DOI:
10.1039/D1RA02309H
(Paper)
RSC Adv., 2021,
11, 31533-31546
Low temperature scalable synthetic approach enabling high bifunctional electrocatalytic performance of NiCo2S4 and CuCo2S4 thiospinels†
Received
23rd March 2021
, Accepted 22nd August 2021
First published on 24th September 2021
Abstract
Ternary metal sulfides are currently in the spotlight as promising electroactive materials for high-performance energy storage and/or conversion technologies. Extensive research on metal sulfides has indicated that, amongst other factors, the electrochemical properties of the materials are strongly influenced by the synthetic protocol employed. Herein, we report the electrochemical performance of uncapped NiCo2S4 and CuCo2S4 ternary systems prepared via solventless thermolysis of the respective metal ethyl xanthate precursors at 200 and 300 °C. The structural, morphological and compositional properties of the synthesized nanoparticles were examined by powder X-ray diffraction (p-XRD), transmission electron microscopy (TEM), high-resolution TEM, scanning electron microscopy (SEM), X-ray photoelectron spectroscopy (XPS) and energy-dispersive X-ray spectroscopy (EDX) techniques. Electrochemical studies indicate that NiCo2S4 nanoparticles synthesized at 300 °C exhibit superior energy storage characteristics with a high specific capacitance of ca. 2650 F g−1 at 1 mV s−1, as compared to CuCo2S4 nanoparticles, which showcased a specific capacitance of ca. 1700 F g−1 at the same scan rate. At a current density of 0.5 A g−1, NiCo2S4 and CuCo2S4 nanoparticles displayed specific capacitances of 1201 and 475 F g−1, respectively. In contrast, CuCo2S4 nanoparticles presented a higher electrocatalytic activity with low overpotentials of 269 mV for oxygen evolution reaction (OER), and 224 mV for the hydrogen evolution reaction (HER), at 10 mA cm−2. The stability of the catalysts was examined for 2000 cycles in which a negligible change in both OER and HER activities was observed.
1. Introduction
Designing energy storage devices and conversion technologies based on clean and renewable energy is critical for sustainable energy development. Supercapacitors have demonstrated high functional capability in terms of power density, lifecycle, and charge–discharge rate and have therefore received great attention as efficient energy storage devices.1 Responding to the projected high energy consumptions, recent studies have mainly focused on improving device energy density and rate capability.2 The hydrogen production via the electrochemical splitting of water is another intensively investigated strategy of renewable energy storage and/or conversion.3 While the approach presents a simple way of producing high purity hydrogen, its practical application is impeded by the large overpotential involved.3,4 To attain faster reaction kinetics and scale-up hydrogen production, the process requires the use of earth-abundant, cost-effective and highly efficient electrocatalysts.5 On this account, numerous electroactive materials have been investigated for both energy storage and energy conversion applications. Transition metal nitrides,6 transition metal dichalcogenides,7 hybrids of metals and metal phosphides/metal nitrides,8 carbon nanomaterials, their doped derivatives, and composites,9 and ternary thiospinels,1b,10 are examples of advanced materials which have demonstrated remarkable performance.
NiCo2S is a normal thiospinel with remarkable electrochemical performance.1b,11 This superior performance is believed to be due to the high conductivity of the material, which is attributed to its metallic character.11 Since the first report by Chen et al.,12 a large number of studies on the use of NiCo2S4 in supercapacitors,13 batteries,14 dye-sensitized solar cells,15 oxygen reduction/evolution reactions,16 and hydrogen generation reactions17 have been carried out. Recently, CuCo2S4, another member of the ternary thiospinels, has been reported for its potential in energy storage/conversion applications.18 Similar to NiCo2S4, CuCo2S4, has a normal thiospinel crystal structure with copper and cobalt ions occupying the tetrahedral and octahedral sites, respectively.19 It is paramagnetic and metallic, with unique electronic properties. For instance, it exhibits a low resistivity (10−4 Ω), and s-wave superconductivity at low temperature.20 Specifically, CuCo2S4 has mainly been studied as an electrode material for lithium-ion batteries,18c sodium-ion batteries,18b and supercapacitors.18e Limited studies on the electrocatalytic activity of CuCo2S4 in oxygen reduction/evolution reactions,18d and hydrogen generation reactions18a are known. Both bulk and nanostructured NiCo2S4/CuCo2S4 based materials have been studied. It has been shown that, nano-engineering of the electrodes/electrocatalysts enhances the electrochemical performance of the materials.1b,21
The synthetic protocol employed in nanomaterials preparation is among the key factors determining the final structure and resultant properties of the materials.1b,22 Years of extensive research on nanoscience and nanotechnology has provided us with numerous synthetic approaches, with solution-based techniques dominating the field.23 A characteristic feature of nanomaterials synthesized in solution is the presence of surface adsorbed ligands which provide particle stabilization via electrostatic or steric repulsive interactions. Long-chain amines, carboxylates, thiols, and phosphines are some of the commonly employed ligands.24 While capping ligands are indispensable during nanoparticles synthesis, studies have shown that the presence of these ligands on the surface of a nanoparticle may, in some cases, act as impurities, blocking the particle's active sites and diminish the material's end application, particularly catalysis. For example, Ung et al.25 studied the effect of various amine and carboxylate ligands on the electrocatalytic activity of CoP towards hydrogen generation in which the uncapped CoP nanocrystals demonstrated higher activity than the capped nanocrystals. Lu et al.26 examined the electrocatalytic activity of Ag nanorods for oxygen reduction reaction in which the surfactant-free nanorods displayed higher activity than the nanorods capped with polyvinylpyrrolidone. In another study, Henckel et al.27 investigated the role of dodecylamine, as a surfactant, on the hydrogen generation activity of WSe2. Removing the ligand from the catalytic sites of the material was observed to improve the kinetics of the reaction. The synthesis of nanomaterials free of capping ligands is therefore crucial for their catalytic performance. Several techniques for the removal of the capping ligands after nanomaterials synthesis have been developed.26–28 However, most of these techniques are laborious, expensive, and sometimes alter the shape and stability of the particles.29
The melt method is a recently described approach of synthesis which allows the preparation of nanomaterials in the absence of any passivating ligands.30 This provides the possibility of synthesizing nanomaterials with abundant active sites for enhanced electrochemical performance. Unlike solution-based techniques, nanomaterials are synthesized via solvent-free, surfactant-free thermolytic decomposition of their corresponding molecular precursors.30,31 The approach is therefore simple, inexpensive and eco-friendly. Herein, we report the synthesis of uncapped NiCo2S4 and CuCo2S4 nanoparticles via the melt approach using respective metal ethyl xanthate precursors. The efficiency of the synthesized ternary systems for supercapacitance and water splitting applications has been examined. To the best of our knowledge, this is the first study investigating the electrochemical properties of the two systems synthesized using the melt approach.
2. Experimental
2.1. Chemicals
Potassium ethyl xanthogente (96%, Sigma-Aldrich), cupric chloride dihydrate (97%, Saarchem), cobalt(II) acetate tetrahydrate (98%, Saarchem); and nickel(II) acetate tetrahydrate, chloroform (min 99%), acetone and hexane, purchased from Merck Chemicals. All chemicals were used as received with no further purification.
2.2. Instrumentation
Elemental analysis of the complexes was performed on a PerkinElmer automated model 2400 series II CHNS/O analyzer. Thermogravimetric analysis was done from 30 to 600 °C at 10 °C min−1 using a PerkinElmer Pyris 6 under N2 gas flow. p-XRD analysis was performed using a Bruker AXS D8 diffractometer equipped with a nickel-filtered Cu Kα radiation (λ = 1.5418 Å) at 40 kV, 40 mA, at room temperature. The diffraction patterns were recorded in the high angle 2θ range of 10–70° at a scan speed of 0.2 s per step and an increment of 0.01314. TEM and HRTEM analyses of the synthesized ternary systems were carried out using a JEOL 1400 TEM and JEOL 2100 HRTEM, respectively. Samples were prepared by placing a drop of the particles dilute solution on Formvar-coated grids (150 mesh) for TEM and holey carbon grids for HRTEM. The samples were allowed to dry completely at room temperature, viewed at accelerating voltages of 120 kV and 200 kV for TEM and HRTEM, respectively. Images were captured digitally using a Mega view III camera; stored and measured using soft imaging systems iTEM software (TEM) and Gatan camera with Gatan software (HRTEM). Analysis of surface morphology of the synthesized particles was carried out using a Zeiss Ultra Plus FEG Scanning Electron Microscope (SEM) equipped with an Oxford detector EDX at 20 kV which uses Aztec software for elemental analysis. Kratos Axis Ultra DLD spectroscopy was used to record the X-ray photoelectron spectroscopy (XPS) and the equipment setup and specification were as previously reported.32 Curve fitting and quantitative analysis were conducted using Vision software. NIST XPS database was used for peak assignment for the high-resolution spectra and all binding energies were calibrated against carbon at 284.9 eV.
2.3. Synthesis of the precursors
2.3.1. Synthesis of bis(O-ethylcarbonodithioato)nickel(II); complex (1). In a typical reaction, nickel acetate tetrahydrate (1.2443 g, 5.0 mmol) was dissolved in distilled water (25.0 mL) followed by its drop-wise addition into the aqueous solution of potassium ethyl xanthogenate (1.603 g, 10.0 mmol). The reaction mixture was stirred for 1 hour, after which the formed precipitates were washed with distilled water, dried under vacuum and recrystallized from chloroform.Elemental analysis for C6H10O2S4Ni: calc. C, 23.91%; H, 3.35%; S, 42.51%. Found: C, 23.81%; H, 3.2%; S, 42.17%.
2.3.2. Synthesis of bis(O-ethylcarbonodithioato)copper(II); complex (2). The synthetic procedure used to prepare complex (1) was followed using copper(II) chloride dihydrate (0.8524 g, 5 mmol) as the source of copper.Elemental analysis for C6H10O2S4Cu: calc. C, 23.57%; H, 3.30%; S, 41.86%. Found: C, 23.1%; H, 3.14%; S, 41.16%.
2.3.3. Synthesis of bis(O-ethylcarbonodithioato)cobalt(II); complex (3). Complex (3) was also prepared using a similar synthetic procedure as Section 2.3.2 above, using cobalt(II) acetate tetrahydrate (1.2454 g, 5 mmol) as the cobalt source.Elemental analysis for C6H10O2S4Co: calc. C, 23.93%; H, 3.35%; S, 42.50%. Found: C, 22.03%; H, 3.33%; S, 41.09%.
2.4. The solventless synthesis of NiCo2S4 and CuCo2S4 nanoparticles
Stoichiometric quantities of the required complexes (complexes (1) and (3) for NiCo2S4; and complexes (2) and (3) for CuCo2S4) were ground together to obtain a homogenized mixture. The mixture was then spread in a ceramic boat and placed at the center of a quartz tube. The sample was heated in the furnace at a specified temperature (200 °C or 300 °C) for one hour under N2 flow and then allowed to cool down to room temperature.
2.5. Electrochemical studies
Electrochemical characterization of the synthesized materials was performed using Gamry Potentiostat using a three-electrode system. A paste consisting of the synthesized sample (80 wt%), acetylene black (10 wt%), and polyvinylidene difluoride (PVDF, 10 wt%) was prepared using N-methyl pyrrolidinone (NMP) as a solvent. This paste was then applied to pre-cleaned and weighted nickel foam. The paste was then dried under a vacuum at 60 °C for 10 hours and used as a working electrode. A graphite rod/platinum wire and saturated calomel electrode (SCE) were used as counter and reference electrodes, respectively. All the experiments for energy storage and electrocatalysis were performed using 3 M and 1 M KOH electrolyte, respectively. Charge storage capacity was measured using cyclic voltammetry (CV) and galvanostatic charge–discharge (CD) at various scan rates and current densities. Electrocatalytic properties of the synthesized electrodes were studied using linear sweep voltammetry (LSV), cyclic voltammetry and chronoamperometry (CA). LSV was performed at a scan rate of 2 mV s−1 for both OER and HER measurements.
3. Results and discussion
3.1. Characterization of the precursors and the nanoparticles
The preparation of complexes (1), (2), and (3) involved a well-known synthetic procedure.33 The thermal stability behavior of the complexes was analyzed by TGA and the results are shown in Fig. S1, ESI.† The analysis indicates that all three complexes decompose in a single step to give respective metal sulfide. Specifically, complex (1) shows initial decomposition at 132 °C and complete decomposition occurs at 228 °C. A weight loss of 72% is observed, and the remaining residual weight (28%) agrees well with that of NiS (29%). Complex (2) decomposes at 210 °C with an onset temperature of 77 °C. This yields a 30% residue, which corresponds to the theoretical value calculated for CuS (31%). On the other hand, complex (3) starts decomposing at 96 °C and complete decomposition occurs at 203 °C. A 27% residual weight which correlates with that of CoS (29%), is produced. Generally, all three complexes show complete decomposition at moderate temperatures, which is a characteristic feature of xanthate complexes. Compared to several other precursors, the low decomposition temperature and formation of volatile by-products (COS and an alkene) make xanthates complexes favourable precursors for the solventless synthesis of metal sulfides.34
The two ternary systems were synthesized via thermolysis of stoichiometric mixtures of the required complexes at 200 and 300 °C. The solventless pyrolysis of a mixture of complexes (1) and (3) at both 200 and 300 °C produced black residues, which upon p-XRD analysis, were identified as phase-pure cubic NiCo2S4 (ICDD #: 00-020-0782) (Fig. 1a). The corresponding diffraction peaks are sharp, which denotes the crystallinity of the materials. The relative broad diffraction peaks observed for NiCo2S4 synthesized at 200 °C are indicative of small-sized particles. The average crystallite sizes were calculated from the Scherrer equation and were found to be 5.3 and 12.8 nm at 200 and 300 °C, respectively. On the other hand, the solventless pyrolysis of a mixture of complexes (2) and (3) at the two temperatures yielded phase-pure cubic CuCo2S4 (ICDD #: 00-042-1450) (Fig. 1b). The diffraction peaks are also sharp in this case, signifying the synthesis of crystalline CuCo2S4. The average crystallite sizes of CuCo2S4 synthesized at 200 and 300 °C were found to be 20.1 and 22.9 nm from the Scherrer equation, respectively.
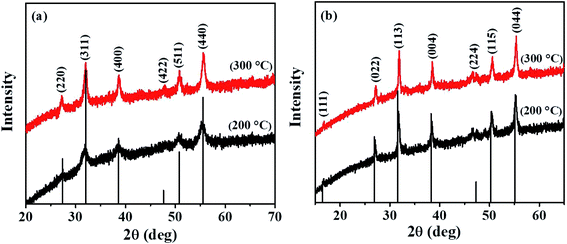 |
| Fig. 1 p-XRD patterns of (a) NiCo2S4 and (b) CuCo2S4 nanoparticles synthesized at 200 °C and 300 °C. | |
The lattice parameters of the synthesized materials were calculated from the XRD data using the relation 1/d2 = (h2 + k2 + l2)/a2. The values were found to be approximately 9.350 Å and 9.348 Å for NiCo2S4 nanoparticles synthesized at 200 and 300 °C, respectively, and 9.443 Å for CuCo2S4 nanoparticles synthesized at 200 and 300 °C, respectively. These values compare well with the theoretical lattice parameters (9.387 Å for NiCo2S4 and 9.474 Å for CuCo2S4).
Morphological properties of the synthesized materials were examined using TEM/HRTEM and SEM analyses. TEM analysis of NiCo2S4 prepared at 200 °C reveals the synthesis of highly agglomerated particles with undefined morphology (Fig. 2a). Carrying out the synthesis at a higher reaction temperature of 300 °C produced particles with a more defined morphology and reduced degree of particle–particle agglomeration. Elongated nanoparticles with approximately uniform sizes were obtained (Fig. 2b). The observed average particle size correlates with that calculated from the Debye–Scherrer equation. Due to the high degree of agglomeration, the average size of the particles synthesized at 200 °C could not be estimated from their TEM image. The agglomeration observed at both 200 and 300 °C is mainly due to the absence of a surface passivating agent. In the absence of surfactants, control over size and shape is challenging. The initially formed nano-sized particles have high surface energy and tend to agglomerate to lower their surface energy. Although melt reactions are sometimes characterized as ‘self-capping’, it has been shown that the control over the size and shape of the nanoparticles is mainly achieved with long alkyl chain precursors. For instance, Zhang et al.33c reported the solventless synthesis of Ag2S nanoparticles via thermal decomposition of silver xanthate precursors of varying alkyl chain lengths. The size of the particles was observed to decrease with increasing the alkyl chain length of the precursor. O'Brien et al.35 employed a similar approach to synthesize PbS nanocrystals from various xanthate precursors in which control over the size and shape of the nanocrystals was achieved by increasing precursor chain length. Therefore, the use of short-chain xanthates is thought to be another reason for the observed agglomeration behavior.
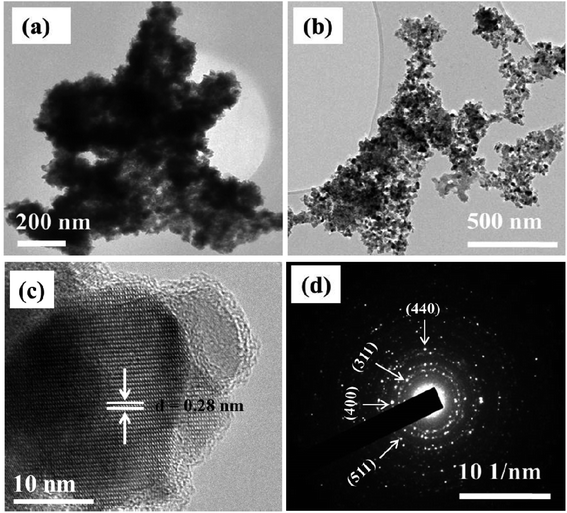 |
| Fig. 2 TEM images of NiCo2S4 nanoparticles synthesized at (a) 200 °C and (b) 300 °C. Corresponding HRTEM (c) and SAED (d) images of the particles synthesized at 300 °C. | |
The HRTEM image of NiCo2S4 nanoparticles synthesized at 300 °C shows unidirectional lattice fringes with a d-spacing of 0.28 nm, which matches that of the (311) plane of the cubic phase NiCo2S4 (Fig. 2c). The corresponding selected area electron diffraction (SAED) image shows bright spots, further detailing the crystalline nature of the material. The diffraction rings can be indexed to the (400), (311), (511) and (440) planes of cubic NiCo2S4 (ICDD #: 00-020-0782) (Fig. 2d).
TEM images of CuCo2S4 nanoparticles synthesized at both 200 °C and 300 °C are shown to exhibit a sheet-like morphology (Fig. 3a and b). As was the case with NiCo2S4 nanoparticles, the tendency of particle–particle agglomeration is also observed in this case. The HRTEM images of the particles synthesized at 300 °C show two types of lattice fringes with a d-spacing of 0.33 and 0.28 nm which matches that of the (022) and (113) planes of cubic CuCo2S4, respectively (Fig. 3c and d). The areas shown by red arrows indicate the presence of slightly distorted lattice planes (Fig. 3c). This can be a result of rapid growth, which is a characteristic feature of the melt method.36 Rapid growth can cause atoms to be displaced from their original positions, inducing various crystal defects.
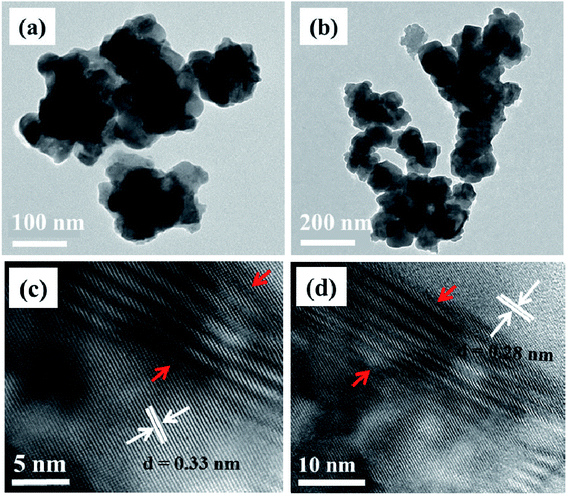 |
| Fig. 3 TEM images of CuCo2S4 nanoparticles synthesized at (a) 200 °C and (b) 300 °C; (c and d) HRTEM images of the particles synthesized at 300 °C. | |
The composition of the synthesized materials was examined by Energy Dispersive X-ray Spectroscopy (EDX). The EDX spectra of NiCo2S4 and CuCo2S4 nanoparticles synthesized at both 200 and 300 °C indicate the presence of only the desired elements (Ni, Co and S for NiCo2S4; and Cu, Co and S for CuCo2S4) (Fig. S2, ESI†). The corresponding quantitative compositions are displayed in Table 1. It can be seen that the obtained experimental compositions, match the expected theoretical compositions with slight deviations (Table 1). Elemental mapping of the samples shows a homogeneous distribution of constituent elements in all the samples (Fig. 4).
Table 1 Atomic percent compositions of NiCo2S4 and CuCo2S4 nanoparticles
Sample |
Pyrolysis temperature (°C) |
Atomic% composition |
Mole ratio |
Ni |
Cu |
Co |
S |
NiCo2S4 |
200 |
17.10 |
— |
28.67 |
54.22 |
1.19 : 2.0 : 3.79 |
NiCo2S4 |
300 |
17.29 |
— |
27.88 |
54.84 |
1.21 : 1.95 : 3.83 |
CuCo2S4 |
200 |
— |
20.05 |
31.11 |
48.84 |
1.4 : 2.17 : 3.4 |
CuCo2S4 |
300 |
— |
20.70 |
28.84 |
50.46 |
1.45 : 2.01 : 3.53 |
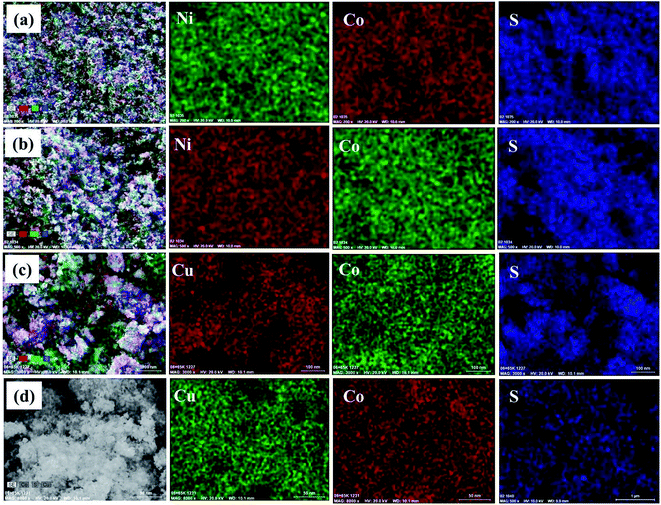 |
| Fig. 4 Elemental mapping of NiCo2S4 nanoparticles synthesized at (a) 200 °C and (b) 300 °C; and CuCo2S4 nanoparticles synthesized at (c) 200 °C and (d) 300 °C. | |
XPS analysis was carried out to study the surface composition and chemical states of the two ternary systems. NiCo2S4 and CuCo2S4 synthesized at 300 °C were used as representative samples. The survey spectra of the two systems are shown in Fig. 5. The presence of Cu 2p peaks at 943 eV for CuCo2S4 and Ni 2p peaks at 870 eV for NiCo2S4 together with Co 2p at 790 eV and S 2p at 164 eV confirms that CuCo2S4 and NiCo2S4 were successfully synthesized. The O 1s (530 eV) and C 1s (285 eV) peaks resulted from the method used in the preparation of the sample and from the metal ethyl xanthate precursors. A slight stoichiometric variation is evident as the elements nickel, cobalt, and sulfur have the tendency to exist in two mineralogical forms, i.e., NiCo2S4 or CoNi2S4. Therefore, with different oxidation states, cobalt can interchangeably occupy tetrahedral or octahedral positions, which may affect the peak intensity and composition.
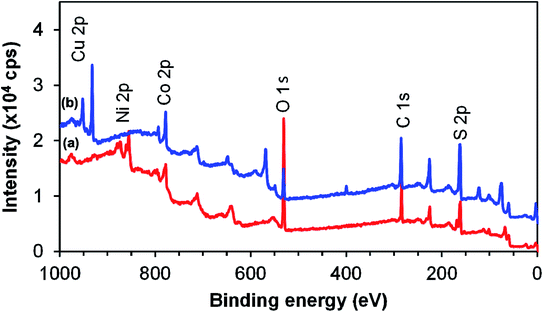 |
| Fig. 5 X-ray photoelectron survey spectra of NiCo2S4 (a) and CuCo2S4 (b) synthesized at 300 °C. | |
Fig. 6 shows high-resolution spectra of Co 2p for (a) CuCo2S4 and (b) NiCo2S4; S 2p for (c) CuCo2S4 and (d) NiCo2S4; Cu 2p for CuCo2S4 (e), and Ni 2p for NiCo2S4 (f). The Co 2p for both samples showed three oxidation states Co3+, Co2+ and Co+, and the NiCo2S4 sample showed the presence of strong satellite peaks at high binding energy. The CuCo2S4 sample exhibited a high concentration of Co3+ species (70.1%) compared with 45.3% of NiCo2S4 sample. Mixed oxidation states of Co were related to the presence of various sulfur oxidation states (S2−, S22−, C–S) as observed in Fig. 6c for CuCo2S4 and (d) for NiCo2S4. The NiCo2S4 sample in Fig. 6d also showed the presence of 10.3% of C–S at 165.1 eV from metal ethyl xanthate precursor compounds and 17.7% sulfate (SO42−) peak at 168.4 eV as a result of high-temperature treatment. Fig. 6e shows Cu 2p with different oxidation states at 932.3 eV for Cu0 and 934.1 and 935.8 eV for Cu2+ oxidation states. The corresponding spin-spit coupling components (ΔE = 19.4 eV) were observed at high binding energies. The Ni 2p peak for NiCo2S4 sample in Fig. 6f also showed mixed oxidation states at different binding energies with 26.4% metallic (Ni0) at 853.2 eV and Ni2+ at 855.9 (51.2%) and 858.5 eV (21.4%).
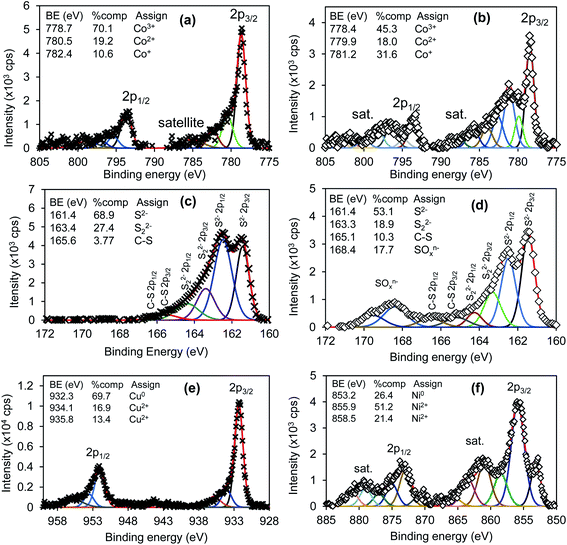 |
| Fig. 6 High-resolution spectra of CuCo2S4 (left column) and NiCo2S4 (right columns) for (a) and (b) Co 2p; (c) and (d) S 2p; (e) Cu 2p and (f) Ni 2p. | |
3.2. Electrochemical studies
Henceforth, the NiCo2S4 nanoparticles synthesized at 200 °C and 300 °C will be referred to as NCS-200 and NCS-300, respectively, whereas CuCo2S4 nanoparticles synthesized at 200 °C and 300 °C will be referred to as CCS-200 and CCS-300 respectively.
3.2.1. Energy storage application. The electrochemical charge storage mechanism of the synthesized NiCo2S4 and CuCo2S4 at different annealing temperatures was first analyzed using CV tests. As observed from the CV curves at 5 mV s−1, all the samples showed a distinct set of oxidation and reduction peaks (Fig. 7a). More specifically, a higher intensity of redox peak indicates improved intercalation of electrolyte ions within the interstitial sites of the thiospinels structure.37 As predicted by this study, the 300 °C annealed samples showed a well-defined structure with minimal nanoparticle agglomeration, allowing a higher exposed surface area for the improved pseudocapacitive performance compared to the 200 °C annealed samples. The CV curves for NCS-300 and CCS-300 electrodes at varying scan rates can be summarized from Fig. 7b and c. As the scan rates were decreased from 300 mV s−1 to 1 mV s−1, pseudocapacitive characteristics of the synthesized electrodes were observed. This could be due to the improved accessibility of the electrolyte ions to facilitate near-surface redox reactions provided by our annealing process. The real-time charge storage performance of NCS-300 and CCS-300 was further analyzed using galvanostatic charge–discharge (CD) test in which a longer charge–discharge time was displayed by NCS-300, indicating higher charge storage activity (Fig. 7d–f).
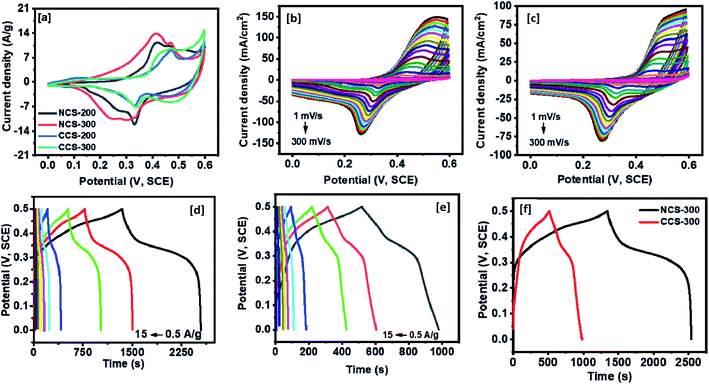 |
| Fig. 7 (a) Comparison data for CV curves at the scan rate of 5 mV s−1 for all the NCS and CCS samples. CV curves at various scan rates for NCS-300 (b) and (c) CCS-300. The potential vs. time plots at various current densities for (d) NCS-300 and (e) CCS-300. (f) CD curves for NCS-300 and CCS-300 at the current density of 0.5 A g−1. | |
The specific capacitance of NCS-300 and CCS-300 as a function of scan rates are shown in Fig. 8a and b. NCS-300 displayed the highest specific capacitance of ca. 2650 F g−1 at 1 mV s−1, whereas CCS-300 achieved a specific capacitance of ca. 1700 F g−1 at the same scan rate. The variation of specific capacitance of the two electrodes as a function of current density is shown in Fig. 8c and d. It can be seen that NCS-300 exhibits the highest specific capacitance of 1201 F g−1 at the applied current density of 0.5 A g−1. This value is much higher compared to several other reported NiCo2S4 electrodes prepared by other methods. For instance, Pu et al. employed a sacrificial template approach based on the Kirkendall effect to synthesize hollow hexagonal NiCo2S4 nanoplates, which delivered a specific capacitance of 437 F g−1 at a current density of 1 A g−1.38 Xu et al. synthesized NiCo2S4 nanoparticles via a one-step solvothermal approach, which displayed a specific capacitance of 519.51 F g−1 at a current density of 2 A g−1.39 Similarly, mesoporous NiCo2S4 nanosheets prepared by Wu et al. via a one-pot hydrothermal method displayed a specific capacitance of 744 F g−1 at a current density of 1 A g−1.40 A further comparison with composites or heterostructures of NiCo2S4 indicates that the specific capacitance observed for NCS-300 is also higher than,41 or comparable to39 several other values reported for NiCo2S4-based electrodes. As it is well-known, the synthetic methodology employed in the preparation of nanomaterials has a high influence on the properties and applications of the materials. It can therefore be speculated that the solventless approach employed herein plays an important on the observed performance. This is due to the fact that the method allows the synthesis of nanomaterials free of long-chained capping ligands,42 which have been shown to be detrimental to the electrochemical performance of the materials.25–27 Contrary to the performance observed for NCS-300, CCS-300 showcased a specific capacitance of 475 F g−1 at the same current density of 0.5 A g−1. Although this performance is much lower than that of NCS-300, it is still higher than43 or comparable to38,44 some of the previously reported CuCo2S4/NiCo2S4-based electrodes. Generally, NCS-300 displayed better energy storage characteristics than CCS-300, which can be attributed to its high conductivity.45 A detailed comparison of the observed specific capacitance values with previously reported values for unary and binary metal oxide/sulfide systems is given in Table S1.†
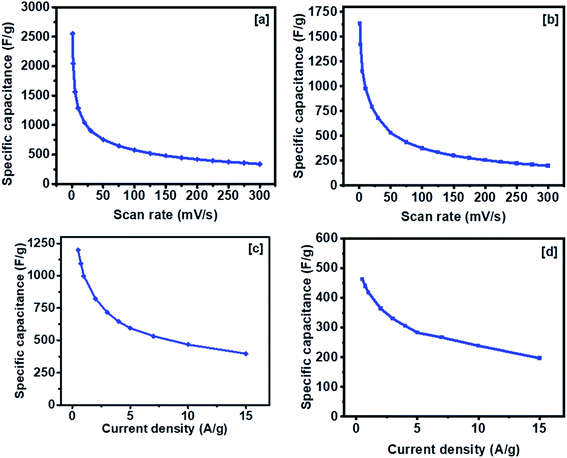 |
| Fig. 8 Variation of specific capacitance at different scan rates from 1 to 300 mV s−1 (a, b). Variation of specific capacitance vs. current density from 0.5 to 15 A g−1 (c, d). | |
3.2.2. Energy generation application. Considering the higher oxidation and reduction activity of the 300 °C annealed samples, we tried to further analyze their electrocatalytic performance on the overall water splitting reaction for the fuel cell applications.The OER and HER activity of the synthesized NCS-300 and CCS-300 electrodes can be summarized in Fig. 9. Unlike supercapacitors, the catalytic activity observed from the polarization curves for NCS-300 and CCS-300 shows a distinct behavior. This is due to two different sets of reaction mechanisms involved in HER and OER reactions. The overall water splitting mechanism includes adsorption and desorption of hydrogen and oxygen atoms by breaking the water molecule over the catalyst surface.46 The thiospinel structure facilitates tetrahedral and octahedral coordination to allow bi-functional oxygen and hydrogen evolution reactions.46b Both the thiospinel catalysts showed high OER and HER activity. The CCS-300 showed higher OER catalysis with a low overpotential of 269 mV than NCS-300 (318 mV) at 10 mA cm−2 (Fig. 9a). On the other hand, NCS-300 showed higher HER catalysis with a low overpotential of 209 mV as compared to CCS-300 (224 mV) at 10 mA cm−2 (Fig. 9b). The high OER/HER activity could be due to coordination between high spin Co3+ and Cu2+/Ni2+ oxidation states with sulfur facilitating higher electronic conductivity for bifunctional catalysis.47 The NiCo2S4 nanowires/Ni foam electrode prepared by Sivanantham et al. achieved low overpotentials of 210 mV for HER and 260 mV for OER at 10 mA cm−2, which is comparable to CCS-300.48 The catalytic activity observed for CCS-300 is also comparable to that of CuCo2S4/NiCo2S4 electrocatalyst synthesized via a hydrothermal approach.49 Furthermore, the OER activity displayed by CCS-300 is superior to that of CuCo2S4 nanoparticles (395 mV at 10 mA cm−2),50 NiCo2S4@N/S-rGO composite (470 mV at 10 mA cm−2),51 and CuCo2S4 nanosheets (310 mV at 10 mA cm−2),52 prepared by other methods. A more detailed comparison for the HER/OER activity of CCS-300 and NCS-300 with previously reported catalysts is summarized in Table S2.† The Tafel plots for HER/OER activity for the two catalysts are summarized in Fig. 9c and d. The kinetics of the catalytic reaction observed from the Tafel plots directly correlate to the HER/OER performance observed during the polarization test in Fig. 9a and b. The Tafel slopes for the OER activity were calculated to be 89 and 103 mV dec−1 for NCS-300 and CCS-300, respectively, whereas the Tafel slopes for the HER activity were found to be 150 and 185 mV dec−1 for NCS-300 and CCS-300, respectively. For comparison purposes, the electrochemical performance of commercial Pt catalyst was also measured. As seen in Fig. S3,† Pt is a good catalyst for the HER process (overpotential of 33 mV); however, the catalyst displays an overpotential of 545 mV for the OER process, which is higher than that displayed by CCS-300 (269 mV).
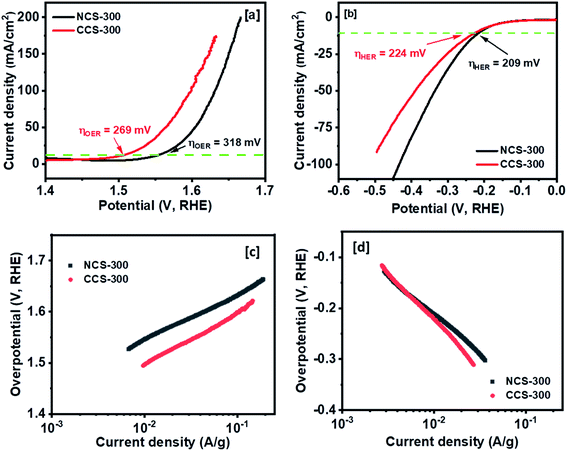 |
| Fig. 9 OER activity for NCS-300 and CCS-300. Polarization curves for OER activity (a) and HER activity (b) at a scan rate of 2 mV s−1. Tafel plots of OER (c) and HER (d) process for NCS-300 and CCS-300. | |
The electrocatalytic stability test for both the catalyst electrodes was further examined for 2000 cycles. As shown in Fig. 10a, both the catalysts showed a negligible change in their OER/HER catalytic performance and maintained their low overpotential values for 2000 cycles. The OER chronoamperometry curves in Fig. 10b shows that both the catalysts retained their high current value corresponding to stable catalytic activity after continuous polarization for more than 15 h.
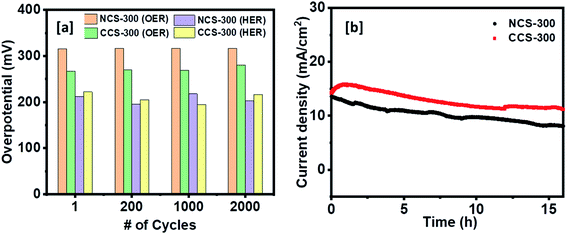 |
| Fig. 10 (a) The overpotential values at 10 mA cm−2 for HER and OER reactions for stability test for 2000 cycles. (b) Chronoamperometry stability test for NCS-300 and CCS-300. | |
The electrochemical surface area of NCS-300 and CCS-300 was calculated by performing CV at various scan rates in non-faradic regions. The plotting relation of current density with scan rate is found to be linear. The slope was observed to be 38 mC cm−2 for NCS-300 and 39.4 mC cm−2 for CCS-300. The higher slope (electrochemical surface area) of CCS-300 suggests better electrochemical properties for the water-splitting application (Fig. 11).
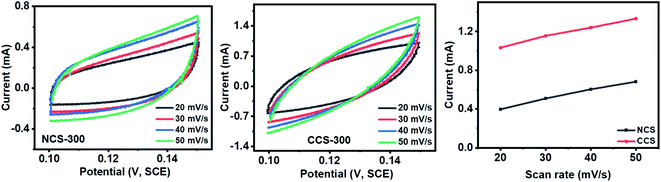 |
| Fig. 11 CV curves at various scan rates for NCS-300 and CCS-300. | |
4. Conclusion
In summary, we have employed a facile, solventless approach to synthesize uncapped NiCo2S4 and CuCo2S4 nanoparticles for supercapacitance and water splitting applications. The synthesis was achieved via thermolysis of respective metal ethyl xanthate precursors at 200 and 300 °C. p-XRD and TEM analyses reveal the synthesis of highly crystalline phase pure nanomaterials with some degree of particle–particle agglomeration. Electrochemical characterizations indicate that the particles synthesized at a high temperature of 300 °C exhibits better energy storage characteristics. The highest specific capacitance of 1201 F g−1 at a current density of 0.5 A g−1 was observed for NiCo2S4 nanoparticles, whereas CuCo2S4 nanoparticles displayed a specific capacitance of 475 F g−1 at the same current density. Conversely, the best electrocatalytic activity was observed for CuCo2S4 nanoparticles, which require overpotentials of 269 mV for OER, and 224 mV for HER, to deliver a current density of 10 mA cm−2. The stability of the catalysts was examined for 2000 cycles in which a minor change in both OER and HER activities was observed.
Conflicts of interest
There are no conflicts to declare.
Acknowledgements
The authors are grateful to the National Research Foundation (NRF) through the South African Research Chair initiative (SARChI) for financial support (Grant number: 64820). M. D. K. also thanks for funding from the European Union's Horizon 2020 research and innovation program under the Marie Skłodowska-Curie grant agreement no. 847413 for funding. Scientific work published as part of an international co-financed project founded from the program of the Minister of Science and Higher Education entitled “PMW” in the years 2020–2024; agreement no. 5005/H2020-MSCA-COFUND/2019/2.
References
-
(a) P. Simon, Y. Gogotsi and B. Dunn, Where do batteries end and supercapacitors begin?, Science, 2014, 343(6176), 1210–1211 CrossRef CAS PubMed;
(b) P. Kulkarni, S. Nataraj, R. G. Balakrishna, D. Nagaraju and M. Reddy, Nanostructured binary and ternary metal sulfides: synthesis methods and their application in energy conversion and storage devices, J. Mater. Chem. A, 2017, 5(42), 22040–22094 RSC.
- C. Yang, L. Zhang, N. Hu, Z. Yang, H. Wei and Y. Zhang, Reduced graphene oxide/polypyrrole nanotube papers for flexible all-solid-state supercapacitors with excellent rate capability and high energy density, J. Power Sources, 2016, 302, 39–45 CrossRef CAS.
- Y. Yan, B. Y. Xia, B. Zhao and X. Wang, A review on noble-metal-free bifunctional heterogeneous catalysts for overall electrochemical water splitting, J. Mater. Chem. A, 2016, 4(45), 17587–17603 RSC.
-
(a) S. Anantharaj, S. R. Ede, K. Sakthikumar, K. Karthick, S. Mishra and S. Kundu, Recent trends and perspectives in electrochemical water splitting with an emphasis on sulfide, selenide, and phosphide catalysts of Fe, Co, and Ni: a review, ACS Catal., 2016, 6(12), 8069–8097 CrossRef CAS;
(b) X. Zou and Y. Zhang, Noble metal-free hydrogen evolution catalysts for water splitting, Chem. Soc. Rev., 2015, 44(15), 5148–5180 RSC.
-
(a) U. Shamraiz, A. Badshah and B. Raza, Ultrafine α-CoOOH Nanorods Activated with Iron for Exceptional Oxygen Evolution Reaction, Langmuir, 2020, 36(9), 2223–2230 CrossRef CAS;
(b) U. Shamraiz, B. Raza, S. Ullah, A. Badshah and M. A. Nadeem, Low cost efficient Sr(OH)2 promoted Pd/rGO electrocatalyst for direct alcohol fuel cell, Appl. Surf. Sci., 2020, 507, 145022 CrossRef CAS;
(c) U. Shamraiz, Z. Ahmad, B. Raza, A. Badshah, S. Ullah and M. A. Nadeem, CaO promoted Graphene-Supported Palladium Nanocrystals as a Universal Electrocatalyst for Direct Liquid Fuel Cells, ACS Appl. Mater. Interfaces, 2020, 12, 4396–4404 CrossRef CAS.
- H. Wang, J. Li, K. Li, Y. Lin, J. Chen, L. Gao, V. Nicolosi, X. Xiao and J.-M. Lee, Transition metal nitrides for electrochemical energy applications, Chem. Soc. Rev., 2021, 50(2), 1354–1390 RSC.
- P. Prabhu, V. Jose and J.-M. Lee, Design strategies for development of TMD-based heterostructures in electrochemical energy systems, Matter, 2020, 2(3), 526–553 CrossRef.
- J. Chen, C. Fan, X. Hu, C. Wang, Z. Huang, G. Fu, J. M. Lee and Y. Tang, Hierarchically porous Co/CoxMy (M = P, N) as an efficient Mott–Schottky electrocatalyst for oxygen evolution in rechargeable Zn–air batteries, Small, 2019, 15(28), 1901518 CrossRef.
-
(a) X. Hu, Y. Chen, M. Zhang, G. Fu, D. Sun, J.-M. Lee and Y. Tang, Alveolate porous carbon aerogels supported Co9S8 derived from a novel hybrid hydrogel for bifunctional oxygen electrocatalysis, Carbon, 2019, 144, 557–566 CrossRef CAS;
(b) L. Dai, D. W. Chang, J. B. Baek and W. Lu, Carbon nanomaterials for advanced energy conversion and storage, Small, 2012, 8(8), 1130–1166 CrossRef CAS PubMed;
(c) G. Fu, Y. Tang and J. M. Lee, Recent Advances in Carbon-Based Bifunctional Oxygen Electrocatalysts for Zn–Air Batteries, ChemElectroChem, 2018, 5(11), 1424–1434 CrossRef CAS;
(d) G. Fu, X. Yan, Y. Chen, L. Xu, D. Sun, J. M. Lee and Y. Tang, Boosting bifunctional oxygen electrocatalysis with 3D graphene aerogel-supported Ni/MnO particles, Adv. Mater., 2018, 30(5), 1704609 CrossRef;
(e) V. Jose, H. Hu, E. Edison, W. Manalastas Jr, H. Ren, P. Kidkhunthod, S. Sreejith, A. Jayakumar, J. M. V. Nsanzimana and M. Srinivasan, Modulation of Single Atomic Co and Fe Sites on Hollow Carbon Nanospheres as Oxygen Electrodes for Rechargeable Zn–Air Batteries, Small Methods, 2021, 5(2), 2000751 CrossRef CAS.
-
(a) G. Fu, J. Wang, Y. Chen, Y. Liu, Y. Tang, J. B. Goodenough and J. M. Lee, Exploring Indium-Based Ternary Thiospinel as Conceivable High-Potential Air-Cathode for Rechargeable Zn–Air Batteries, Adv. Energy Mater., 2018, 8(31), 1802263 CrossRef;
(b) G. Fu, Y. Wang, Y. Tang, K. Zhou, J. B. Goodenough and J.-M. Lee, Superior oxygen electrocatalysis on nickel indium thiospinels for rechargeable Zn–air batteries, ACS Mater. Lett., 2019, 1(1), 123–131 CrossRef CAS.
- L.-L. Feng, G. Yu, Y. Wu, G.-D. Li, H. Li, Y. Sun, T. Asefa, W. Chen and X. Zou, High-index faceted Ni3S2 nanosheet arrays as highly active and ultrastable electrocatalysts for water splitting, J. Am. Chem. Soc., 2015, 137(44), 14023–14026 CrossRef CAS PubMed.
- H. Chen, J. Jiang, L. Zhang, H. Wan, T. Qi and D. Xia, Highly conductive NiCo2S4 urchin-like nanostructures for high-rate pseudocapacitors, Nanoscale, 2013, 5(19), 8879–8883 RSC.
-
(a) L. Shen, J. Wang, G. Xu, H. Li, H. Dou and X. Zhang, NiCo2S4 Nanosheets Grown on Nitrogen-Doped Carbon Foams as an Advanced Electrode for Supercapacitors, Adv. Energy Mater., 2015, 5(3), 1400977 CrossRef;
(b) S. Shinde, M. Jalak, G. Ghodake, N. Maile, V. Kumbhar, D. Lee, V. Fulari and D.-Y. Kim, Chemically synthesized nanoflakes-like NiCo2S4 electrodes for high-performance supercapacitor application, Appl. Surf. Sci., 2019, 466, 822–829 CrossRef CAS.
- H. Chen, X. Ma and P. K. Shen, NiCo2S4 nanocores in situ encapsulated in graphene sheets as anode materials for lithium-ion batteries, Chem. Eng. J., 2019, 364, 167–176 CrossRef CAS.
- K. Liu, A. Wei, J. Liu, Z. Liu, Z. Xiao and Y. Zhao, NiCo2S4 nanosheet thin film counter electrodes prepared by a two-step approach for dye-sensitized solar cells, Mater. Lett., 2018, 217, 185–188 CrossRef CAS.
- Y. Gong, J. Wang, Y. Lin, Z. Yang, H. Pan and Z. Xu, Synthesis of 1D to 3D nanostructured NiCo2S4 on nickel foam and their application in oxygen evolution reaction, Appl. Surf. Sci., 2019, 476, 600–607 CrossRef CAS.
- H. Fan, H. Yu, X. Wu, Y. Zhang, Z. Luo, H. Wang, Y. Guo, S. Madhavi and Q. Yan, Controllable preparation of square nickel chalcogenide (NiS and NiSe2) nanoplates for superior Li/Na ion storage properties, ACS Appl. Mater. Interfaces, 2016, 8(38), 25261–25267 CrossRef CAS.
-
(a) S. Czioska, J. Wang, X. Teng and Z. Chen, Hierarchically structured CuCo2S4 nanowire arrays as efficient bifunctional electrocatalyst for overall water splitting, ACS Sustainable Chem. Eng., 2018, 6(9), 11877–11883 CrossRef CAS;
(b) Y. Gong, J. Zhao, H. Wang and J. Xu, CuCo2S4/reduced graphene oxide nanocomposites synthesized by one-step solvothermal method as anode materials for sodium ion batteries, Electrochim. Acta, 2018, 292, 895–902 CrossRef CAS;
(c) R. Verma, R. Kothandaraman and U. Varadaraju, In situ carbon coated CuCo2S4 anode material for Li-ion battery applications, Appl. Surf.
Sci., 2017, 418, 30–39 CrossRef CAS;
(d) S. Xie, J. Gou, B. Liu and C. Liu, Synthesis of cobalt-doped nickel sulfide nanomaterials with rich edge sites as high-performance supercapacitor electrode materials, Inorg. Chem. Front., 2018, 5(5), 1218–1225 RSC;
(e) L.-Q. Fan, F. Pan, Q.-M. Tu, Y. Gu, J.-L. Huang, Y.-F. Huang and J.-H. Wu, Synthesis of CuCo2S4 nanosheet arrays on Ni foam as binder-free electrode for asymmetric supercapacitor, Int. J. Hydrogen Energy, 2018, 43(52), 23372–23381 CrossRef CAS.
- D. J. Vaughan, Sulfide mineralogy and geochemistry, Walter de Gruyter GmbH & Co KG, 2018, vol. 61 Search PubMed.
- R. Bouchard, P. Russo and A. Wold, Preparation and electrical properties of some thiospinels, Inorg. Chem., 1965, 4(5), 685–688 CrossRef CAS.
- J. Jiang, Y. Zhang, P. Nie, G. Xu, M. Shi, J. Wang, Y. Wu, R. Fu, H. Dou and X. Zhang, Progress of nanostructured electrode materials for supercapacitors, Adv. Sustainable Syst., 2018, 2(1), 1700110 CrossRef.
- J. Munaro, P. Dolcet, S. Nappini, E. Magnano, N. Dengo, G. Lucchini, A. Speghini and S. Gross, The role of the synthetic pathways on properties of Ag2S nanoparticles for photothermal applications, Appl. Surf. Sci., 2020, 514, 145856 CrossRef CAS.
-
(a) D. Wang, T. Xie and Y. Li, Nanocrystals: Solution-based synthesis and applications as nanocatalysts, Nano Res., 2009, 2(1), 30–46 CrossRef CAS;
(b) S. V. Ganachari, N. R. Banapurmath, B. Salimath, J. S. Yaradoddi, A. S. Shettar, A. M. Hunashyal, A. Venkataraman, P. Patil, H. Shoba and G. B. Hiremath, Synthesis Techniques for Preparation of Nanomaterials, Handbook of Ecomaterials, Springer, Cham, 2017. DOI: DOI:10.1007/978-3-319-48281-1_149-1.
- N. L. Pickett and P. O'Brien, Syntheses of semiconductor nanoparticles using single-molecular precursors, Chem. Rec., 2001, 1(6), 467–479 CrossRef CAS PubMed.
- D. Ung and B. M. Cossairt, The Effect of Surface Ligands on CoP for the Hydrogen Evolution Reaction, ACS Appl. Energy Mater., 2019, 2(3), 1642–1645 CrossRef CAS.
- Y. Lu, Y. Wang and W. Chen, Silver nanorods for oxygen reduction: strong effects of protecting ligand on the electrocatalytic activity, J. Power Sources, 2011, 196(6), 3033–3038 CrossRef CAS.
- D. A. Henckel, O. Lenz and B. M. Cossairt, Effect of ligand coverage on hydrogen evolution catalyzed by colloidal WSe2, ACS Catal., 2017, 7(4), 2815–2820 CrossRef CAS.
- D. Li, C. Wang, D. Tripkovic, S. Sun, N. M. Markovic and V. R. Stamenkovic, Surfactant removal for colloidal nanoparticles from solution synthesis: the effect on catalytic performance, ACS Catal., 2012, 2(7), 1358–1362 CrossRef CAS.
- Z. L. Wang, J. M. Petroski, T. C. Green and M. A. El-Sayed, Shape transformation and surface melting of cubic and tetrahedral platinum nanocrystals, J. Phys. Chem. B, 1998, 102(32), 6145–6151 CrossRef CAS.
- M. D. Khan, G. Murtaza, N. Revaprasadu and P. O'Brien, Synthesis of chalcopyrite-type and thiospinel minerals/materials by low temperature melts of xanthates, Dalton Trans., 2018, 47(27), 8870–8873 RSC.
-
(a) M. D. Khan, M. Aamir, G. Murtaza, M. A. Malik and N. Revaprasadu, Structural investigations of SnS1−xSex solid solution synthesized from chalcogeno-carboxylate complexes of organo-tin by colloidal and solvent-less routes, Dalton Trans., 2018, 47(30), 10025–10034 RSC;
(b) R. Akram, M. D. Khan, C. Zequine, C. Zhao, R. K. Gupta, M. Akhtar, J. Akhtar, M. A. Malik, N. Revaprasadu and M. H. Bhatti, Cobalt sulfide nanoparticles: Synthesis, water splitting and supercapacitance studies, Mater. Sci. Semicond. Process., 2020, 109, 104925 CrossRef CAS;
(c) G. B. Shombe, M. D. Khan, C. Zequine, C. Zhao, R. K. Gupta and N. Revaprasadu, Direct solvent free synthesis of bare α-NiS, β-NiS and α-β-NiS composite as excellent electrocatalysts: Effect of self-capping on supercapacitance and overall water splitting activity, Sci. Rep., 2020, 10(1), 1–14 CrossRef PubMed.
- S. R. Nxele, P. Mashazi and T. Nyokong, Electrode modification using alkynyl substituted Fe(II) phthalocyanine via electrografting and click chemistry for electrocatalysis, Electroanalysis, 2015, 27(10), 2468–2478 CrossRef CAS.
-
(a) M. Akhtar, M. A. Malik, F. Tuna and P. O'Brien, The synthesis of iron sulfide nanocrystals from tris(O-alkylxanthato) iron(III) complexes, J. Mater. Chem. A, 2013, 1(31), 8766–8774 RSC;
(b) E. A. Lewis, P. D. McNaughter, Z. Yin, Y. Chen, J. R. Brent, S. A. Saah, J. Raftery, J. A. Awudza, M. A. Malik and P. O'Brien, In situ synthesis of PbS nanocrystals in polymer thin films from lead(II) xanthate and dithiocarbamate complexes: evidence for size and morphology control, Chem. Mater., 2015, 27(6), 2127–2136 CrossRef CAS;
(c) C. Zhang, S. Zhang, L. Yu, Z. Zhang, P. Zhang and Z. Wu, Size-controlled synthesis of monodisperse Ag2S nanoparticles by a solventless thermolytic method, Mater. Lett., 2012, 85, 77–80 CrossRef CAS.
-
(a) C. J. Carmalt, C. W. Dinnage, I. P. Parkin and J. W. Steed, Synthesis and characterization of a homoleptic thiolate complex of titanium(IV), Inorg. Chem., 2000, 39(12), 2693–2695 CrossRef CAS PubMed;
(b) N. Thammakan and E. Somsook, Synthesis and thermal decomposition of cadmium dithiocarbamate complexes, Mater. Lett., 2006, 60(9–10), 1161–1165 CrossRef CAS;
(c) M. L. Redígolo, D. S. Koktysh, K. van Benthem, S. J. Rosenthal and J. H. Dickerson, Europium sulfide nanoparticles in the sub-2 nm size regime, Mater. Chem. Phys., 2009, 115(2–3), 526–529 CrossRef;
(d) Y. S. Niwate and S. S. Garje, Preparation of Tin Chalcogenide Nanoparticles Using Tribenzyltin(IV) Semi-and Thiosemicarbazone Precursors, Synth. React. Inorg., Met.-Org., Nano-Met. Chem., 2011, 41(1), 36–43 CrossRef CAS.
- P. D. McNaughter, S. A. Saah, M. Akhtar, K. Abdulwahab, M. A. Malik, J. Raftery, J. A. Awudza and P. O'Brien, The effect of alkyl chain length on the structure of lead(II) xanthates and their decomposition to PbS in melt reactions, Dalton Trans., 2016, 45(41), 16345–16353 RSC.
- M. D. Khan, M. Aamir, M. Sohail, S. Bhoyate, M. Hyatt, R. K. Gupta, M. Sher and N. Revaprasadu, Electrochemical investigation of uncapped AgBiS2 (schapbachite) synthesized using in situ melts of xanthate precursors, Dalton Trans., 2019, 48(11), 3714–3722 RSC.
-
(a) S. Bhoyate, P. K. Kahol and R. K. Gupta, Nanostructured materials for supercapacitor applications, 2018 Search PubMed;
(b) S. Bhoyate, K. Mensah-Darkwa, P. K. Kahol and R. K. Gupta, Recent development on nanocomposites of graphene for supercapacitor applications, Curr. Graphene Sci., 2017, 1(1), 26–43 CrossRef.
- J. Pu, F. Cui, S. Chu, T. Wang, E. Sheng and Z. Wang, Preparation and electrochemical characterization of hollow hexagonal NiCo2S4 nanoplates as pseudocapacitor materials, ACS Sustainable Chem. Eng., 2014, 2(4), 809–815 CrossRef CAS.
- J. Xu, Y. Yang, H. Chu, J. Tang, Y. Ge, J. Shen and M. Ye, Novel NiCo2S4@reduced graphene oxide@carbon nanotube nanocomposites for high performance supercapacitors, RSC Adv., 2016, 6(102), 100504–100510 RSC.
- Z. Wu, X. Pu, X. Ji, Y. Zhu, M. Jing, Q. Chen and F. Jiao, High energy density asymmetric supercapacitors from mesoporous NiCo2S4 nanosheets, Electrochim. Acta, 2015, 174, 238–245 CrossRef CAS.
-
(a) H. Chen, X. L. Liu, J. M. Zhang, F. Dong and Y. X. Zhang, Rational synthesis of hybrid NiCo2S4@MnO2 heterostructures for supercapacitor electrodes, Ceram. Int., 2016, 42(7), 8909–8914 CrossRef CAS;
(b) T. Wang, Q. Le, G. Zhang, S. Zhu, B. Guan, J. Zhang, S. Xing and Y. Zhang, Facile preparation and sulfidation analysis for activated multiporous carbon@NiCo2S4 nanostructure with enhanced supercapacitive properties, Electrochim. Acta, 2016, 211, 627–635 CrossRef CAS.
- E. Lewis, S. Haigh and P. O'Brien, The synthesis of metallic and semiconducting nanoparticles from reactive melts of precursors, J. Mater. Chem. A, 2014, 2(3), 570–580 RSC.
-
(a) L. Chen, Y. Zuo, Y. Zhang and Y. Gao, A novel CuCo2S4/polyacrylonitrile ink for flexible film supercapacitors, Mater. Lett., 2018, 215, 268–271 CrossRef CAS;
(b) H. Li, Z. Li, Z. Wu, M. Sun, S. Han, C. Cai, W. Shen, X. Liu and Y. Fu, Enhanced electrochemical performance of CuCo2S4/carbon nanotubes composite as electrode material for supercapacitors, J. Colloid Interface Sci., 2019, 549, 105–113 CrossRef CAS PubMed.
- J. Cheng, S. Gao, P. Zhang, B. Wang, X. Wang and F. Liu, Influence of crystallinity of CuCo2S4 on its supercapacitive behavior, J. Alloys Compd., 2020, 825, 153984 CrossRef CAS.
- C. Xia, P. Li, A. N. Gandi, U. Schwingenschlögl and H. N. Alshareef, Is NiCo2S4 really a semiconductor?, Chem. Mater., 2015, 27(19), 6482–6485 CrossRef CAS.
-
(a) Z. W. Seh, J. Kibsgaard, C. F. Dickens, I. Chorkendorff, J. K. Nørskov and T. F. Jaramillo, Combining theory and experiment in electrocatalysis: Insights into materials design, Science, 2017, 355(6321), eaad4998 CrossRef PubMed;
(b) N.-T. Suen, S.-F. Hung, Q. Quan, N. Zhang, Y.-J. Xu and H. M. Chen, Electrocatalysis for the oxygen evolution reaction: recent development and future perspectives, Chem. Soc. Rev., 2017, 46(2), 337–365 RSC.
-
(a) C. Zhang, S. Bhoyate, C. Zhao, P. K. Kahol, N. Kostoglou, C. Mitterer, S. J. Hinder, M. A. Baker, G. Constantinides and K. Polychronopoulou, Electrodeposited nanostructured CoFe2O4 for overall water splitting and supercapacitor applications, Catalysts, 2019, 9(2), 176 CrossRef;
(b) M. Ghimire, S. Bhoyate, R. Gupta, X. Shen, F. Perez, J. Alam and S. Mishra, Physical Properties and Theoretical Study of NixCo3−xO4 (0 ≤ x ≤ 1.5) Nanostructures as High-Performance Electrode Materials for Supercapacitors, J. Nanosci. Nanotechnol., 2019, 19(8), 4481–4494 CrossRef CAS;
(c) C. Zequine, S. Bhoyate, F. Wang, X. Li, K. Siam, P. Kahol and R. K. Gupta, Effect of solvent for tailoring the nanomorphology of multinary CuCo2S4 for overall water splitting and energy storage, J. Alloys Compd., 2019, 784, 1–7 CrossRef CAS;
(d) C. Zhao, C. Zhang, S. Bhoyate, P. K. Kahol, N. Kostoglou, C. Mitterer, S. Hinder, M. Baker, G. Constantinides and K. Polychronopoulou, Nanostructured Fe–Ni Sulfide: A Multifunctional Material for Energy Generation and Storage, Catalysts, 2019, 9(7), 597 CrossRef CAS.
- A. Sivanantham, P. Ganesan and S. Shanmugam, Hierarchical NiCo2S4 nanowire arrays supported on Ni foam: an efficient and durable bifunctional electrocatalyst for oxygen and hydrogen evolution reactions, Adv. Funct. Mater., 2016, 26(26), 4661–4672 CrossRef CAS.
- L. Ma, J. Liang, T. Chen, Y. Liu, S. Li and G. Fang, 3D CuCo2S4/NiCo2S4 core–shell composites as efficient bifunctional electrocatalyst electrodes for overall water splitting, Electrochim. Acta, 2019, 326, 135002 CrossRef CAS.
- A. M. Wiltrout, C. G. Read, E. M. Spencer and R. E. Schaak, Solution synthesis of thiospinel CuCo2S4 nanoparticles, Inorg. Chem., 2016, 55(1), 221–226 CrossRef CAS PubMed.
- Q. Liu, J. Jin and J. Zhang, NiCo2S4@graphene as a bifunctional electrocatalyst for oxygen reduction and evolution reactions, ACS Appl. Mater. Interfaces, 2013, 5(11), 5002–5008 CrossRef CAS PubMed.
- M. Chauhan, K. P. Reddy, C. S. Gopinath and S. Deka, Copper cobalt sulfide nanosheets realizing a promising electrocatalytic oxygen evolution reaction, ACS Catal., 2017, 7(9), 5871–5879 CrossRef CAS.
Footnote |
† Electronic supplementary information (ESI) available. See DOI: 10.1039/d1ra02309h |
|
This journal is © The Royal Society of Chemistry 2021 |