DOI:
10.1039/D1RA01583D
(Paper)
RSC Adv., 2021,
11, 10524-10531
Ancillary ligand modulated stereoselective self-assembly of triple-stranded Eu(III) helicate featuring circularly polarized luminescence†
Received
27th February 2021
, Accepted 4th March 2021
First published on 10th March 2021
Abstract
Creating optically pure metal assemblies is a hot research topic in the realms of chiral supramolecules. Here, three new triple-stranded europium(III) helicates Eu2L3(L′)2 [L = 4,4′-bis(4,4,4-trifluoro-1,3-dioxobutyl)diphenyl sulphide; L′ = 1,10-phenanthroline (Phen) or R/S-2,2′-bis(diphenylphosphinyl)-1,1′-binaphthyl (R/S-BINAPO)] were synthesized in order to investigate the effects of ancillary ligands on controlling the stereoselective self-assembly of lanthanide helicates. X-ray single crystal structure analysis showed that Eu2L3(Phen)2 crystalized in an achiral space group P
with the equivalent amount of P and M helicates in one single cell. The isolated Eu2L3(S-BINAPO)2 and Eu2L3(R-BINAPO)2 were verified to be enantiopure by 1H, 19F, 31P NMR and DOSY NMR analyses. Additionally, the mirror-image CD spectra also demonstrated the successful syntheses of the enantiomers and the presence of an effective chirality transformation from BINAPO to achiral L. Furthermore, the perfect mirror-image circularly polarized luminescence (CPL) spectra of Eu2L3(S-BINAPO)2 and Eu2L3(R-BINAPO)2 indicated the existence of the excited state chirality of the Eu3+ center associated with |glum| values reaching 0.112. In addition, the photophysical properties of three helicates were also discussed.
Introduction
In recent years, circularly polarized luminescence (CPL) materials1 have attracted great interest because of their potential application in 3D displays,2 optical storage,3 communication of spin information4 and CPL probes.5 Lanthanide helicates are regarded as the favorable candidates of CPL materials in view of their supramolecular helical chirality and the characteristic electron transition from Ln3+ ions.6 The partial magnetic dipole character endowed the Ln3+ ions with a higher luminescence dissymmetry factor (glum),7 typically in the range of 10−2–0.5,8 which is obviously larger than those of pure organic luminophores (glum = 10−5–10−3).9 To date, the highest glum value for CPL materials in solution was recorded to be 1.38 from a Eu3+ complex, Cs[Eu((+)-hfbc)4].10
On the other hand, the chirality amplification effect arising from the supramolecular chirality provides a structural foundation for the helicates to produce the large glum values. In 2009, Gunnlaugsson et al. prepared a chiral lanthanide helicate and reported its CPL property for the first time.11 In recent years, Gunnlaugsson,12 Sun,13 and Law14 have successfully prepared a few optical pure dinuclear lanthanide helicates based on chiral 2,6-dipicolinic amides derivatives, and some of which have been used for CPL study. Although the glum values were satisfactory, the examples for the preparation of chiral lanthanide helicates were still rare. The introduction of chiral ancillary ligand was proved to be an effective way to control the stereoselective of lanthanide assemblies.15
Our group has been concerned with the research of luminescent lanthanide helicates composed of β-diketonate ligands.16 The highly effective sensitizing ability of β-diketones ensured the high luminescence quantum yields (QYs) of helicates. More recently, we reported several examples of chiral helicates featured with CPL properties, which showed the moderate |glum| values.17 Generally, the large degree of twisting of helix is favorable for chirality amplification, and probably brings the increase of luminescence dissymmetry.
Herein, three optical active and inactive helicates were constructed by employing an achiral Phen and two chiral R- and S-BINAPO as the second ligands. Single crystal X-ray crystallographic analysis showed Eu2L3(Phen)2 was a racemic mixture with a pair of ΔΔ- and ΛΛ-helicate existing in one single cell. While the diastereoselective self-assembly during the preparation of Eu2L3(S-BINAPO)2 and Eu2L3(R-BINAPO)2 can be verified by NMR spectra and semiempirical geometry optimization. The photophysical measurements showed that the helicates all present the satisfactory luminescence quantum yields, 31% and 34% for Eu2L3(Phen)2 and Eu2L3(R/S-BINAPO)2, respectively. In addition, the chiral helicates Eu2L3(S-BINAPO)2 and Eu2L3(R-BINAPO)2 present a modest circularly polarized luminescence dissymmetry (|glum| = 0.112 at 595 nm).
Results and discussion
Characterization of L and complexes
The syntheses routes of L and its corresponding complexes Eu2L3(Phen)2 and Eu2L3(R/S-BINAPO)2 are outlined in Scheme 1. The C2-symmetric achiral bis-β-diketone 4,4′-bis(4,4,4-trifluoro-1,3-dioxobutyl)phenyl sulphide (L) was prepared via two steps, the first was the Friedel–Crafts acylation of phenyl sulfide, then followed with a Claisen condensation of 4,4′-diacetyl phenyl sulfide and ethyl trifluoroacetate. The corresponding intermediate and ligand were characterized by ESI-TOF-MS and 1H NMR (Fig. S1–S3†).
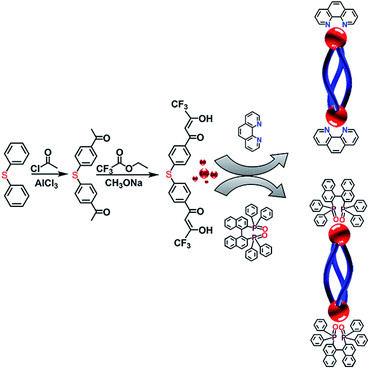 |
| Scheme 1 Synthetic routes of L and the corresponding complexes Eu2L3(Phen)2 and Eu2L3(BINAPO)2. | |
The triple-stranded helicates Ln2L3(L′)2 were isolated by stirring a 3
:
2
:
2 mixture of L, L′ and LnCl3·6H2O (Ln = Gd, Eu) at room temperature in CH3OH. High-resolution ESI-TOF-MS analyses affirmed the successful preparation of triple-stranded helicates. For instance, Fig. 1a presents three clusters of peaks, corresponding to the positive charged [M + H]+, [M + K]+ and [M + Na]+ species of Eu2L3(S-BINAPO)2, at m/z 2995.1158, 3017.1653 and 3033.1340. The mass spectra of the other complexes also showed the expected molecule ions peaks (Fig. S4–S7†). The assignments were further affirmed by comparing the isotopic distributions of the experimental with simulated results.
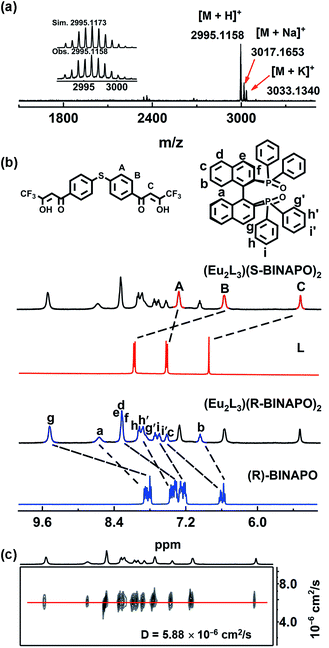 |
| Fig. 1 (a) ESI-TOF-MS of Eu2L3(S-BINAPO)2 with inset representing the simulated (Sim.) and observed (Obs.) isotopic distributions. (b) 1H NMR (400 MHz) spectra of L, R-BINAPO and Eu2L3(R-BINAPO)2 and Eu2L3(S-BINAPO)2 (THF-d8). (c) 1H DOSY spectrum of Eu2L3(S-BINAPO)2 in THF-d8. | |
1H, 31P, 19F NMR, and DOSY NMR results also supported the successful preparation of Eu2L3(S-BINAPO)2 and Eu2L3(R-BINAPO)2 and their stabilities in THF (Fig. 1b, S8 and S9†). As shown in Fig. 1b, 1H NMR of Eu2L3(S-BINAPO)2 shows a single set of clear and broadening signals from L and R/S-BINAPO. Considering the absence of C3 symmetry of the helicates, three sets of H signals from three different ligands should be observed. However, with the influence of paramagnetism of Eu3+ ion, for instance, only a single peak attributed to methine HC resonance of β-diketone was observed at 5.34 ppm, not the expected three peaks. A more facile way to infer the formation of a single species was the employment of 31P and 19F NMR, in which only the singlet peaks were observed (Fig. S8 and S9†). Additionally, one single diffusion rate of Eu2L3(S-BINAPO)2 (D = 5.88 × 10−6 cm2 s−1) in 1H NMR diffusion ordered spectroscopy (DOSY) also supported the presence a single species in THF (Fig. 1c).
X-ray single crystal structure of Eu2L3(Phen)2
The single crystals of Eu2L3(Phen)2 were grown by diffusion of diethyl ether to a THF/toluene solution of the complex. The structural analysis revealed that Eu2L3(Phen)2 was the expected triple-stranded helicate with each Eu3+ center ligated by six oxygen (O) atoms from three β-diketones and two nitrogen (N) atoms from Phen (Fig. 2a), and adopting a square antiprism as coordination geometry (Fig. S10†). The Eu–N and Eu–O distances are in ranges of 2.331–2.383 Å and 2.574–2.617 Å, respectively. The Eu–Eu distance in one helicate reached to 13.229 Å. The corresponding crystallographic parameters were list in Table S1.† It was found that the Eu2L3(Phen)2 crystalized in an achiral triclinic space group P
, where a pair of racemic mixtures of ΔΔ-Eu2L3(Phen)2 and ΛΛ-Eu2L3(Phen)2 were observed in one single cell. This result indicated that the absence of chiral element in self-assembly process will result in the formation of the racemic helicate.
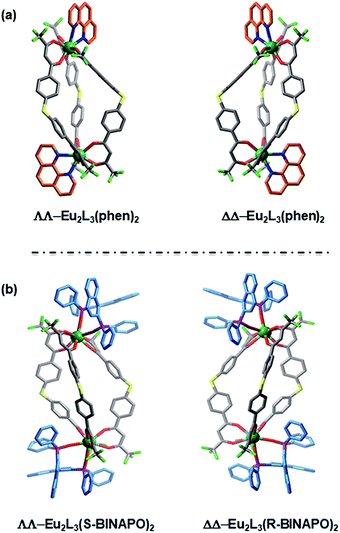 |
| Fig. 2 (a) X-ray crystallographic structure of Eu2L3(Phen)2 and (b) Sparkle/RM1 optimized geometries of Eu2L3(S-BINAPO)2 and Eu2L3(R-BINAPO)2. | |
As reported by us and others,18 the chiral ancillary ligands could induce the metal supramolecule assemblies to form a single diastereoisomer. Herein, we employed the chiral BINAPO as ancillary ligands to construct the diastereopure helicates. Unfortunately, the single crystals of Eu2L3(R/S-BINAPO)2 were not obtained, although several methods had been tried. To better understand the chiral induction, we resorted to a molecular mechanical modeling built from LUMPAC 3.0 software with a Sparkle/RM1 model.19 Taking the ΔΔ-Eu2L3 helicate as an example, its assembly with R-BINAPO and S-BINAPO were both considered. As can be seen in Fig. S11,† the ΔΔ-Eu2L3 with R-BINAPO as ancillary ligand has a relative lower system energy than that with S-BINAPO. Thus, it was proposed that the R-BINAPO could induce the Eu2L3 to form P helical conformation with the metal center the ΔΔ configuration.
Photoluminescent properties of the complexes
The UV-vis spectra of Eu2L3(Phen)2, Eu2L3(R/S-BINAPO)2 and free L in THF are shown in Fig. 3. In free ligand, two clear bands with λmax = 241 nm (1.8 × 104 dm3 mol−1 cm−1) and λmax = 366 nm (4.1 × 104 dm3 mol−1 cm−1) were observed, which could be attributed to the π–π* transitions localizing at benzene ring and the ILCT transition from benzene ring to β-diketone units, respectively. In comparison with free ligand, three complexes all present the obvious hypsochromic shifts with the absorption maxima from 370 nm to 350 nm. This significant shift arose from the large twisting of L in complexes, which disrupted the conjugation of β-diketone moieties. While the high energy absorbances at 277 and 237 nm were attributed to the absorptions of Phen and R/S-BINAPO, respectively.
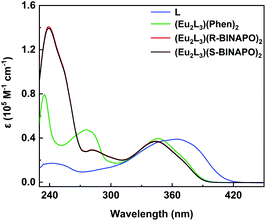 |
| Fig. 3 UV-vis absorption spectra of L (3.3 × 10−6 M) and helicates Eu2L3(Phen)2 and Eu2L3(R/S-BINAPO)2 (1.0 × 10−5 M) in THF. | |
The excitation and emission spectra of three Eu3+ complexes are shown in Fig. 4 and S12.† Upon excitation at 370 nm, three Eu3+ complexes displayed five sharp emission bands at 580, 594, 613, 650 and 702 nm, respectively, relating to the 5D0 → 7FJ (J = 0–4) transitions. In excitation spectra, the spectral bands could be well matched with the absorption bands of complexes in 280–400 nm regions, while the possible energy transfer from ancillary ligands could be excluded due to the absence of corresponding absorbance at high energy bands of 240–260 nm.
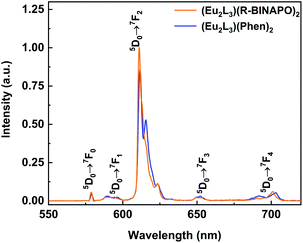 |
| Fig. 4 Emission spectra of complexes Eu2L3(Phen)2 and Eu2L3(R-BINAPO)2 in THF (λex = 370 nm, c = 1.0 × 10−5 M). | |
In emission spectra of Eu3+ complexes, the 5D0 → 7F2 and 5D0 → 7F1 transitions are of special importance. The former is the electric dipole hypersensitive transition, which is usually suggested to be sensitive to the coordination geometry of Eu3+ ion. Whereas, the 5D0 → 7F1 transition is insensitive to the coordination sphere. Thus, the integrated intensity ratio of I(5D0 → 7F2)/I(5D0 → 7F1) was often employed as a probe to infer the coordination geometry symmetry of Eu3+ ion, the larger of this value generally means the larger deviation of the Eu3+ from the highly symmetric coordination geometry.20 Here, the intensity ratios reached to 25.4 and 28.3 for Eu2L3(Phen)2 and Eu2L3(R/S-BINAPO)2, respectively. It indicated the presence of a lower symmetry around Eu3+ ion.
Another manner to infer the symmetry of Eu3+ site is to count the numbers of crystal-field splits.21 On the basis of 5D0 → 7F2 transitions with the five peaks at 612 nm (detailed splits can be seen from the deconvolution analyses in Fig. S13†), the local symmetry around Eu3+ ions in Eu2L3(Phen)2 and Eu2L3(BINAPO)2 were most probably to be C1. However, the assignment of the point group symmetry was not in line with that observed D4d coordination geometry calculated from the single crystal structure of Eu2L3(Phen)2 (Fig. S10†). It is reasonable to consider the possibility of the presence of different coordination geometries around Eu3+ ion in solution and in solid state.22
The large ratio of I(5D0 → 7FJ)/I(5D0 → 7F1) transition generally implies that the complex has a relatively large radiative transition probability as judged from eqn (1).
|
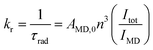 | (1) |
The radiative rate constant (kr) is proportional to the intensity ratio of Itot/IMD, Itot and IMD represent the total integrated emission of 5D0 → 7FJ and 5D0 → 7F1 transitions, respectively. AMD,0 is a constant (14.65 s−1), representing the spontaneous emission probability of 5D0 → 7F1 transition. n is the refractive index of solution.
The large kr value does not indicate the high luminescence efficiency of Eu3+ center, because the non-radiative transition (knr) is inevitable. The both deactivation pathways finally determine the intrinsic quantum yields (ΦEu) as shown in eqn (2).
|
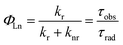 | (2) |
τobs is the experimental lifetimes. From the emission decay curves of Eu
3+ ion monitored at the
5D
0 →
7F
2 transition (Fig. S14 and S15
†), the lifetimes of Eu
2L3(Phen)
2, Eu
2L3(
S-BINAPO)
2 and Eu
2L3(
R-BINAPO)
2 were fitted to be 613, 635 and 635 μs, respectively. On the basis of the calculated radiative lifetimes (
τrad) and measured lifetimes,
ΦEu were calculated to reach up to 74% and 84% for Eu
2L3(Phen)
2 and Eu
2L3(
R/
S-BINAPO)
2. With
eqn (2), the radiative (
kr) and nonradiative rate constants (
knr) were estimated and listed in
Table 1. Additionally, the complexes also showed the large Eu
3+ center luminescence quantum yields (
Φoverall) of 31% and 34% for Eu
2L3(Phen)
2 and Eu
2L3(
R/
S-BINAPO)
2 (Fig. S16–S18
†).
Table 1 Radiative (kr) and nonradiative (knr) decay rate constants, measured emission lifetime of Eu3+ (τobs), sensitization efficiency (ηsens), intrinsic quantum yield (ΦEu), and overall quantum yield (Φoverall), glum values for 5D0 → 7FJ of Eu3+ ion. Error in τobs: ±0.05 ms; 10% relative error in the other values; λex = 370 nm
Complexes |
kr (s−1) |
knr (s−1) |
τobs (μs) |
ΦEu (%) |
ηsens (%) |
Φoverall (%) |
glum 5D0 → 7FJ (J = 0, 1, 2, 3, 4) |
J = 0 |
J = 1 |
J = 2 |
J = 3 |
J = 4 |
Eu2L3(R-BINAPO)2 |
1328 |
245 |
635 |
84 |
40 |
33.7 |
0.006 |
0.112 |
−0.009 |
0.003 |
−0.001 |
Eu2L3(S-BINAPO)2 |
1327 |
247 |
635 |
84 |
41 |
34.3 |
−0.006 |
−0.112 |
0.009 |
−0.029 |
0.001 |
Eu2L3(Phen)2 |
1208 |
422 |
613 |
74 |
42 |
30.8 |
— |
— |
— |
— |
— |
From the eqn (3), the sensitizing efficiency (ηsen) was also calculated. In Eu3+ complexes, the energy level difference ΔE (T1 − 5D0) between the triplet states (T1) of ligands and 5D0 energy level of Eu3+ ion usually determined the sensitizing efficiency. An empirical energy gap to realize a highly effective energy transfer was proposed to be in range of 2500–5000 cm−1 for Eu3+ complexes.23 By virtue of phosphorescence spectra of the Gd3+ complexes, the ΔE were estimated to be 3246 and 2540 cm−1 for Eu2L3(Phen)2 and Eu2L3(R/S-BINAPO)2 (Fig. S19†), which exactly located at the proposed energy gap range. In addition, the possible energy transfer from ancillary ligands to Eu3+ ion was excluded because of the absence of the excitation bands attributing to Phen and BINAPO.
Chiroptical properties of Eu2L3(R/S-BINAPO)2
In view of the introduction of chiral R-BINAPO and S-BINAPO and above spectral analyses, it was inferred that chiral ancillary could regulate the diastereoselective self-assembly of triple-stranded helicate. Therefore, the chirality of the helicate was firstly characterized by CD spectroscopy. From the Fig. 5, the two complexes Eu2L3(S-BINAPO)2 and Eu2L3(R-BINAPO)2 showed mirror-image Cotton effect in ranges of 250–400 nm, relating to the UV-vis absorption region of helicates. Moreover, two clear exciton couplings were observed in range of 250–315 and 315–400 nm, which were ascribed to the transitions from chiral BINAPO and achiral L ligand, respectively. In Eu2L3(R-BINAPO)2, a negative exciton coupling (a negative and positive signals at 300 and 260 nm) attributed to R-BINAPO was observed; conversely, a positive exciton coupling was obtained in case of S-BINAPO. Notably, the achiral L also present the chiroptical signal with a positive exciton coupling for Eu2L3(S-BINAPO)2 and a negative case for Eu2L3(R-BINAPO)2. It implied that the chirality was transferred from chiral phosphine oxide ancillary ligands to achiral L through the generation of supramolecular chirality.
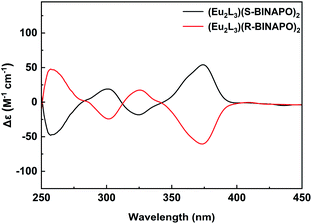 |
| Fig. 5 CD spectra of Eu2L3(S-BINAPO)2 (black line) and Eu2L3(R-BINAPO)2 (red line) in THF (1.0 × 10−5 M). | |
The exciton coupling in metal complexes originated from the interactions of chromophores, in which the electron transition was no longer localized at one ligand, but became delocalization over the entire assemblies. Here, three bis-β-diketone were ligated at two metal centers, which forced them to close together. From the suggested empirical rule,24 the relationships between the absolute configurations of the metal centers and the couplet signs have been built. Generally, the negative exciton couplet related to a Δ configuration of metal center; conversely, the positive exciton couplet correlated a Λ configuration. From this rule, the configurations estimated from the optimized molecular structures were in line with the observed CD patterns.
The excited state chiroptical property can be confirmed from the CPL spectra of helicates. As shown in Fig. 6, a pair of mirror-image CPL signals appeared at the 5D0 → 7FJ (J = 0–4) transition region. In these bands, the 5D0 → 7F2 and 5D0 → 7F1 transitions present the larger CPL intensities than other 5D0 → 7F0,3,4 transition. The strong 5D0 → 7F2 emission was due to the largest emitting intensity at 612 nm. In contrast, the 5D0 → 7F1 transition at 595 nm also displayed a relatively large intensity, although its total emission was low. This mainly benefitted from its magnetic dipole transition characteristic. The luminescence dissymmetry factor, glum value (−2 ≤ glum ≤ 2) is the standard to estimate the excess of left vs. right circularly polarized luminescence, where glum = 2(IL − IR)/(IL + IR), IR and IL refer to the left and right circularly polarized emission intensities, respectively. The |glum| values of the 5D0 → 7FJ transitions were summarized in Table 1. The largest |glum| values were measured to be about 0.112 for 5D0 → 7F1 transition at 595 nm, while the 5D0 → 7F2 transitions were about 0.009. These results were comparable to the previously reported CPL Eu3+ complexes.25
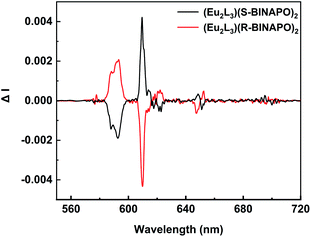 |
| Fig. 6 CPL spectra of Eu2L3(S-BINAPO)2 (black line) and Eu2L3(R-BINAPO)2 (red line) (λex = 370 nm, 1.0 × 10−5 M in THF). | |
Conclusions
In summary, we successfully synthesized an optical inactive helicate Eu2L3(Phen)2 and a pair of optical active enantiomers Eu2L3(S-BINAPO)2 and Eu2L3(R-BINAPO)2 by employing achiral Phen and chiral R/S-BINAPO as second ligands. X-ray crystallographic analysis revealed that achiral ancillary could not control the stereoselective in the self-assembly process of the helicate, only a racemate could be obtained. Whereas, in the case of chiral BINAPO as ancillary ligands, the diastereoselectivity was realized. The mirror-image CD and CPL signs combining the NMR and semiempirical mechanical methodology proved that the chiral BINAPO could regulate the diastereoselective self-assembly of lanthanide helicate. In addition, the introduction of ancillary ligands not only offer the possibility for obtaining optical pure lanthanide helicate, but also increase the luminescence quantum yields from Eu3+ center and achieve a modest circularly polarized luminescence (CPL) with |glum| values reaching to 0.112.
Experimental
Materials and instruments
Diphenyl sulfide, ethyl trifluoroacetate, 1,10-phenanthroline, (R/S)-BINAPO and LnCl3·6H2O salts (Ln = Eu and Gd) were purchased from Chembee Chemical Reagent Co., Ltd. (China); other chemicals and solvents are of analytical reagent grade.
The NMR experiments were measured on a Bruker Avance III 400 MHz spectrometer. EI-MS and ESI-TOF-MS were recorded on Agilent 5975N and Bruker maXis mass spectrometers, respectively.
Elemental analyses were recorded on an Elementar Vario EL cube analyzer. Single crystal X-ray crystal data of Ln2L3(Phen)2 were recorded on a Xcalibur, Eos, Gemini diffractometer with Mo Kα radiation (λ = 0.71073 Å). The structure was solved by direct methods and refined on F2 by full-matrix least-squares using the SHELXTL-2018 program.26 UV-vis spectra were measured by a PerkinElmer Lambda 25 spectrometer. Photoluminescence spectra containing the emission, excitation and luminescence lifetime measurements were recorded on an Edinburgh FLS 1000 spectrophotometer, and the luminescence quantum yield (Φ) were estimated by an integrating sphere equipped on this instrument. The Φ values were calculated using the following equation:
where
Ereference is the emission spectrum of the excitation light as only the reference in the sphere,
Esample is the emission spectrum of the excitation light as only the sample in the sphere,
Lemission is the emission spectrum of the sample. The method is accurate within 10%. CD and CPL experiments were performed on an Olis DM245 spectrofluorimeter.
Synthetic procedures
4,4′-Diacetyldiphenyl sulphide. Anhydrous AlCl3 (2.15 g, 16.1 mmol) was added to 30 mL dichloromethane in a 100 mL round bottom flask and stirred for 5 min. Then acetyl chloride (1.27 g, 16.1 mmol) in 15 mL of dichloromethane was slowly added dropwise. After cooling to 0 °C with ice-bath, diphenyl sulfide (1.00 g, 5.37 mmol) in 15 mL of dichloromethane was added. After stirring overnight, the reaction solution was poured into 150 mL ice-water, then hydrolyzed with 0.5 M HCl. The crude product was extracted with CH2Cl2 for two times, the organic layer was combined and dried with anhydrous Na2SO4, then evaporated to remove the solutions in vacuo. Recrystallization the crude product from ethanol led to the white flake crystals (1.24 g, 86%). 1H NMR (400 MHz, CDCl3) δ 7.94–7.86 (m, 4H), 7.44–7.37 (m, 4H), 2.58 (s, 6H). EI-MS m/z 271.35 (M + H)+.
4,4′-Bis(4,4,4-trifluoro-1,3-dioxobutyl)diphenyl sulphide (L). L was prepared by the Claisen condensation of 4,4′-diacetyldiphenyl sulphide and ethyl trifluoroacetate in 1,2-dimethoxyethane (DME). To add freshly prepared sodium methoxide (0.80 g, 14.8 mmol) and ethyl trifluoroacetate (2.11 g, 14.8 mmol) into a Schlenk bottle containing 30 mL DME, and stirred the solution to become clear and transparent. Then 4,4′-diacetyl phenyl sulfide (1.00 g, 3.7 mmol) was added, and then solution was stirred for 24 hours at room temperature. The final reaction solution was quenched with ice-water, then acidified to pH 2–3 using 2.0 M HCl solution, the resulting yellow precipitate was filtered and recrystallized from ethyl acetate solvents to give yellow flake crystal (1.26 g, 87%). 1H NMR (400 MHz, THF-d8): 8.09–8.07 (m, 4H), 7.55–753 (m, 4H), 6.83 (s, 2H). ESI-MS m/z 549.07 [L + (CH3OH)2 + Na]+.
Synthesis of Ln2L3(Phen)2
L (1.00 g, 2.10 mmol), NEt3 (0.70 g, 7.2 mmol) and 1,10-phenanthroline (0.50 g, 2.8 mmol) were dissolved in 30 mL methanol and stirred for 5 min, then LnCl3·6H2O (1.4 mmol) in 5 mL methanol was added, which was accompanied by the presence of a large amount of precipitation. The mixture was then refluxed for 12 h. After cooling to room temperature, the precipitation was filtered and washed by 10 mL CH3OH and 10 mL H2O, respectively, then dried under vacuum.
Eu2(L)3(Phen)2. Yield: 72%. Anal. Calc. for C84H46F18N4O12S3Eu2 (2045.4026): C, 49.33; H, 2.27; N, 2.74; S, 4.70. Found: C, 49.41; H, 2.49; N, 2.71; S, 4.82. ESI-MS: m/z = 2046.0363.
Gd2(L)3(Phen)2. Yield: 82%. Anal. Calc. for C84H46F18N4O12S3Gd2 (2055.9777): C, 49.33; H, 2.27; N, 2.74; S, 4.70. Found: C, 49.41; H, 2.29; N, 2.71; S, 4.82. ESI-MS: m/z = 2056.0778.
Synthesis of Ln2(L)3 ((R/S)-BINAPO)2
L (1.00 g, 2.10 mmol), NEt3 (0.70 g, 7.2 mmol) and (R/S)-BINAPO (1.9 g, 2.8 mmol) were dissolved in 30 mL methanol and stirred for 5 min, then LnCl3·6H2O (1.4 mmol) in 5 mL methanol was added, which was accompanied by the presence of a large amount of precipitation. The mixture was then refluxed for 12 h. After cooling to room temperature, the precipitation was filtered and washed by 10 mL CH3OH and 10 mL H2O, respectively, then dried under vacuum.
Eu2L3(R-BINAPO)2. Yield: 81%. Anal. Calc. for C148H94F18O16S3P4Eu2 (2994.34): C, 59.37; H, 3.16; S, 3.21. Found: C, 59.14; H, 3.21; S, 3.47. 1H NMR (400 MHz, THF-d8) δ 9.49 (s, 8H), 9.43–9.41 (m, 4H), 8.31–8.27 (m, 12H), 8.02–7.85 (m, 24H), 7.74–7.65 (m, 8H), 7.58–7.52 (m, 4H), 7.38–7.29 (m, 12H), 7.03–6.96 (m, 4H), 6.61–6.59 (m, 12H), 5.34 (s, 6H). ESI-MS: m/z = 2995.1156 [M + H]+; 3017.1649 [M + Na]+; 3033.1338 [M + K]+.
Eu2L3(S-BINAPO)2. Yield: 82%. Anal. Calc. for C148H94F18O16S3P4Eu2 (2994.34): C, 59.37; H, 3.16; S, 3.21. Found: C, 59.26; H, 3.33; S, 3.09. 1H NMR (400 MHz, THF-d8) δ 9.49 (s, 8H), 9.43–9.41 (m, 4H), 8.31–8.27 (m, 12H), 8.02–7.85 (m, 24H), 7.74–7.65 (m, 8H), 7.58–7.52 (m, 4H), 7.38–7.29 (m, 12H), 7.03–6.96 (m, 4H), 6.61–6.59 (m, 12H), 5.34 (s, 6H). ESI-MS: m/z = 2995.1158 [M + H]+; 3017.1653 [M + Na]+; 3033.1340 [M + K]+.
Gd2L3(R-BINAPO)2. Yield: 84%. Anal. Calc. for C148H94F18O16S3P4Gd2 (3004.28): C, 59.16; H, 3.15; S, 3.20. Found: C, 59.14; H, 3.19; S, 3.22. ESI-MS: m/z = 3005.9389 [M + H]+.
Conflicts of interest
There are no conflicts to declare.
Acknowledgements
This work is financially supported by the National Natural Science Foundation of China (No. 520730805 & 51872077 & 51773054) for supporting this work.
References
-
(a) L. Zhang, H. Wang, S. Li and M. Liu, Chem. Soc. Rev., 2020, 49, 9095–9120 RSC;
(b) M. Hu, H.-T. Feng, Y.-S. Yuan, Y.-S. Zheng and B.-Z. Tang, Coord. Chem. Rev., 2020, 416, 213329–213363 CrossRef CAS;
(c) W. Ma, L. Xu, A. F. de Moura, X. Wu, H. Kuang, C. Xu and N. A. Kotov, Chem. Rev., 2017, 117, 8041–8093 CrossRef CAS PubMed;
(d) Y. Sang, J. Han, T. Zhao, P. Duan and M. Liu, Adv. Mater., 2020, 32, 1900110–1900143 CrossRef CAS PubMed;
(e) W. Shang, X. Zhu, T. Liang, C. Du, L. Hu, T. Li and M. Liu, Angew. Chem., Int. Ed., 2020, 59, 12811–12816 CrossRef CAS PubMed;
(f) L. Wang, L. Yin, W. Zhang, X. Zhu and M. Fujiki, J. Am. Chem. Soc., 2017, 139, 13218–13226 CrossRef CAS PubMed.
-
(a) S. Huo, P. Duan, T. Jiao, Q. Peng and M. Liu, Angew. Chem., Int. Ed., 2017, 56, 12174–12178 CrossRef CAS PubMed;
(b) D.-Y. Kim, J. Korean Phys. Soc., 2006, 49, S505–S508 CrossRef;
(c) F. Zinna, U. Giovanella and L. Di Bari, Adv. Mater., 2015, 27, 1791–1795 CrossRef CAS PubMed;
(d) E. J. Werner, A. Datta, C. J. Jocher and K. N. Raymond, Angew. Chem., Int. Ed., 2008, 47, 8568–8580 CrossRef CAS PubMed;
(e) T. W. Victor, K. H. O'Toole, L. M. Easthon, M. Ge, R. J. Smith, X. Huang, H. Yan, Y. S. Chu, S. Chen, D. Gursoy, M. Ralle, B. Imperiali, K. N. Allen and L. M. Miller, J. Am. Chem. Soc., 2020, 142, 2145–2149 CrossRef CAS PubMed.
- N. P. M. Huck, W. F. Jager, B. de Lange and B. L. Feringa, Science, 1996, 273, 1686–1688 CrossRef CAS.
-
(a) F. Zinna, S. Voci, L. Arrico, E. Brun, A. Homberg, L. Bouffier, T. Funaioli, J. Lacour, N. Sojic and L. Di Bari, Angew. Chem., Int. Ed., 2019, 58, 6952–6956 CrossRef CAS PubMed;
(b) M. Li, S.-H. Li, D. Zhang, M. Cai, L. Duan, M.-K. Fung and C.-F. Chen, Angew. Chem., Int. Ed., 2018, 57, 2889–2893 CrossRef CAS PubMed;
(c) J. F. Sherson, H. Krauter, R. K. Olsson, B. Julsgaard, K. Hammerer, I. Cirac and E. S. Polzik, Nature, 2006, 443, 557–560 CrossRef CAS PubMed.
-
(a) R. Sethy, J. Kumar, R. Métivier, M. Louis, K. Nakatani, N. M. T. Mecheri, A. Subhakumari, K. G. Thomas, T. Kawai and T. Nakashima, Angew. Chem., Int. Ed., 2017, 56, 15053–15057 CrossRef CAS PubMed;
(b) Y. Yang, R. C. da Costa, M. J. Fuchter and A. J. Campbell, Nat. Photonics, 2013, 7, 634–638 CrossRef CAS;
(c) Y. Imai, Y. Nakano, T. Kawai and J. Yuasa, Angew. Chem., Int. Ed., 2018, 57, 8973–8978 CrossRef CAS PubMed;
(d) S. Shuvaev, E. A. Suturina, K. Mason and D. Parker, Chem. Sci., 2018, 9, 2996–3003 RSC;
(e) K. Staszak, K. Wieszczycka, V. Marturano and B. Tylkowski, Coord. Chem. Rev., 2019, 397, 76–90 CrossRef CAS;
(f) M. Iwamura, M. Fujii, A. Yamada, H. Koike and K. Nozaki, Chem.–Asian J., 2019, 14, 561–567 CrossRef CAS PubMed;
(g) T. Wu, P. Bouř and V. Andrushchenko, Sci. Rep., 2019, 9, 1068–1073 CrossRef PubMed.
-
(a) S. V. Eliseeva and J.-C. G. Bünzli, Chem. Soc. Rev., 2010, 39, 189–227 RSC;
(b) J. C. Bünzli and C. Piguet, Chem. Soc. Rev., 2005, 34, 1048–1077 RSC;
(c) M. W. Loble, J. M. Keith, A. B. Altman, S. C. Stieber, E. R. Batista, K. S. Boland, S. D. Conradson, D. L. Clark, J. Lezama Pacheco, S. A. Kozimor, R. L. Martin, S. G. Minasian, A. C. Olson, B. L. Scott, D. K. Shuh, T. Tyliszczak, M. P. Wilkerson and R. A. Zehnder, J. Am. Chem. Soc., 2015, 137, 2506–2523 CrossRef CAS PubMed;
(d) E. G. Moore, A. P. S. Samuel and K. N. Raymond, Acc. Chem. Res., 2009, 42, 542–552 CrossRef CAS PubMed.
-
(a) S. Feuillastre, M. Pauton, L. Gao, A. Desmarchelier, A. J. Riives, D. Prim, D. Tondelier, B. Geffroy, G. Muller, G. Clavier and G. Pieters, J. Am. Chem. Soc., 2016, 138, 3990–3993 CrossRef CAS PubMed;
(b) C. M. Cruz, S. Castro-Fernández, E. Maçôas, J. M. Cuerva and A. G. Campaña, Angew. Chem., Int. Ed., 2018, 57, 14782–14786 CrossRef CAS PubMed;
(c) P. Reiné, J. Justicia, S. P. Morcillo, S. Abbate, B. Vaz, M. Ribagorda, Á. Orte, L. Álvarez de Cienfuegos, G. Longhi, A. G. Campaña, D. Miguel and J. M. Cuerva, J. Org. Chem., 2018, 83, 4455–4463 CrossRef PubMed;
(d) E. M. Sánchez-Carnerero, F. Moreno, B. L. Maroto, A. R. Agarrabeitia, M. J. Ortiz, B. G. Vo, G. Muller and S. de la Moya, J. Am. Chem. Soc., 2014, 136, 3346–3349 CrossRef PubMed;
(e) M. Deng, N. D. Schley and G. Ung, Chem. Commun., 2020, 56, 14813–14816 RSC.
-
(a) D. E. Barry, J. A. Kitchen, L. Mercs, R. D. Peacock, M. Albrecht and T. Gunnlaugsson, Dalton Trans., 2019, 48, 11317–11325 RSC;
(b) Y. Hasegawa, Y. Miura, Y. Kitagawa, S. Wada, T. Nakanishi, K. Fushimi, T. Seki, H. Ito, T. Iwasa, T. Taketsugu, M. Gon, K. Tanaka, Y. Chujo, S. Hattori, M. Karasawa and K. Ishii, Chem. Commun., 2018, 54, 10695–10697 RSC;
(c) J. Zhang, L. Dai, A. M. Webster, W. T. K. Chan, L. E. Mackenzie, R. Pal, S. L. Cobb and G.-L. Law, Angew. Chem., Int. Ed., 2021, 60, 1004–1010 CrossRef CAS PubMed;
(d) V. Y. Chang, K. U. D. Calvinho, R. C. Tovar, V. A. Johnson, D. A. Straus and G. Muller, Eur. J. Inorg. Chem., 2020, 2020, 3815–3828 CrossRef CAS;
(e) J. G. Ski, P. Starynowicz, K. T. Hua, J. L. Lunkley, G. Muller and J. Lisowski, J. Am. Chem. Soc., 2008, 130, 17761–17773 CrossRef PubMed.
-
(a) Z. Shen, Y. Sang, T. Wang, J. Jiang, Y. Meng, Y. Jiang, K. Okuro, T. Aida and M. Liu, Nat. Commun., 2019, 10, 3976–3983 CrossRef PubMed;
(b) Z.-B. Sun, J.-K. Liu, D.-F. Yuan, Z.-H. Zhao, X.-Z. Zhu, D.-H. Liu, Q. Peng and C.-H. Zhao, Angew. Chem., Int. Ed., 2019, 58, 4840–4846 CrossRef CAS;
(c) S. Lee, K. Y. Kim, S. H. Jung, J. H. Lee, M. Yamada, R. Sethy, T. Kawai and J. H. Jung, Angew. Chem., Int. Ed., 2019, 58, 18878–18882 CrossRef CAS PubMed;
(d) K. Takaishi, M. Yasui and T. Ema, J. Am. Chem. Soc., 2018, 140, 5334–5338 CrossRef CAS PubMed;
(e) K. Takaishi, K. Iwachido and T. Ema, J. Am. Chem. Soc., 2020, 142, 1774–1779 CrossRef CAS PubMed;
(f) Y. Li, A. Yagi and K. Itami, J. Am. Chem. Soc., 2020, 142, 3246–3253 CrossRef CAS PubMed.
- J. L. Lunkley, D. Shirotani, K. Yamanari, S. Kaizaki and G. Muller, J. Am. Chem. Soc., 2008, 130, 13814–13815 CrossRef CAS PubMed.
- F. Stomeo, C. Lincheneau, J. P. Leonard, J. E. O'Brien, R. D. Peacock, C. P. McCoy and T. Gunnlaugsson, J. Am. Chem. Soc., 2009, 131, 9636–9637 CrossRef CAS PubMed.
-
(a) O. Kotova, S. Comby, K. Pandurangan, F. Stomeo, J. E. O'Brien, M. Feeney, R. D. Peacock, C. P. McCoy and T. Gunnlaugsson, Dalton Trans., 2018, 47, 12308–12317 RSC;
(b) D. E. Barry, J. A. Kitchen, K. Pandurangan, A. J. Savyasachi, R. D. Peacock and T. Gunnlaugsson, Inorg. Chem., 2020, 59, 2646–2650 CrossRef CAS PubMed;
(c) S. J. Bradberry, G. Dee, O. Kotova, C. P. McCoy and T. Gunnlaugsson, Chem. Commun., 2019, 55, 1754–1757 RSC;
(d) J. A. Thomas, Dalton Trans., 2011, 40, 12005–12016 RSC.
-
(a) X.-Z. Li, L.-P. Zhou, L.-L. Yan, D.-Q. Yuan, C.-S. Lin and Q.-F. Sun, J. Am. Chem. Soc., 2017, 139, 8237–8244 CrossRef CAS PubMed;
(b) L.-X. Cai, L.-L. Yan, S.-C. Li, L.-P. Zhou and Q.-F. Sun, Dalton Trans., 2018, 47, 14204–14210 RSC;
(c) Q.-Y. Zhu, L.-P. Zhou, L.-X. Cai, X.-Z. Li, J. Zhou and Q.-F. Sun, Chem. Commun., 2020, 56, 2861–2864 RSC.
- C. T. Yeung, W. T. Chan, S. C. Yan, K. L. Yu, K. H. Yim, W. T. Wong and G.-L. Law, Chem. Commun., 2015, 51, 592–595 RSC.
-
(a) Y. B. Tan, M. Yamada, S. Katao, Y. Nishikawa, F. Asanoma, J. Yuasa and T. Kawai, Inorg. Chem., 2020, 59, 12867–12875 CrossRef CAS PubMed;
(b) Y. Kitagawa, M. Tsurui and Y. Hasegawa, ACS Omega, 2020, 5, 3786–3791 CrossRef CAS PubMed;
(c) C. Dee, F. Zinna, E. Kreidt, L. Arrico, A. Rodríguez-Rodríguez, C. Platas-Iglesias, L. Di Bari and M. Seitz, J. Rare Earths, 2020, 38, 564–570 CrossRef CAS;
(d) L. Arrico, C. De Rosa, L. Di Bari, A. Melchior and F. Piccinelli, Inorg. Chem., 2020, 59, 5050–5062 CrossRef CAS PubMed;
(e) F. Zinna, L. Arrico and L. Di Bari, Chem. Commun., 2019, 55, 6607–6609 RSC;
(f) M. Starck, L. E. MacKenzie, A. S. Batsanov, D. Parker and R. Pal, Chem. Commun., 2019, 55, 14115–14118 RSC;
(g) Y. B. Tan, Y. Okayasu, S. Katao, Y. Nishikawa, F. Asanoma, M. Yamada, J. Yuasa and T. Kawai, J. Am. Chem. Soc., 2020, 142, 17653–17661 CrossRef CAS PubMed;
(h) T. Y. Bing, T. Kawai and J. Yuasa, J. Am. Chem. Soc., 2018, 140, 3683–3689 CrossRef CAS PubMed.
-
(a) Y. Zhang, Y. Zhou, T. Gao, P. Yan and H. Li, Chem. Commun., 2020, 56, 13213–13216 RSC;
(b) Z. Zhang, Y. Zhou, H. Li, T. Gao and P. Yan, Dalton Trans., 2019, 48, 4026–4034 RSC.
-
(a) Y. Zhou, H. Li, T. Zhu, T. Gao and P. Yan, J. Am. Chem. Soc., 2019, 141, 19634–19643 CrossRef CAS PubMed;
(b) G. Han, Y. Zhou, Y. Yao, Z. Cheng, T. Gao, H. Li and P. Yan, Dalton Trans., 2020, 49, 3312–3320 RSC;
(c) Y. Zhou, Y. Yao, Z. Cheng, T. Gao, H. Li and P. Yan, Inorg. Chem., 2020, 59, 12850–12857 CrossRef CAS;
(d) M. Dubey, A. Kumar, V. M. Dhavale, S. Kurungot and D. S. Pandey, CrystEngComm, 2015, 17, 8202–8206 RSC;
(e) S. Bi, Y. Zhou, Y. Yao, Z. Cheng, T. Gao, P. Yan and H. Li, Aust. J. Chem., 2020, 74, 145–150 CrossRef.
-
(a) D. Liu, Y. Zhou, Y. Zhang, H. Li, P. Chen, W. Sun, T. Gao and P. Yan, Inorg. Chem., 2018, 57, 8332–8337 CrossRef CAS PubMed;
(b) K. T. Hua, J. Xu, E. E. Quiroz, S. Lopez, A. J. Ingram, V. A. Johnson, A. R. Tisch, A. de Bettencourt-Dias, D. A. Straus and G. Muller, Inorg. Chem., 2012, 51, 647–660 CrossRef CAS;
(c) M. Leonzio, M. Bettinelli, L. Arrico, M. Monari, L. Di Bari and F. Piccinelli, Inorg. Chem., 2018, 57, 10257–10264 CrossRef CAS PubMed;
(d) M. Gorecki, L. Carpita, L. Arrico, F. Zinna and L. Di Bari, Dalton Trans., 2018, 47, 7166–7177 RSC;
(e) J. Yuasa, H. Ueno and T. Kawai, Chem, 2014, 20, 8621–8627 CrossRef CAS PubMed;
(f) T. Harada, Y. Nakano, M. Fujiki, M. Naito, T. Kawai and Y. Hasegawa, Inorg. Chem., 2009, 48, 11242–11250 CrossRef CAS PubMed.
- J. D. L. Dutra, T. D. Bispo and R. O. Freire, J. Comput. Chem., 2014, 35, 772–775 CrossRef CAS PubMed.
- T. Zhu, P. Chen, H. Li, W. Sun, T. Gao and P. Yan, Phys. Chem. Chem. Phys., 2015, 17, 16136–16144 RSC.
- C. Görller-Walrand and K. Binnemans, Handbook on the Physics and Chemistry of Rare Earths, Elsevier, Amsterdam, 1996, pp. 121–283 Search PubMed.
- D. Shirotani, T. Suzuki and S. Kaizaki, Inorg. Chem., 2006, 45, 6111–6113 CrossRef CAS PubMed.
-
(a) F. J. Steemers, W. Verboom, D. N. Reinhoudt, E. B. van der Tol and J. W. Verhoeven, J. Am. Chem. Soc., 1995, 117, 9408–9414 CrossRef CAS;
(b) M. Latva, H. Takalo, V.-M. Mukkala, C. Matachescu, J. C. Rodriguez-Ubis and J. Kankare, J. Lumin., 1997, 75, 149–169 CrossRef CAS.
-
(a) S. D. Bonsall, M. Houcheime, D. A. Straus and G. Muller, Chem. Commun., 2007, 35, 3676–3678 RSC;
(b) S. Petoud, G. Muller, E. G. Moore, J. Xu, J. Sokolnicki, J. P. Riehl, U. N. Le, S. M. Cohen and K. N. Raymond, J. Am. Chem. Soc., 2007, 129, 77–83 CrossRef CAS PubMed.
-
(a) M. Seitz, K. Do, A. J. Ingram, E. G. Moore, G. Muller and K. N. Raymond, Inorg. Chem., 2009, 48, 8469–8479 CrossRef CAS;
(b) K. Okutani, K. Nozaki and M. Iwamura, Inorg. Chem., 2014, 53, 5527–5537 CrossRef CAS PubMed;
(c) M. Leonzio, A. Melchior, G. Faura, M. Tolazzi, F. Zinna, L. Di Bari and F. Piccinelli, Inorg. Chem., 2017, 56, 4413–4421 CrossRef CAS PubMed;
(d) O. Kotova, S. Blasco, B. Twamley, J. O'Brien, R. D. Peacock, J. A. Kitchen, M. Martínez-Calvo and T. Gunnlaugsson, Chem. Sci., 2015, 6, 457–471 RSC;
(e) C.-T. Yeung, K.-H. Yim, H.-Y. Wong, R. Pal, W.-S. Lo, S.-C. Yan, M. Yee-Man Wong, D. Yufit, D. E. Smiles, L. J. McCormick, S. J. Teat, D. K. Shuh, W.-T. Wong and G.-L. Law, Nat. Commun., 2017, 8, 1128–1137 CrossRef PubMed;
(f) N. Shi, R. Wang, X. Wang, J. Tan, Y. Guan, Z. Li, X. Wan and J. Zhang, Chem. Commun., 2019, 55, 1136–1139 RSC.
- G. M. Sheldrick, Acta Crystallogr., Sect. C: Struct. Chem., 2015, 71, 3–8 Search PubMed.
Footnote |
† Electronic supplementary information (ESI) available. CCDC 994501. For ESI and crystallographic data in CIF or other electronic format see DOI: 10.1039/d1ra01583d |
|
This journal is © The Royal Society of Chemistry 2021 |
Click here to see how this site uses Cookies. View our privacy policy here.