DOI:
10.1039/D1RA01140E
(Paper)
RSC Adv., 2021,
11, 14399-14407
Unique hierarchical SiO2@ZnIn2S4 marigold flower like nanoheterostructure for solar hydrogen production†
Received
10th February 2021
, Accepted 10th April 2021
First published on 16th April 2021
Abstract
The novel marigold flower like SiO2@ZnIn2S4 nano-heterostructure was fabricated using an in situ hydrothermal method. The nanoheterostructure exhibits hexagonal structure with marigold flower like morphology. The porous marigold flower assembly was constructed using ultrathin nanosheets. Interestingly, the thickness of the nanopetal was observed to be 5–10 nm and tiny SiO2 nanoparticles (5–7 nm) are decorated on the surface of the nanopetals. As the concentration of SiO2 increases the deposition of SiO2 nanoparticles on ZnIn2S4 nanopetals increases in the form of clusters. The optical study revealed that the band gap lies in the visible range of the solar spectrum. Using X-ray photoelectron spectroscopy (XPS), the chemical structure and valence states of the as-synthesized SiO2@ZnIn2S4 nano-heterostructure were confirmed. The photocatalytic activities of the hierarchical SiO2@ZnIn2S4 nano-heterostructure for hydrogen evolution from H2S under natural sunlight have been investigated with regard to the band structure in the visible region. The 0.75% SiO2@ZnIn2S4 showed a higher photocatalytic activity (6730 μmol−1 h−1 g−1) for hydrogen production which is almost double that of pristine ZnIn2S4. Similarly, the hydrogen production from water splitting was observed to be 730 μmol−1 h−1 g−1. The enhanced photocatalytic activity is attributed to the inhibition of charge carrier separation owing to the hierarchical morphology, heterojunction and crystallinity of the SiO2@ZnIn2S4.
1 Introduction
In recent years, the combined extreme energy shortages and environmental degradation have been triggered by technological development that demands a huge amount of fossil oil. Therefore, intensive studies focus on investigating alternative energy resources with renewable and environmentally-friendly advantages.1,2 Among them, hydrogen will be considered a possible candidate for future use in conventional fuels.3 Photocatalytic H2S & water splitting is one of the most promising approaches for hydrogen production, as it can perform a conversion that converts solar light into chemical energy.4–6 Various materials with important photocatalytic hydrogen evolution properties, such as MoS2, BiVO4, CuO, Ti3C2 etc., have been prepared to date.7–10 Ternary ZnIn2S4 is a semiconductor chalcogenide with great visible-light-responsive photocatalytic activity, considerable chemical stability and ample flat band potential which attracted considerable interest in lithium ion batteries and gas sensor applications.11–15
As one of the most promising n-type semiconductors, ZnIn2S4 has captured much interest in the field of photocatalytic hydrogen generation, which can be attributed to its non-toxic properties and suitable band structure (2.2–2.5 eV)16,17 Similar to other photocatalysts, ZnIn2S4 suffers electron–hole recombination, slow oxygen evolution reaction kinetics and low visible light utilization.18 Combining ZnIn2S4 with other semiconductors is an effective method to inhibit the recombination of photogenerated charge carriers and improve the photocatalytic activity. Until now, many ZnIn2S4 nanoheterostructures and doped ZnIn2S4 with various morphologies such as ZnIn2S4/Ni12P5,19 g-C3N4/Ag:ZnIn2S4,20 SnS@ZnIn2S4,21 Ag-doped ZnIn2S4,22 CdS/QDs/ZnIn2S4,23 Ti3C2 MXene@TiO2/ZnIn2S4 (ref. 24) have been reported to exhibit admirable visible-light photocatalytic activity. These literatures emphasized that while some prodigious work has been done in the field of photocatalysis, catalytic efficiency is still insufficient. It also needs more studies to explore new ways of further improving it.
SiO2 is an insulator mesoporous material. SiO2 has been widely used in the fields of catalysis, separation and adsorption due to its large specific surface area, abundant surface hydroxyl groups, environmental friendliness, stable chemical properties, uniform pore size and relatively high mechanical strength.25–27 SiO2 is mainly used in many heterostructures to enhance the photocatalytic performance of other semiconductor by increasing the surface area of the catalyst and it affects on the charge carrier recombination.28,29 In past, various attempts have been made to enhance the photocatalytic activity of individual semiconductors by coupling with SiO2 such as g-C3N4/SiO2,30 SiO2@TiO2core@Shell,31 SiO2/CNOs/TiO2,32 gC3N4/TiO2/Fe3O4@SiO2,33 γ-Fe2O3@SiO2@TiO2,34 TiO2–SiO2Ag,35 NiO/SiO2,36 ZnFe2O4@SiO2@TiO2.37 So, to overcome the problem of fast recombination of photoinduced electron–hole pair and enhance the photocatalytic performance of pure ZnIn2S4, we synthesized a ZnIn2S4 and decorated with SiO2 nanoparticles to enhance the separation and transfer efficiency of electrons. With this motivation, we have prepared a new SiO2@ZnIn2S4 hybrid semiconductor by coupling SiO2 and ZnIn2S4. To the best of our knowledge, this is the first report on SiO2@ZnIn2S4 heterostructure for H2 production from H2S & Water splitting.
In the view of above findings, we report the hierarchical SiO2@ZnIn2S4 nano-heterostructure for efficient generation of hydrogen, which was synthesized using facile hydrothermal method. These fabricated heterostructures were thoroughly characterized using XRD, FESEM, UV-Vis spectroscopy, PL and TEM. The fabrication of SiO2@ZnIn2S4 nano-heterostructure increases the charge separation and also enhances the solar light response. Thus, under solar light irradiation, the high output rate of hydrogen is observed. In addition, the component effect on photocatalytic efficiency of SiO2@ZnIn2S4 nano-heterostructure and the proposed photocatalytic mechanism have been further investigated.
2 Experimental sections
Zinc nitrate hexahydrate (Zn(NO3)2·6H2O), Indium nitrate (In(NO3)3·4H2O), Thiourea (CSNH2CS),Silicon Dioxide (SiO2) used for the preparation of catalysts are of analytical grade (SD-fine-chem limited, India) and used without any further purification.
2.1 Synthesis of ZnIn2S4 and SiO2@ZnIn2S4
All the chemicals used for SiO2@ZnIn2S4 synthesis were analytical grade and used without further purification. The hydrothermal method was used to synthesise SiO2@ZnIn2S4 photocatalysts and the procedure is briefly defined as follows: first, 1 mole of hexahydrate zinc nitrate (Zn(NO3)2·6H2O) (99.99%) and 2 moles of indium nitrate (In(NO3)3·4H2O) (99.99%) and 8 moles of thiourea were mixed in a beaker (a) in 70 mL distilled water and stirred at room temperature for 15 minutes. After stirring, 0.25 wt% of silicon dioxide was applied to the above solution in beaker (a). The clear solution was then moved to a Teflon autoclave with a capacity of 150 mL and held in an electric oven at 150 °C for 30 hours. As the synthetic catalyst was washed several times with absolute ethanol and deionized water, the yellow powder was obtained. For pure ZnIn2S4, 0.25%SiO2@ZnIn2S4, 0.50%SiO2@ZnIn2S4, 0.75%SiO2@ZnIn2S4, 1% SiO2@ZnIn2S4 were labelled as (ZS-1), (ZS-2), (ZS-3), (ZS-4) and as (ZS-5) respectively and were subsequently used for further analysis and comparison.
2.2 Photocatalytic study
2.2.1 Photocatalytic hydrogen generation from water. The photochemical reaction was carried in a 70 mL total volume air-tight cylindrical quartz reactor with a cooling jacket for water circulation. All the reactions were carried out at ambient conditions under natural sunlight on sunny days (March to May months) between 10 am to 3 pm at Pune, located in the Maharashtra state of India. The intensity of solar light was measured by using a digital Lux meter. The measured average intensity of solar light reaching the surface of the earth was 145
000 Lux. In a typical photocatalytic experiment, 15 mg of the photocatalyst was dispersed in 25 mL total volume containing 20% methanol (v/v) in aqueous solution. The 45 mL free space of photoreactor made airtight with rubber septum followed by ultrasonication for 5 min for the uniform dispersion of catalyst. The solution mixture was then purged with Ultra High Purity nitrogen gas (UHP-99.999%) to remove all the gases in the headspace of the reactor and dissolved oxygen from the reaction mixture. Before and after irradiation with solar light, the gas in the free space of the reactor was analyzed using gas chromatography (GC). The generated gas was analyzed immediately using GC with a specific time interval.
2.2.2 Photocatalytic hydrogen generation from water. The hydrogen generation is performed in a cylindrical quartz photochemical thermostatic reactor introducing 0.5 g SiO2@ZnIn2S4. The reactor was filled with 700 mL 0.5 M aqueous KOH and purged with Argon for 30 min. Hydrogen sulfide is bubbled through the solution for 1 h at the rate of 2.5 mL min−1 at 299 K. SiO2@ZnIn2S4 photocatalyst is introduced as a suspension into a cylindrical quartz reactor and irradiated with the sunlight at a constant stirring with a continuous flow of H2S (2.5 mL min−1). The excess of H2S was trapped in 10% NaOH solution. The amount of H2 gas evolved was measured using a graduated gas burette and analyzed using gas chromatography technique (GC) equipped with thermal conductivity detector (TCD) and Porapak-Q packed column with N2 as a carrier gas.
2.3 Samples characterization
The phase formation and crystallite size of all synthesized samples were estimated via X-ray Diffractometry (XRD-D8, Advance, Bruker-AXS) with Ni-filtered Cu-Kα radiation (λ = 1.5418 Å). Optical properties of the bare and Au loaded samples were studied by UV-Vis-DRS spectrophotometer (UV 2600 spectrometer, Lambda-950, Perkin-Elmer) in the spectral range of 200–800 nm. The surface morphology was characterized using Field Emission Scanning Electron Microscopy (FESEM; Hitachi, S-4800 II) and Field Emission Transmission Electron Microscopy (FETEM; JEM-2000 FS). Image processing and interplanar distance (d) evaluation were performed with the help of micrograph Gatan software. Surface characterization of all SiO2@ZnIn2S4 samples was carried out using X-ray Photoelectron Spectroscopy (XPS, ESCA3000, VG Scientific Ltd.) at a pressure of >1 × 10–9 Torr. The general scan C1s, S2p, In3d, Sn3d, and Zn2p core level spectra were recorded with non-mono chromatized Mg-Kα radiation (photon energy-1253.6 eV). Baseline correction and peak fitting for all the samples were done using software package XPS peak-41. The core level binding energies (BEs) were aligned with respect to the C1s binding energy of 285 eV. The collected gas sample was analyzed using a Gas chromatography system (Shimadzu GC-2014) with portapak-Q packed column coupled with TCD detector and nitrogen (N2-UHP) as a carrier gas.
3 Results and discussions
3.1 Structural study
The phase purity and crystallinity of the as-synthesized pristine ZnIn2S4 and SiO2@ZnIn2S4 nano-heterostructure were investigated by using X-ray diffraction analysis. Fig. 1 depicts the XRD diffractogram of the as-synthesized samples (ZS1–ZS5). The pristine ZnIn2S4 (ZS1) shows diffraction peaks at 27.93°, 47.57°, 33.62°, 44.44°, 29.14° and 56.89° are indexed to crystalline planes (311), (440), (400), (511), (222), and (533), respectively. The diffraction peaks reveal presence of cubic phase of ZnIn2S4 (JCPDS 00-023-0295).38 For the SiO2@ZnIn2S4 nano-heterostructures, it has the similar XRD patterns like pristine ZnIn2S4 and the diffraction peaks corresponding to SiO2 are not observed because very small amount of SiO2 (i.e. less than equal to 1%).This also could be because of nano tiny SiO2 surface is covered with dense nano-petals of ZnIn2S4, which may prevent XRD from detecting the inner SiO2. Additionally, the peak intensity of ZnIn2S4 slightly decreased with the increase of SiO2 content. The crystalite size is obtained for nanoheterostructure is around 15 nm against bare ZnIn2S4 i.e. 13.65 nm. The lattice parameter of SiO2@ZnIn2S4 is a = b = c 10.78 Å having cubic system (space group Fd3m & space group number 227). The XRD findings may suggest the coexistence of SiO2 and ZnIn2S4 in the nano-heterostructures of SiO2@ZnIn2S4, which will be further illustrated by the following observations from XPS, FESEM and TEM. No diffraction peaks were obtained for any impurities, which indicate the pure nature of the SiO2@ZnIn2S4 samples as-synthesised.
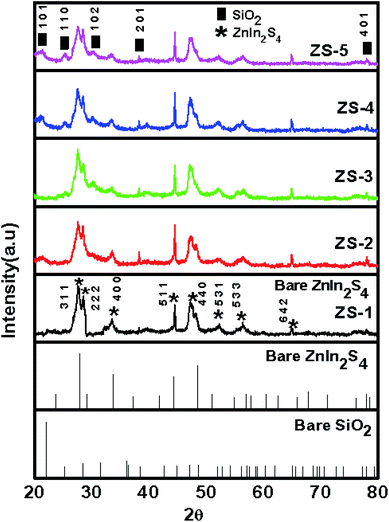 |
| Fig. 1 X-ray diffraction patterns of (ZS1) pure ZnIn2S4 (ZS2) 0.25% SiO2@ZnIn2S4 (ZS3) 0.50% SiO2@ZnIn2S4 (ZS4) 0.75% SiO2@ZnIn2S4 and (ZS5) 1% SiO2@ZnIn2S4 sample Photocatalyst. | |
3.2 Surface and morphological studies
The morphological investigation of as-synthesized SiO2@ZnIn2S4 nano-heterostructures was observed by using field emission scanning electron microscopy (FESEM). Fig. 2 shows FESEM images of as-synthesized pristine ZnIn2S4 (ZS1) and SiO2@ZnIn2S4 (ZS2–ZS5) nano-heterostructures. The low magnification image (Fig. 2a) of pristine ZnIn2S4 demonstrates the ultrathin curled nanosheets which self-assembled in the form of marigold flower. The average diameter of flower was found to be 7–12 um. The high magnification image (Fig. 2b) indicate that these flower are assembled by ultrathin interlocked nanosheets (nanopetal) with the thickness about 5–10 nm. The FESEM images of SiO2@ZnIn2S4 nano-heterostructures (ZS-2 to ZS-5) as shown in Figure (2c to j). From figures, the effect of addition of SiO2 on surface morphologies of nano-heterostructures was investigated.
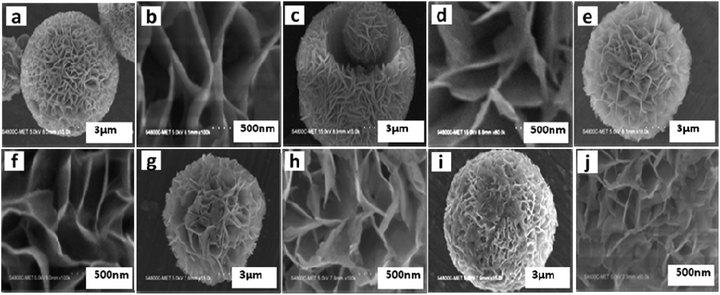 |
| Fig. 2 Representative FE-SEM images of (a and b) pure ZnIn2S4 (c and d) 0.25% SiO2@ZnIn2S4 (e and f) 0.50% SiO2@ZnIn2S4 (g and h) 0.75% SiO2@ZnIn2S4 and (i and j) 1% SiO2@ZnIn2S4 sample photocatalyst. | |
Fig. 2c–j depicts FESEM imagos of as-synthesized nano-heterostructures (ZS-2 to ZS-5), where the flowers like morphology was retained. Most significantly, extremely crystalline ultrathin nanosheets are seen in case of ZS-4 (Fig. 2g and h). This shows the fully grown ultrathin nanosheets curled and form nanoflowers. Furthermore, as percentage of SiO2 increases (ZS-5), the small SiO2 nanoparticles deposited on nanopetals of flower & ultrathin nanosheets (nanopetals) get distorted (Fig. 2i and j). As the concentration of SiO2 increases there is a deposition of the SiO2 particles on the nanopetals of ZnIn2S4. Further, increasing concentration, there is a formation of SiO2 clusters on nanopetal of the flowers. We observed uniform deposition/coating for sample ZS-4 (Deposition of 0.75% SiO2).
The microstructural aspects of as-synthesized SiO2@ZnIn2S4 (ZS-4) nano-heterostructure were confirmed by field emission transmittance electron microscopy (FETEM). Fig. 3 depicts the TEM images of SiO2@ZnIn2S4. The TEM images of SiO2@ZnIn2S4 clearly shows flower-like structure formed by ultrathin nanosheets (nanopetals). These results are well in accordance with FESEM (Fig. 2). More specific details about the nano-heterostructure of SiO2@ZnIn2S4 was further examined by HRTEM, as shown in Fig. 3c. The lattice fringes are distinctly identified with interplanar distances of 0.312 nm and 0.4 nm, which correlates to the d spacing of (311) and (101) planes of ZnIn2S4 and SiO2, respectively.39,40 Fig. 3d demonstrate the selected area diffraction pattern of as-synthesized sample, which clearly shows polycrystalline nature of SiO2@ZnIn2S4 nano-heterostructure. From Fig. 3e, the observed diffraction dots can be assigned to (311) and (101) planes of ZnIn2S4 and SiO2, respectively. These results are consistent with XRD results. To investigate the distribution of SiO2 in SiO2@ZnIn2S4 nano-heterostructure the EDS analysis was performed (Fig. 3e and f). These results clearly indicate the uniform distribution of Si, Zn, In, S, & O in SiO2@ZnIn2S4 nano-heterostructure. More significantly, the TEM results confirm the successful formation of SiO2@ZnIn2S4 nano-heterostructure and there exists intimate contact between SiO2 and ZnIn2S4.
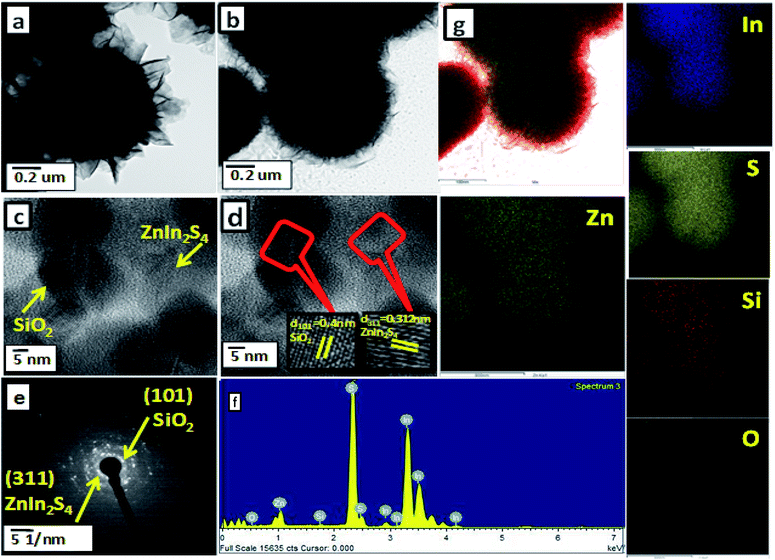 |
| Fig. 3 (a) FE-TEM images of pure ZnIn2S4, (b) FE-TEM images of SiO2@ZnIn2S4, (c) HRTEM (d) SAED pattern (e) EDAX spectra and (f) elemental mapping of 2% SiO2 loaded ZnIn2S4. | |
We can easily predict the mechanism of the flower-like structure of SiO2@ZnIn2S4 based on morphological studies. Initially, the nucleation begins to form the Zn2+, In3+ and S2− ions with the dissociation of the sulphur source, zinc and indium nitrate precursor at a low temperature. The degree of dissociation of zinc and indium salts is greater in the water-like polar solvent. In the supersaturated solution, the petite nuclei were formed and there was further growth of nanoparticles over a time (Scheme 1). In order to minimize their surface energy that further evolves anisotropically along with 2D directions, these new nanoparticles impulsively agglomerated, aligned and confer the formation of ultra-thin nanosheets (Nanopetals). And then after prolonged hydrothermal reaction the curled nanopetals are formed because of surface tension created on the petals. Further, this curving nature petals self-assembled and form marigold like flower structure. During reaction the disperse SiO2 nanoparticles gets deposited on the surface of the nanopetals.
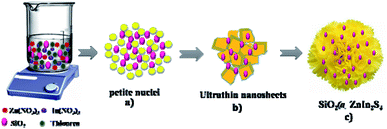 |
| Scheme 1 Schematic representation of the formation mechanism of SiO2@ZnIn2S4 nanoheterostructure. | |
3.3 Optical properties
The optical absorption study of the pristine ZnIn2S4 and SiO2@ZnIn2S4 nano-heterostructures is important for its photocatalytic application. The UV-Vis diffuse reflectance spectra of as-synthesized nano-heterostructures (ZS-1 to ZS-5) are shown in the Fig. 4. The ZnIn2S4 shows an absorption edge of about 570 nm and its band gap is estimated to be 2.16 eV.41 Compared to the pristine ZnIn2S4, the all SiO2@ZnIn2S4 nano-heterostructures exhibited a small red shift in UV-vis diffuse reflectance absorption spectrum (DRS) and the visible light absorption intensity was also increased due to SiO2 nanoparticles decoration. The addition of SiO2 will greatly improve light harvesting, separation and transfer of photoinduced charge carriers (h+/e−) and can be shown by the following spectrum of photoluminescence. Thus, SiO2@ZnIn2S4 nano-heterostructures demonstrated promising usage as a photocatalyst under visible light irradiation relative to pristine ZnIn2S4.
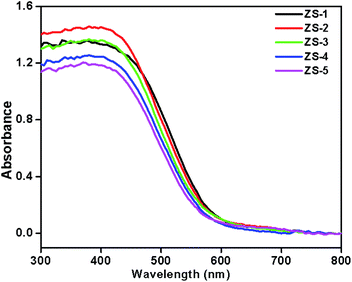 |
| Fig. 4 UV-DRS spectra of (ZS1) pure ZnIn2S4 (ZS2) 0.25% SiO2@ZnIn2S4 (ZS3) 0.50% SiO2@ZnIn2S4 (ZS4) 0.75% SiO2@ZnIn2S4 and (ZS5) 1% SiO2@ZnIn2S4 sample photocatalyst. | |
Photoluminescence (PL) emission spectrum measurement was performed for pristine ZnIn2S4 (ZS1) and SiO2@ZnIn2S4 (ZS2–ZS5) nano-heterostructures (Fig. 5). This spectrum can provide useful information on capture and recombination charge carriers in semiconductor. The ZS-1 PL spectrum displays a broad peak ∼572 nm, attributing to the band gap transfer emission with the light energy nearly equivalent to the ZnIn2S4 band gap energy.42 For SiO2@ZnIn2S4 Samples, their PL spectra are similar to that of pristine ZnIn2S4, but after SiO2 modification a significant decrease in PL intensity was observed. Such obvious reduction in PL intensity strength revealed the suppression in recombination rate of photogenerated e− & h+ in the SiO2@ZnIn2S4. Interestingly, ZS-4 has shown lowest PL Intensity which indicates optimum concentration of SiO2 can inhibit electron hole recombination strongly. At very high concentration of SiO2 i.e. 1% the transport of electrons get hindered which ultimately increased the electron hole recombination. However, at moderate SiO2 concentration i.e. 0.75% to electron transport to the surface is quite high. Therefore, we conclude that of the ZS-4 sample has more efficient charge carrier separation compared to other samples.43
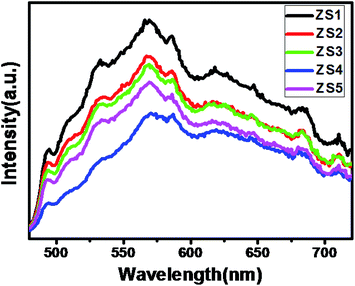 |
| Fig. 5 Photoluminescence spectra of (ZS1) pure ZnIn2S4 (ZS2) 0.25% SiO2@ZnIn2S4 (ZS3) 0.50% SiO2@ZnIn2S4 (ZS4) 0.75% SiO2@ZnIn2S4 and (ZS5) 1% SiO2@ZnIn2S4 sample photocatalyst. | |
3.4 XPS study
The SiO2@ZnIn2S4 XPS spectra are analyzed in a contrastive manner in order to further confirm the formation of the SiO2@ZnIn2S4 nano-heterostructure and determine its elemental composition and valence states. The Zn 2p, In 3d, S 2p, Si 2P, and O 1 s high-resolution XPS spectra provides more detailed information on the chemical state of these elements in Fig. 6a–e respectively. Fig. 6a shows the Zn 2p region in SiO2@ZnIn2S4 nano-heterostructure with high resolution. Based on figure, 2p1/2 and 2p3/2 splitting respectively defined the doublet of Zn 2p. The binding energies at 1046.02 eV associated with Zn2+ p1/2, whereas those of 1021.24 eV were correlated with Zn2+ p3/2.44 Fig. 6b shows the In 3d high-resolution spectrum and the SiO2@ZnIn2S4 nano-heterostructure peak at 453.54 eV and 445.18 eV corresponds to In3+ 3d3/2 and In3+ 3d5/2 respectively.21 Furthermore, the XPS signal (Fig. 6c) observed at a 162.89 eV binding energy is attributed to S2− 2p3/2 which is inconsistent with ZnIn2S4 results.45 Similarly, Fig. 6d and e shows the high resolution spectrum of the energy levels of Si 2P and O 1 s. The binding energies located at 102.1 eV and 532.3 eV verified the existence of Si & O element in SiO2@ZnIn2S4 nano-heterostructure.46 On the basis of the above results, it indicates that SiO2 nanoparticle are distributed uniformly on ZnIn2S4. Together with the TEM and optical results, these results suggest that ZnIn2S4 was hybridized with SiO2 by the successful formation of nano-heterostructure, which provides synergistic interaction between SiO2 and ZnIn2S4 matrices for the transfer of charge carriers.
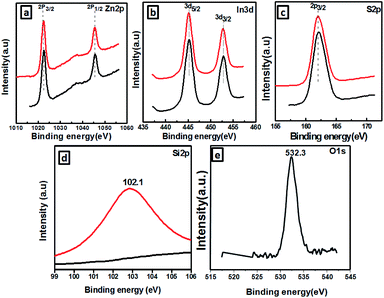 |
| Fig. 6 XPS spectra of the as-prepared SiO2@ZnIn2S4 (a) Zn2p, (b) In3d, (c) S2p, (d) Si3d. | |
3.5 Photocatalytic study
3.5.1 Photocatalytic activity measurement. As conferred earlier, SiO2@ZnIn2S4 is a good semiconductor having a narrow band gap falling in the visible region at room temperature. Considering the good response towards solar light, photo-catalytic activities of SiO2@ZnIn2S4 have been investigated. Herein, we report the photocatalytic H2 evolution performance from water and H2S under natural sun light.
3.5.2 Photocatalytic H2 evolution from H2O. The effect of SiO2 loading on ZnIn2S4 and their photo-catalytic activity of hydrogen generation were studied under natural sunlight irradiation using Na2S/Na2S2O3 as a scavenger electron donor. A series of experiments were performed using different concentrations of SiO2 on the surface of ZnIn2S4, and their results are given in Table 1. The investigation results indicated that the maximum hydrogen generation, i.e. 796 μmol h−1 g−1, was obtained for the sample ZS-4, whereas pure ZnIn2S4, shows hydrogen evolution rates of 138 μmol h−1 g−1. Furthermore, 0.25% SiO2@ZnIn2S4, 0.50% SiO2@ZnIn2S4 & 1% SiO2@ZnIn2S4 give 237, 480 & 643 μmol h−1 g−1 respectively from water splitting. Fig. 7 shows the time-dependent hydrogen generation using the as-synthesized ZnIn2S4 and SiO2@ZnIn2S4 nanoheterostructures. The linearity of the graph shows a continuous and stable hydrogen generation rate. Which reveals that most exciting electrons are available for proton reduction. For this experiment, we used 70 mL DI water and Na2S/Na2S2O3 mixture as a sacrificial agent. In the present case, 0.25 M Na2S and 0.35 M Na2S2O3 mixture were used to resist the photo-corrosion of the semiconductor. Moreover, the Na2S solution acts as a hole scavenger, and it oxidized S2− into S2−, which is beneficial for hydrogen generation from SiO2@ZnIn2S4 rather than alcohol. The photocatalytic hydrogen generation mechanism from water is well reported and discussed in the previous literature.47 The Semiconductor photocatalyst after interaction with solar light with energy greater than or equal to band gap energy generates electrons in CB and holes in VB. It causes redox reactions of adsorbed species on a semiconducting material. The photogenerated holes from VB irreversibly oxidize S2−, which was reduced back to S2− by Na2S2O3 and radially got adsorbed on the surface of semiconductor producing protons (H+) and free radicals, while electrons from CB reduce H+ ions into molecular hydrogen. |
 | (1) |
Table 1 The H2 generation rates for as-synthesized ZnIn2S4, SiO2 & SiO2@ZnIn2S4
Sr. no. |
Sample code |
H2 evolution rate from water (μmol h−1 g−1) |
H2 evolution rate from H2S (μmol h−1 g−1) |
1 |
ZS1 |
138 |
3350 |
2 |
ZS2 |
237 |
4320 |
3 |
ZS3 |
480 |
5650 |
4 |
ZS4 |
796 |
6730 |
5 |
ZS5 |
643 |
6050 |
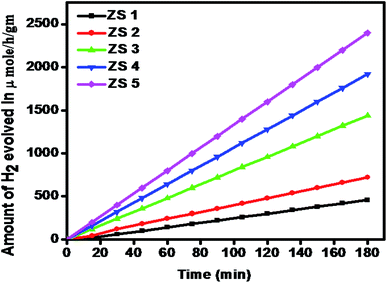 |
| Fig. 7 Time versus volume of H2 (μmole) evolution from H2O of the all samples synthesized at (ZS-1) pure ZnIn2S4 and SiO2@ZnIn2S4 nanoheterostructure prepared with a different percent of SiO2 loading (ZS-2) 0.25%, (ZS-3) 0.50%, (ZS-4) 0.75%, and (ZS-5) 1%. | |
Oxidation:
|
SO32−+ 2OH− + 2h+ → SO42− + 2H+
| (2) |
|
S22− + SO32− → S2O32− + S2−
| (4) |
|
SO32− + S2− + 2h+ → S2O32−
| (5) |
Reduction:
3.7.1 Photocatalytic H2 evolution from H2S splitting. The photocatalytic activity of the as-synthesized pristine ZnIn2S4 and SiO2@ZnIn2S4 nano-heterostructure is evaluated for hydrogen production via. H2S splitting under natural sunlight. Fig. 8 and Table 1 shows amount of hydrogen evolved via. H2S splitting using ZS-1 to ZS-5 samples. The graph linearity clearly shows stable evolution rate of hydrogen. Control experiments indicated that no evolution of hydrogen was detected in the absence of photocatalyst.
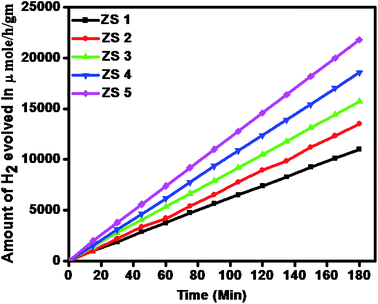 |
| Fig. 8 Time versus volume of H2 (μmole) evolution from H2S of the all samples (ZS-1) pure ZnIn2S4 and SiO2@ZnIn2S4 nanoheterostructure prepared with a different percent of SiO2 loading (ZS-2) 0.25%, (ZS-3) 0.50%, (ZS-4) 0.75%, and (ZS-5) 1%. | |
From Fig. 8, it is observed that pristine ZnIn2S4 exhibits hydrogen production rate of 3350 μmol h−1 g−1, The enhanced photocatalytic H2 generation can be attributed to the hydrothermal assisted growth of flower-like ZnIn2S4 assembled from ultrathin nanosheets (nanopetals) which facilitates more catalyst surface for reaction and light harvesting. The photocatalytic hydrogen production activity of SiO2@ZnIn2S4 nano-heterostructure increases with increase in SiO2 content. The sample ZS-2 exhibits significant H2 production rate 4320 μmol h−1 g−1, which is 30% higher compared to the ZS1 sample. Amongst all of the photocatalysts (ZS1–ZS5), 0.75 wt% loaded SiO2@ZnIn2S4 nano-heterostructure (ZS-4) showed the highest rate for H2 production (6730 μmol h−1 g−1) under natural sunlight. The optimized 0.75 wt% loaded SiO2@ZnIn2S4 shows twice hydrogen production rate as compared to pristine ZnIn2S4. The highest H2 evolution was achieved for ZS-4 (0.75 wt% loaded SiO2@ZnIn2S4) and is due to the high crystallinity, flower like morphology and band structure of SiO2@ZnIn2S4. More specifically, in contrast to earlier studies, the H2 evolution obtained for sample ZS-4 is much greater. The sample ZS-5 shows substantially less evolution of H2 due to the increase in band gap relative to sample ZS-4. It is mainly due to the fact that the relatively high weight ratio of SiO2 in SiO2@ZnIn2S4 (ZS-5) can increase shielding in the light absorption and opacity, which ultimately reduce penetration of light through the depth of the reaction solution. The efficient charge separation and possible hydrogen production mechanism in SiO2@ZnIn2S4 nano-heterostructure explained by considering the SiO2@ZnIn2S4 band gap energy diagram as shown in Scheme 2. Initially, the nano-heterostructure SiO2@ZnIn2S4 is irradiated under sunlight during photocatalysis. The photo-excitation of the charge carriers occurs in ZnIn2S4, which induces h+ in the valence band (VB) and e− in the ZnIn2S4 conduction band (CB). The photo-induced holes in the VB oxidizes S22−/SO32− to oxidation products (SO42−/S2O32−).48 Meanwhile, photo-induced electrons in the CB will move to the deposited SiO2 nanoparticles in order to reduce H+ to H2.
|
H2S + OH− ↔ HS− + H2O
| (7) |
|
H2S + OH− ↔ S2− + H2O
| (8) |
|
Photo catalyst → eCB− + hVB+
| (9) |
|
2HS− + 2hVB+ → S22− + 2H+
| (11) |
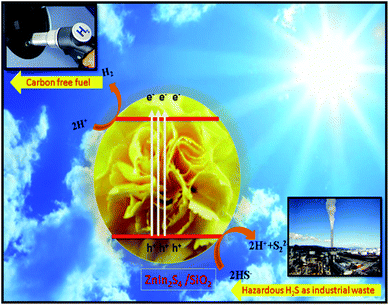 |
| Scheme 2 Schematic representation of the photocatalytic mechanism of SiO2@ZnIn2S4 heterostructure. | |
The existence of SiO2 nanoparticles in SiO2@ZnIn2S4 nano-heterostructure will thus prevent direct charge recombination and promote the separation of electrons and holes. Additionally, provide more active sites for surface reactions, resulting in the enhanced photocatalytic H2 production using SiO2@ZnIn2S4. The enhancement of hydrogen evolution rate is also supported by photoluminance (PL) study. PL Study shows that there is a decrease in PL intensity in case of ZS-4 Sample which indicates many vacancy defects in the composites which ultimately inhibits charge carrier recombination. In nutshell, the nanoheterostructure, flower like morphology is responsible for good hydrogen evolution in case of ZS-4 Sample.
4 Conclusions
In nutshell, SiO2@ZnIn2S4 nanoheterostructure with marigold flower like structure was synthesized successfully. The photocatalytic efficiency of the SiO2@ZnIn2S4 nanoheterostructure for the evolution of hydrogen under natural sunlight was investigated. The highest H2 evolution i.e. 796 μmol h−1 g−1 for water splitting & 6730 μmol h−1 g−1 for H2S Splitting using 0.75 wt percent SiO2 loading on ZnIn2S4 was achieved which is almost twice of pristine ZnIn2S4. It is due to high crystallinity, flower-like morphology and band structure of SiO2@ZnIn2S4. This study highlights the nanoheterostructure with marigold flower like morphology which shows efficient charge separations due to intimate contact of the SiO2 with nanopetals of flowers. Such kind of other nanoheterostructures may have potential as a photocatalyst for higher hydrogen evolution.
Conflicts of interest
There is no any conflict of intrest.
Acknowledgements
ARG like to thanks Dr R. J. Barnabas (Principal Ahmednagar College Ahmednagar) for useful suggestions and discussion and JRD Tata, SPPU for financial support. The authors would like to thank Nanocrystalline materials group. BBK would like to thanks the Ministry of Electronics and Information Technology (MeitY), Government of India and C-MET Pune for providing research facilities.
References
- H. She, Y. Sun, S. Li, J. Huang, L. Wang, G. Zhu and Q. Wang, Appl. Catal., B, 2019, 245, 439–447 CrossRef CAS
. - Y. A. Sethi, R. P. Panmand, S. R. Kadam, A. K. Kulkarni, S. K. Apte, S. D. Naik, N. Munirathnam, M. V. Kulkarni and B. B. Kale, Nanostructured CdS sensitized CdWO4 nanorods for hydrogen generation from hydrogen sulfide and dye degradation under sunlight, J. Chem. Inf. Model., 2017, 487, 504–512 CAS
. - P. Kumar, R. Boukherroub and K. Shankar, Sunlight-driven water-splitting using twodimensional carbon based semiconductors, J. Mater. Chem. A, 2018, 6, 12876–12931 RSC
. - S. R. Kadam, S. W. Gosavi, B. B. Kale, N. Suzuki, C. Terashima and A. Fujishima, Sci. Rep., 2019, 9, 1–10 CAS
. - A. K. Kulkarni, R. P. Panmand, Y. A. Sethi, S. R. Kadam, S. P. Tekale, G.-H. Baeg, A. V. Ghule and B. B. Kale, In situ preparation of N doped orthorhombic Nb2O5 nanoplates/rGO composites for photocatalytic hydrogen generation under sunlight, Int. J. Hydrogen Energy, 2018, 43, 19873 CrossRef CAS
. - R. Singh and S. Dutta, A review on H2 production through photocatalytic reactionsusing TiO2/TiO2- assisted catalysts, Fuel, 2018, 220, 607–620 CrossRef CAS
. - K. Fan, Z. Jin, G. Wang, H. Yang, D. Liu, H. Hu, G. Lu and Y. Bi, Catal. Sci. Technol., 2018, 8, 2352–2363 RSC
. - F. Opoku, K. K. Govender, C. G. C. E. van Sittert and P. P. Govender, New J. Chem., 2017, 41, 11701–11713 RSC
. - A. A. Dubale, A. G. Tamirat, H.-M. Chen, T. A. Berhe, C.-J. Pan, W.-N. Su and B.-J. Hwang, J. Mater. Chem. A, 2016, 4, 2205–2216 RSC
. - T. Su, Z. D. Hood, M. Naguib, L. Bai, Si Luo, C. M. Rouleau, I. N. Ivanov, H. Ji, Z. Qin and Z. Wu, Nanoscale, 2019, 11, 8138–8149 RSC
. - X. L. Ge, C. X. Li, Z. Q. Li and L. W. Yin, Tannic acid tuned metal-organic framework as a high-efficiency chemical anchor of polysulfide for lithium-sulfur batteries, Electrochim. Acta, 2018, 281, 700–709 CrossRef CAS
. - E. A. Dolgopolova, A. M. Rice, C. R. Martin and N. B. Shustova, Photochemistry and photophysics of MOFs: steps towards MOF-based sensing enhancements, Chem. Soc. Rev., 2018, 47, 4710–4728 RSC
. - F. C. Leng, H. Liu, M. L. Ding, Q. P. Lin and H. L. Jiang, Boosting photocatalytic hydrogen production of porphyrinic MOFs: the metal location in metalloporphyrin matters, ACS Catal., 2018, 8, 4583–4590 CrossRef CAS
. - S. Wang, B. Y. Guan and X. W. (David) Lou, Construction of ZnIn2S4-In2O3 hierarchical tubular heterostructures for efficient CO2 photoreduction, J. Am. Chem. Soc., 2018, 140, 5037–5040 CrossRef CAS PubMed
. - B. Valizadeh, T. N. Nguyen, B. Smit and K. C. Stylianou, Porous metal-organic framework@polymer beads for iodine capture and recovery using a gas-sparged column, Adv. Funct. Mater., 2018, 28, 1801596–1801601 CrossRef
. - W. Pudkon, S. Kaowphong, S. Pattisson, P. J. Miedziak, H. Bahruji, T. E. Davies, D. J. Morgan and G. J. Hutchings, Catal. Sci. Technol., 2019, 9, 5698–5711 RSC
. - S. B. Kale, R. S. Kalubarme, M. A. Mahadadalkar, H. S. Jadhav, A. P. Bhirud, J. D. Ambekar, C.-J. Park and B. B. Kale, Phys. Chem. Chem. Phys., 2015, 17, 31850–31861 RSC
. - Y. Xia, Q. Li, K. Lv, D. Tang and M. Li, Appl. Catal., B, 2017, 206, 344–352 CrossRef CAS
. - D. Zeng, Z. Lu, X. Gao, B. Wu and W.-J. Ong, Catal. Sci. Technol., 2019, 9, 4010–4016 RSC
. - Y. Gao, K. Qian, B. Xu, Fu Ding, V. Dragutan, I. Dragutan, Y. Sun and Z. Xu, RSC Adv., 2020, 10, 32652–32661 RSC
. - A. R. Gunjal, A. K. Kulkarni, U. V. Kawade, Y. A. Sethi, R. S. Sonawane, J. Ook-Baeg, A. V. Nagawade and B. B. Kale, Nanoscale Adv., 2020, 2, 2577–2586 RSC
. - Yu Gao, B. Xu, C. Mohamed, Yu He, Q. Zhang, F. Vidal, X. Wang, Fu Ding, Y. Sun, D. Ma, Y. Bi and Z. Xu, Appl. Catal., B, 2020, 279, 119403 CrossRef CAS
. - W. Chen, R.-Q. Yan, J.-Q. Zhu, G.-Bo Huang and Z. Chen, Appl. Surf. Sci., 2020, 504, 144406 CrossRef CAS
. - K. Huang, C. Li and X. Meng, J. Colloid Interface Sci., 2020, 580, 669–680 CrossRef CAS PubMed
. - X. Zhao, W. Ju, J. Zhang, B. Liu, J. Zhang and X. Yi, New J. Chem., 2019, 43, 6234–6241 RSC
. - F. Yuan, S. Pascale, J. Lakey, S. Riahi, A. T. McDonald, M. Shrestha, D. J. Tobias, M. Shiraiwa and V. H. Grassian, Chem. Sci., 2019, 10, 2906–2914 RSC
. - J. Sun, H. Bi, S. Su, H. Jia, X. Xie and L. Sun, J. Membr. Sci., 2018, 553, 131–138 CrossRef CAS
. - H. Xu, J. Yan, Y. Xu, Y. Song, H. Li, J. Xia, C. Huan and H. Wan, Appl. Catal., B, 2017, 206, 344–352 CrossRef
. - H. Qiang, X. Niu, C. Nie, S. Hao, W. Zou, J. Ge, D. Chen and W. Yao, Phys. Chem. Chem. Phys., 2016, 18, 31410–31418 RSC
. - L. Wang, H. Zhou, H. Zhang, H. Zhang, Y. Song, H. Zhang and X. Qian Inorg, Chem, 2020, 59(4), 2278–2287 CAS
. - W. Zhang, Y. Zhang, K. Yang, Y. Yang, J. Jia and L. Guo, Nanomaterials, 2019, 9, 1671 CrossRef CAS PubMed
. - AshutoshKumar, M. Khan, X. Zen and I. M. C. Lo, Chem. Eng. J., 2018, 353, 645–656 CrossRef
. - F. Wang, M. Li, L. Yu, F. Sun, Z. Wang, L. Zhang, H. Zeng and X. Xu, Sci. Rep., 2017, 7, 6960 CrossRef PubMed
. - C. Liu, Y. Dong, J. Yang, T. Yao, Y. Wang and Z. Jiang, ACS Appl. Mater. Interfaces, 2013, 5(9), 3824–3832 CrossRef CAS PubMed
. - R. M. Mohameda and F. A. Harraz, Mater. Res. Bull., 2020, 131, 110965 CrossRef
. - X. Meng, Y. Zhuang, H. Tang and C. Lu, J. Alloys Compd., 2018, 761, 15–23 CrossRef CAS
. - X. Lin, X. Guo, D. Liu, Q. Wang, H. Zhai and L. Chang, Mater. Res. Bull., 2015, 63, 72–79 CrossRef CAS
. - K. Huang, C. Li and X. Meng, J. Colloid Interface Sci., 2020, 580, 669–680 CrossRef CAS PubMed
. - Y. Chen, S. Hu, W. Liu, X. Chen, L. Wu, X. Wang, P. Liu and Z. Li, Dalton Trans., 2011, 40, 2607–2613 RSC
. - A. Khan, M. Danish, U. Alam, S. Zafar and M. Muneer, Facile Synthesis of a Z-Scheme ZnIn2S4/MoO3 Heterojunction with Enhanced Photocatalytic Activity under Visible Light Irradiation, ACS Omega, 2020, 5(14), 8188–8199 CrossRef CAS PubMed
. - S. Lai, Z. Yang, J. Li, B. Shao, J. Yang, Y. Wang, J. Qiu and Z. Song, J. Mater. Chem. C, 2015, 3, 7699–7708 RSC
. - J. Wang, Y. Chen, W. Zhou, G. Tian, Y. Xiao, H. Fu and H. Fu, J. Mater. Chem. A, 2017, 5, 8451–8460 RSC
. - W. Pudkon, H. Bahruji, P. J. Miedziak, T. E. Davies, D. J. Morgan, S. Pattisson, S. Kaowphong and G. J. Hutchings, Catal. Sci. Technol., 2020, 10, 2838–2854 RSC
. - M. Li, Y. Tao, J. Tang, Y. Wang, X. Zhang, Y. Tao and X. Wang, Synergetic Organocatalysis for Eliminating Epimerization in RingOpening Polymerizations Enables Synthesis of Stereoregular Isotactic Polyester, J. Am. Chem. Soc., 2019, 141, 281–289 CrossRef CAS PubMed
. - Y. Chen, G. Tian, W. Zhou, Y. Xiao, J. Wang, X. Zhang and H. Fu, Nanoscale, 2017, 9, 5912–5921 RSC
. - M. A. Mahadadalkar, S. B. Kale, R. S. Kalubarme, A. P. Bhirud, J. D. Ambekar, S. W. Gosavi, M. V. Kulkarni, C.-J. Park and B. B. Kale, RSC Adv., 2016, 6, 34724–34736 RSC
. - Y. A. Sethia, A. K. Kulkarnib, A. A. Ambalkara, S. K. Khorea, A. R. Gunjala, S. W. Gosavi and B. B. Kale, Nanoscale Adv., 2021, 3, 508–516 RSC
. - U. V. Kawade, R. P. Panmand, Y. A. Sethi, M. V. Kulkarni, S. K. Apte, S. D. Naik and B. B. Kale, RSC Adv., 2014, 4(90), 49295–49302 RSC
.
Footnote |
† Electronic supplementary information (ESI) available. See DOI: 10.1039/d1ra01140e |
|
This journal is © The Royal Society of Chemistry 2021 |