylated adenine |
Nucleobase |
Conv. (%) |
Product(s) |
β/α ratiob |
Yield (%) |
Reactions were performed in the presence of di-glycosylated adenines 1 and 6 (0.1 mmol) and equimolar amount of the appropriate pyrimidine nucleobase 2, 7 and 8. The yield was calculated as percentage (%) of reaction product with respect to converted reagent. The round and square brackets represent the yield of recovered 2′-deoxyadenosine 4 and adenosine 11, and adenine 5, respectively. The data are the mean value of three experiments with standard deviation equal to or less than 0.1%. Ratio between the β and α anomers, including both pyranose and furanose forms, was determined by semi-preparative HPLC purification and comparison with standard compounds. |
1 |
A |
1 |
2 |
25 |
3(4)[5] |
68 : 32 |
18(10)[4] |
2 |
B |
71 |
3(4)[5] |
85 : 15 |
61(31)[10] |
3 |
C |
82 |
3(4)[5] |
98 : 2 |
70(39)[14] |
4 |
A |
6 |
7 |
21 |
9(11)[5] |
70 : 30 |
16 (10)[4] |
5 |
B |
70 |
9(11)[5] |
86 : 14 |
60 (38)[10] |
6 |
C |
78 |
9(11)[5] |
94 : 6 |
65(39)[12] |
7 |
A |
6 |
8 |
14 |
10(11)[5] |
68 : 32 |
10(5)[2] |
8 |
B |
62 |
10(11)[5] |
82 : 18 |
45(25)[11] |
9 |
C |
70 |
10(11)[5] |
93 : 7 |
49(34)[13] |
This reaction pattern is in accordance with the preferential attack of the nucleobase from the less hindered side of the sugar once adsorbed on the meteorite surface.47 Due to the relevance of ribonucleosides in the “RNA world” hypothesis of the Origin of Life,48 we successively evaluated the efficacy of the trans-glycosylation procedure in the case of ribose. N6-(D-ribos-1-yl)-adenosine 6 (Fig. 1) was prepared as a mixture of the four possible isomers by the procedure previously reported for the synthesis of 1. Adenosine 11 (0.57 mmol) and-D-ribose (2.62 mmol) were dissolved in glacial acetic acid and methanol (1
:
3 v/v) and the reaction was stirred at 40 °C for 72 h to afford 6 in 25% total yield. Synthesis, NMR data and UHPLC-MS analysis of 6 are in SI #1.† The chromatographic profile of 6 showed four peaks corresponding to the formation of expected isomers 6pα, 6pβ, 6fα and 6fβ (Table SI-1, SI #1†). The irradiation experiments were repeated in conditions A–C by reaction of 6 with uracil 7 and cytosine 8 as pyrimidine acceptors. The crudes were analyzed by UHPLC-MS analyses (SI #5†) and purified by semi-preparative HPLC to yield pyrimidine nucleosides 9 and 10, respectively, as a mixture of the corresponding pyranose and furanose isomers (Table SI-1†), besides to unreacted 6, adenosine 11 and adenine 5 (Scheme 2). The isolated products were analyzed by NMR and MS, and the structural data were in accordance with authentical samples or with data reported in the literature.49 The results confirmed the high regioselectivity in the formation of N1-pyrimidine isomers, including canonical uridine 9fβ and cytidine 10fβ, from low to acceptable yield (Table 1, entries 4–9). Conditions B–C afforded the highest conversion of substrate and yield of nucleoside (Table 1, entry 4 versus entries 5–6, and entry 7 versus entries 8–9). Irrespective from the experimental conditions, 9pβ and uridine, and 10pβ and cytidine, were isolated as the major isomers (Table SI-1†), the β-pyranose derivatives being isolated in the highest yield. In accordance with the Baker's rule (that is the neighboring group participation of the 2′-OH of ribose in the formation of the glycosyl bond), nucleosides 9 and 10 were largely obtained as the corresponding β-anomers, with the only exception of condition A, in which case an appreciable amount of the α-isomer was also detected (Table 1).
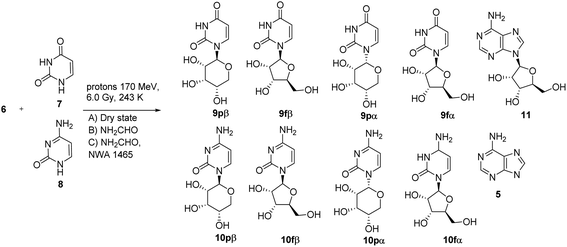 |
| Scheme 2 Synthesis of nucleoside 9–10 from 6 and nucleobase 7–8. | |
Experimental part
General information
All reagents and solvents were purchased from Aldrich and used without further purification. NWA 1465 from Sahara-Naizak was used after previous treatment as described in SI #2.† All reactions were performed under an inert argon atmosphere. Glassware was dried with a flame under a stream of argon gas and allowed to cool under an inert atmosphere prior to use. Flash chromatography was performed on 230–400 mesh silica gel, and thin-layer chromatography was performed on alumina plates coated with silica gel (Merck 60 F254 plates). TLC plates were performed by UV absorbance at 254 nm or staining with bromo cresol. The analysis of the samples was performed by using the Ultimate 3000 Rapid Resolution UHPLC system (DIONEX, Sunnyvale, USA) equipped with C18 REPROSIL-PUR BASIC column (2.5 μm × 150 mm × 2 mm) or, in alternative, a Phenomenex Gemini AXIA PA C18 reversed-phase semi-preparative column (21 mm × 250 mm, 10 μm). Chromatographic separations were achieved using the following conditions: column temperature 30 °C, flow rate 0.2 mL min−1 or 2 mL min−1, gradient elution with phase A (H2O, 0.05% formic acid) and phase B (acetonitrile, CH3CN). The 0–18% linear gradient of phase A to phase B was employed over 50 min, returning to 100% A in 10 min. Products were detected by their absorbance at 250 nm. The UHPLC system was coupled with a mass spectrometer Q-Extractive (Thermo). The instrument was used in positive ionization mode with a full scan program (FS). Nuclear magnetic resonance spectra (1H NMR, 13C NMR) were recorded on Bruker 400 MHz spectrometer.
Synthesis of pyrimidine nucleosides 3, 9 and 10 in dry-film condition (condition A)
Compounds 1 or 6 (0.1 mmol) and the appropriate pyrimidine nucleobase (0.1 mmol) were solubilized in 2.0 mL of water, stirred for 1 min and successive dried under nitrogen and high vacuum until complete solvent evaporation. Thereafter, the mixture was irradiated at 243 K with 170 MeV protons for 3.0 min at the Phasatron accelerator facility of the Joint Institute for Nuclear Research of Dubna (Moscow region, Russia). The uniform proton field was bounded to 10 × 10 cm2 by the collimator system. The averaged linear energy transfer (LET) was 0.57 keV μm−1 and the calculated absorbed dose was 6.0 Gy. Thereafter the sample was analyzed by UHPLC-MS detection without any further purification. To unambiguously assign the structures of nucleoside isomers, the reaction mixtures were purified by semi-preparative HPLC and the isolated products analyzed by 1H–13C-NMR and MS (SI #6†). Structures were assigned by comparison with commercially available samples and data in the literature.49
Synthesis of pyrimidine nucleosides 3, 9 and 10 in formamide and NWA 1465 (conditions B and C)
Compounds 1 or 6 (0.1 mmol) and the appropriate nucleobase (0.1 mmol) were solubilized in 2.0 mL of formamide (in the case of condition C, 10% w/w of NWA 1465 were added in the mixture), stirred for 1.0 min and irradiated as previously reported. In the case of condition C, the samples were filtered at the end of the irradiation to remove NWA 1465. Thereafter the samples were analyzed as described in condition A.
Equipment
Ultimate 3000 Rapid Resolution UHPLC system (DIONEX, Sunnyvale, USA).
Bruker 400 MHz spectrometer (Bruker Billerica, Massachusetts, USA).
Conclusions
The prebiotic synthesis of nucleosides is a relevant process in the bottom-up model for the origin of pristine nucleic acid molecules. Phosphorylation50 and trans-phosphorylation processes51 are available for the successive formation of nucleotides and oligonucleotides, and different mechanisms for the polymerization of these building blocks to larger molecules are reported, encompassing both template and un-template conditions.3,18,26 Two different levels of chemical complexity are operative in the synthesis of nucleosides: (i) the correct regioselectivity in the linkage between the nucleobase and the sugar (that is N1-position for pyrimidines); and (ii) the control of the β-stereochemistry of the glycosyl bond. Multi-steps procedures solved this hurdle by application of scaffold oriented strategies, including the neighboring assistance as in the amino-oxazoline pathway11 or, in alternative, the controlled addition of the 3-OH group of the sugar on the Re-face of the imine intermediate in the formamido pyrimidine chemistry.8 In this latter case, a careful tuning of the pH overcome the regioselectivity problem.8 Irrespective from the synthetic strategy, no-convergent procedures are proposed for the contemporary formation of purine and pyrimidine nucleosides.52 The occurrence of a trans-glycosylation process simplifies this scenario, since the complete set of purine and pyrimidine nucleosides with the correct regio- and stereochemistry can be in principle obtained from only one di-glycosylated derivative, when the appropriate nucleobase is available as glycosyl acceptor. The very fact that di-glycosylated adenine is obtained as a by-product from formamide during the one-pot synthesis of canonical nucleosides and nucleobases, and that they are a “remnant” structural motif in damaged DNA, further suggests that the trans-glycosylation can act as a chemiomimetic process40,53 in expanding the panel of biologically relevant molecules obtainable from a C-1 chemical precursor as simple as formamide. In this chemistry, NWA 1465 shows a relevant effect in the control of the stereochemistry of the transformation, expanding the possible role of meteorites as effective prebiotic chemical factories.54 Meteorites represent a clear-cut case for the role of catalyst complexity in prebiotic process.55 The contemporary presence in meteorites of different minerals and metal oxides in both crystalline and amorphous states furnish a large variety of Lewis acid catalytic sites56 to improve the glycosylation process, in accordance with the role played by organometallic species in this kind of reaction.57 In particular, titanium silico-aluminate, nickel, and iron derivatives present in NWA 1465 are reported as effective Lewis acid transition metal sites in the glycosylation of carbohydrates.58
Conflicts of interest
There are no conflicts to declare.
Acknowledgements
This work is supported by the Italian Space Agency (ASI) BANDO ASI DC-VUM-2017-034 CONTRATTO DI FINANZIAMENTO ASI N. 2019-3-U.0, CUP F86C16000000006 “Vita nello spazio- Origine, presenza, persistenza della vita nello spazio, dalle molecole agli estremofili”.
References
- G. Zubay and T. Mui, Prebiotic Synthesis of Nucleotides, Origins Life Evol. Biospheres, 2001, 31, 87–102 CrossRef CAS PubMed.
- J. E. Šponer, J. Šponer and M. Fuentes-Cabrera, Prebiotic Routes to Nucleosides: A Quantum Chemical Insight into the Energetics of the Multistep Reaction Pathways, Chem.–Eur. J., 2011, 17, 847–854 CrossRef PubMed.
- J. E. Šponer, R. Szabla, R. W. Góra, A. M. Saitta, F. Pietrucci, F. Saija, E. Di Mauro, R. Saladino, M. Ferus, S. Civiš and J. Šponer, Prebiotic Synthesis of Nucleic Acids and Their Building Blocks at the Atomic Level-Merging Models and Mechanisms from Advanced Computations and Experiments, Phys. Chem. Chem. Phys., 2016, 18, 20047–20066 RSC.
- R. Saladino, J. E. Šponer, J. Šponer and E. Di Mauro, Rewarming the Primordial Soup: Revisitations and Rediscoveries in Prebiotic Chemistry, ChemBioChem, 2018, 19, 22–25 CrossRef CAS PubMed.
- F. Micheel and H. Köchling, Über Die Reaktionen Des D-Glucosamins, V. Die Bildung von Glykosiden Des D-Glucosamins Aus Einem Oxazolin-Derivat, Chem. Ber., 1957, 90, 1597–1598 CrossRef CAS.
- S. Konstas, I. Photaki and L. Zervas, Überführung von D-Glucosamin in Oxazolon- Und Oxazolinderivate, Chem. Ber., 1959, 92, 1288–1293 CrossRef CAS.
- R. A. Sanchez and L. E. Orgel, Studies in Prebiotic Synthesis. V. Synthesis and Photoanomerization of Pyrimidine Nucleosides, J. Mol. Biol., 1970, 47, 531–543 CrossRef CAS PubMed.
- S. Becker, I. Thoma, A. Deutsch, T. Gehrke, P. Mayer, Z. Hendrik and T. Carell, A High-Yelding, Strictly Regioselective Prebiotic Purine Nucleoside Formation Pathway, Science, 2016, 352, 833–836 CrossRef CAS PubMed.
- R. Saladino, E. Mincione, C. Crestini, R. Negri, E. Di Mauro and G. Costanzo, Mechanism of Degradation of Purine Nucleosides by Formamide. Implications for Chemical DNA Sequencing Procedures, J. Am. Chem. Soc., 1996, 118, 5615–5619 CrossRef CAS.
- M. W. Powner and J. D. Sutherland, Phosphate-Mediated Interconversion of Ribo- and Arabino-Configured Prebiotic Nucleotide Intermediates, Angew. Chem., Int. Ed., 2010, 49, 4641–4643 CrossRef CAS.
- M. W. Powner, B. Gerland and J. D. Sutherland, Synthesis of Activated Pyrimidine Ribonucleotides in Prebiotically Plausible Conditions, Nature, 2009, 459, 239–242 CrossRef CAS PubMed.
- J. Xu, M. Tsanakopoulou, C. J. Magnani, R. Szabla, J. E. Šponer, J. Šponer, R. W. Góra and J. D. Sutherland, Prebiotically Plausible Synthesis of Pyrimidine β-Ribonucleosides and Their Phosphate Derivatives Involving Photoanomerization, Nat. Chem., 2017, 9, 303–309 CrossRef CAS PubMed.
- R. Saladino, B. M. Bizzarri, L. Botta, J. Šponer, J. E. Šponer, T. Georgelin, M. Jaber, B. Rigaud, M. Kapralov, G. N. Timoshenko, A. Rozanov, E. Krasavin, A. M. Timperio and E. Di Mauro, Proton Irradiation: A Key to the Challenge of N-Glycosidic Bond Formation in a Prebiotic Context, Sci. Rep., 2017, 7, 14709 CrossRef PubMed.
- W. D. Fuller, R. A. Sanchez and L. E. Orgel, Studies in Prebiotic Synthesis. VI. Synthesis of Purine Nucleosides, J. Mol. Biol., 1972, 67, 25–33 CrossRef CAS.
- R. Saladino, E. Carota, G. Botta, M. Kapralov, G. N. Timoshenko, A. Y. Rozanov, E. Krasavin and E. Di Mauro, Meteorite-Catalyzed Syntheses of Nucleosides and of Other Prebiotic Compounds from Formamide under Proton Irradiation, Proc. Natl. Acad. Sci. U. S. A., 2015, 112, E2746–E2755 CrossRef CAS PubMed.
- R. Saladino, G. Botta, S. Pino, G. Costanzo and E. Di Mauro, Genetics First or Metabolism First? The Formamide Clue, Chem. Soc. Rev., 2012, 41, 5526–5565 RSC.
- R. Saladino, C. Crestini, S. Pino, G. Costanzo and E. Di Mauro, Formamide and the Origin of Life, Phys. Life Rev., 2012, 9, 84–104 CrossRef PubMed.
- L. Botta, R. Saladino, B. M. Bizzarri, B. Cobucci-Ponzano, R. Iacono, R. Avino, S. Caliro, A. Carandente, F. Lorenzini, A. Tortora, E. Di Mauro and M. Moracci, Formamide-Based Prebiotic Chemistry in the Phlegrean Fields, Adv. Space Res., 2018, 62, 2372–2379 CrossRef CAS.
- J. E. Šponer, J. Šponer, O. Nováková, V. Brabec, O. Šedo, Z. Zdráhal, G. Costanzo, S. Pino, R. Saladino and E. Di Mauro, Emergence of the First Catalytic Oligonucleotides in a Formamide-Based Origin Scenario, Chem.–Eur. J., 2016, 22, 3572–3586 CrossRef PubMed.
- R. Saladino, C. Crestini, G. Costanzo and E. Dimauro, On the Prebiotic Synthesis of Nucleobases, Nucleotides, Oligonucleotides, Pre-RNA and Pre-DNA Molecules, Top. Curr. Chem., 2005, 259, 29–68 CrossRef CAS.
- B. M. Bizzarri, L. Botta, M. I. Pérez-Valverde, R. Saladino, E. Di Mauro and J. M. García-Ruiz, Silica Metal Oxide Vesicles Catalyze Comprehensive Prebiotic Chemistry, Chem.–Eur. J., 2018, 24, 8126–8132 CrossRef PubMed.
- L. J. Yu, C. J. Wiederholt, J. N. Patro, K. Haraguchi and M. M. Greenberg, Synthesis of Oligonucleotides Containing Fapy·dG (N-6-(2-Deoxy-α,β-D-Erythropentofuranosyl)-2,6-Diamino-4-Hydroxy-5-Formamidopyrimidine) Using a 5′-Dimethoxytrityl Dinucleotide Phosphoramidite, J. Org. Chem., 2005, 70, 141–149 CrossRef PubMed.
- L. Botta, B. M. Bizzarri, D. Piccinino, T. Fornaro, J. Robert Brucato and R. Saladino, Prebiotic Synthesis of Carboxylic Acids, Amino Acids and Nucleic Acid Bases from Formamide under Photochemical Conditions, Eur. Phys. J. Plus, 2017, 132, 317 CrossRef.
- M. Tomasz, R. Lipman, M. S. Lee, G. L. Verdine and K. Nakanishi, Reaction of Acid-Activated Mitomycin C with Calf Thymus DNA and Model Guanines: Elucidation of the Base-Catalyzed Degradation of N7-Alkylguanine Nucleosides, Biochemistry, 1987, 26, 2010–2027 CrossRef CAS PubMed.
- M. Berger and J. Cadet, Isolation and Characterization of the Radiation-Induced Degradation Products of 2′-Deoxyguanosine in Oxygen-Free Aqueous Solutions, Z. Naturforsch. B, 2015, 40, 1519–1531 Search PubMed.
- G. Costanzo, R. Saladino, G. Botta, A. Giorgi, A. Scipioni, S. Pino and E. Di Mauro, Generation of RNA Molecules by a Base-Catalysed Click-Like Reaction, ChemBioChem, 2012, 13, 999–1008 CrossRef CAS PubMed.
- B. M. Bizzarri, J. E. Šponer, J. Šponer, G. Cassone, M. Kapralov, G. N. Timoshenko, E. Krasavin, G. Fanelli, A. M. Timperio, E. Di Mauro and R. Saladino, Meteorite-Assisted Phosphorylation of Adenosine Under Proton Irradiation Conditions, ChemSystemsChem, 2020, 1, e1900039 Search PubMed.
- N. E. Price, K. M. Johnson, J. Wang, M. I. Fekry, Y. Wang and K. S. Gates, Interstrand DNA–DNA Cross-Link Formation between Adenine Residues and Abasic Sites in Duplex DNA, J. Am. Chem. Soc., 2014, 136, 3483–3490 CrossRef CAS PubMed.
- N. E. Price, M. J. Catalano, S. Liu, Y. Wang and K. S. Gates, Chemical and Structural Characterization of Interstrand Cross-Links Formed between Abasic Sites and Adenine Residues in Duplex DNA, Nucleic Acids Res., 2015, 43, 3434–3441 CrossRef CAS PubMed.
- B. Shimizu and M. Miyaki, Studies on Nonspecific Adenosine Deaminase from Takadiastase, J. Biochem., 1966, 59, 265–271 CrossRef PubMed.
- B. Shimizu and M. Miyaki, Transglycosylation from pyrimidines to purines, Tetrahedron Lett., 1968, 9, 855–859 CrossRef.
- B. Shimizu and M. Miyaki, N → N Alkyl and Glycosyl Migration of Purines and Pyrimidines. III. N → N Alkyl and Glycosyl Migration of Purine Derivatives, Chem. Pharm. Bull., 1970, 18, 1446–1456 CrossRef.
- M. Miyaki and B. Shimizu, N → N Alkyl and Glycosyl Migration of Purines and Pyrimidines. III. (1) N → N Alkyl and Glycosyl Migration of Purine Derivatives, Chem. Pharm. Bull., 1970, 18, 1446–1456 CrossRef CAS.
- J. Boryski, Reactions of Transglycosylation in the Nucleoside Chemistry, Curr. Org. Chem., 2008, 12, 309–325 CrossRef CAS.
- J. Boryski, Transglycosylation Reactions of Purine Nucleosides. A Review, Nucleosides and Nucleotides, 1996, 15, 771–791 CrossRef CAS.
- N. Kitadai and S. Maruyama, Origins of Building Blocks of Life: A Review, Geosci. Front., 2018, 9, 1117–1153 CrossRef CAS.
- M. J. McKay and H. M. Nguyen, Recent Advances in Transition Metal-Catalyzed Glycosylation, ACS Catal., 2012, 2, 1563–1595 CrossRef CAS PubMed.
- L. Botta, R. Saladino, B. M. Bizzarri, B. Cobucci-Ponzano, R. Iacono, R. Avino, S. Caliro, A. Carandente, F. Lorenzini, A. Tortora, E. Di Mauro and M. Moracci, Formamide-Based Prebiotic Chemistry in the Phlegrean Fields, Adv. Space Res., 2018, 62, 2372–2379 CrossRef CAS.
-
(a) S. N. Mikhailov and E. V. Efimtseva, Synthesis of enantiomers of 3',4'-seco-2'-desoxythymidine, Chem. Heterocycl. Compd., 1988, 24, 778–782 CrossRef;
(b) Y. M. Ying, W. G. Shan, W. H. Liu and Z. J. Zhan, Alkaloids and Nucleoside Derivatives from a Fungal Endophyte of Huperzia serrata, Chem. Nat. Compd., 2013, 49, 184–186 CrossRef CAS;
(c) R. Huang, X. Zhou, Y. Peng, X. Yang, T. Xu and Y. Liu, Nucleosides from the Marine Sponge Callyspongia SP, Chem. Nat. Compd., 2011, 46, 1010–1011 CrossRef CAS.
- A. M. Downey, C. Richter, R. Pohl, R. Mahrwald and M. Hocek, Direct One-Pot Synthesis of Nucleosides from Unprotected or 5-O-Monoprotected D-Ribose, Org. Lett., 2015, 17, 4604–4607 CrossRef CAS PubMed.
- B. Pullmann, Electronic structure, chemical reactivity, and basicity of purines and pyrazolopyrimidines, J. Chem. Soc., 1959, 1621–1623 Search PubMed.
- A. Eschenmoser and E. Loewenthal, Chemistry of Potentially Prebiological Natural Products, Chem. Soc. Rev., 1992, 21, 1–16 RSC.
- G. T. Rogers, R. S. Shadbolt and T. L. V. Ulbricht, Nucleosides. Part VII. The Reaction of Metal Salts of Thymine and Uracil with Tetra-Acetyl-α-Glucopyranosyl Bromide, J. Chem. Soc. C, 1969, 2, 203–208 RSC.
- H. Vorbrüggen and G. Höfle, Nucleoside Syntheses, XXIII1) On the Mechanism of Nucleoside Synthesis, Chem. Ber., 1981, 114, 1256–1268 CrossRef.
- T. Ukita, H. Hayatsu and Y. Tomita, Reinvestigation of the Condensation Reaction of Acetobromoglucose with Chloromercuri-4-Ethoxy-2(1H)-Pyrimidinone to 1-(Tetra-O-Acetyl-β-D-Glucopyranosyl)-4-Ethoxy-2(1H)-Pyrimidinone, Chem. Pharm. Bull., 1963, 11, 1068–1073 CrossRef CAS PubMed.
- H. Vorbrüggen, U. Niedballa, K. Krolikiewicz, B. Bennua and G. Höfle, in Chemistry and Biology of Nucleosides and Nucleotides, ed. R. Harmon, R. K. Robins and L. B. Towsend, Academic Press, New York, 1978, pp. 251–265 Search PubMed.
- M. Ferus, A. Knížek and S. Civiš, Meteorite-Catalyzed Synthesis of Nucleosides and Other Prebiotic Compounds, Proc. Natl. Acad. Sci. U. S. A., 2015, 112, 7109–7110 CrossRef CAS PubMed.
- H. S. Bernhardt, The RNA World Hypothesis: The Worst Theory of the Early Evolution of Life (except for All the Others)a, Biol. Direct, 2012, 7, 23 CrossRef CAS PubMed.
-
(a) U. Kang, S. M. Ryu, D. Lee and E. K. Seo, Chemical Constituents of the Leaves of Brassica oleracea Var. Acephala, Chem. Nat. Compd., 2018, 54, 1023–1026 CrossRef CAS;
(b) J. Ren, Y. G. Xie, Y. G. Guo, S. K. Yan and H. Z. Jin, Chemical Constituents of Liparis viridiflora, Chem. Nat. Compd., 2019, 55, 552–554 CrossRef CAS;
(c) S. Becker, J. Feldmann, S. Wiedemann, H. Okamura, C. Schneider, K. Iwan, A. Crisp, M. Rossa, T. Amatov and T. Carell, Unified prebiotically plausible synthesis of pyrimidine and purine RNA ribonucleotides, Science, 2019, 366, 76–82 CrossRef CAS.
-
(a) Y. Furukawa, H. J. Kim, D. Hutter and S. A. Benner, Abiotic regioselective phosphorylation of adeno- sine with borate in formamide, Astrobiology, 2015, 15, 1–9 CrossRef;
(b) G. Costanzo, R. Saladino, C. Crestini, F. Ciciriello and E. Di Mauro, Nucleoside Phosphorylation by Phosphate Minerals, J. Biol. Chem., 2007, 282, 16729–16735 CrossRef CAS PubMed;
(c) B. Burcar, M. Pasek, M. Gull, B. J. Cafferty, F. Velasco, N. V. Hud and C. Menor-Salván, Darwin's Warm Little Pond: A One-Pot Reaction for Prebiotic Phosphorylation and the Mobilization of Phosphate from Minerals in a Urea-Based Solvent, Angew. Chem., Int. Ed., 2016, 55, 13249–13253 CrossRef CAS PubMed;
(d) Y. Yamagata, H. Watanabe, M. Saitoh and T. Namba, Volcanic Production of Polyphosphates and Its Relevance to Prebiotic Evolution, Nature, 1991, 352, 516–551 CrossRef CAS PubMed.
-
(a) L. E. Orgel, Prebiotic Chemistry and the Origin of the RNA World, Crit. Rev. Biochem. Mol. Biol., 2004, 39, 99–123 CrossRef CAS PubMed;
(b) J. P. Ferris, Catalysis and Prebiotic RNA Synthesis, Origins Life Evol. Biospheres, 1993, 23, 307–315 CrossRef CAS PubMed.
- A. Biscans, Exploring the Emergence of RNA Nucleosides and Nucleotides on the Early Earth, Life, 2018, 8, 57 CrossRef CAS PubMed.
- J. Peretó, Out of Fuzzy Chemistry: From Prebiotic Chemistry to Metabolic Networks, Chem. Soc. Rev., 2012, 41, 5394–5403 RSC.
- R. Saladino, L. Botta and E. Di Mauro, The Prevailing Catalytic Role of Meteorites in Formamide Prebiotic Processes, Life, 2018, 8, 6 CrossRef PubMed.
- B. M. Bizzarri, P. Manini, V. Lino, M. d'Ischia, M. Kapralov, E. Krasavin, K. Mráziková, J. Šponer, J. E. Šponer, E. Di Mauro and R. Saladino, High-Energy Proton-Beam-Induced Polymerization/Oxygenation of Hydroxynaphthalenes on Meteorites and Nitrogen Transfer from Urea: Modeling Insoluble Organic Matter?, Chem.–Eur. J., 2020, 26, 14919–14928 CrossRef CAS PubMed.
-
(a) E. B. Bauer, Transition metal catalyzed glycosylation reaction- an overview, Org. Biomol. Chem., 2020, 18, 9160–9180 RSC;
(b) B. M. Bizzarri, R. Saladino, I. Delfino, J. M. García-Ruiz and E. Di Mauro, Prebiotic organic chemistry of formamide and the origin of life in planetary conditions: What we know and what is the future, Int. J. Mol. Sci., 2021, 22, 917 CrossRef PubMed.
- J. Boryski, Transglycosylation Reactions of Purine Nucleosides, 1996 Nucleosides. A Review, Nucleosides and Nucleotides, 1996, 15, 771–791 CrossRef CAS.
-
(a) M. Pfaffe and R. Mahrwald, Direct glycosylation of unprotected and unactivated carbohydrates under mild conditions, Org. Lett., 2012, 14, 792–795 CrossRef CAS PubMed;
(b) X. Li and J. Zhu, Recent Advances in Transition Metal–Catalyzed O-Glycosylations, J. Carbohydr. Chem., 2012, 31, 284–324 CrossRef;
(c) G. Zhang, Q. Liu, L. Shi and J. Wang, Ferric sulfate hydrat-catalyzed O-glycosilation using glycals with or without microwave irradiation, Tetrahedron, 2008, 64, 339–344 CrossRef CAS.
Footnote |
† Electronic supplementary information (ESI) available. See DOI: 10.1039/d1ra02379a |
|
This journal is © The Royal Society of Chemistry 2021 |
Click here to see how this site uses Cookies. View our privacy policy here.